DOI:
10.1039/D1SC06100C
(Edge Article)
Chem. Sci., 2022,
13, 3169-3175
NHC and visible light-mediated photoredox co-catalyzed 1,4-sulfonylacylation of 1,3-enynes for tetrasubstituted allenyl ketones†
Received
4th November 2021
, Accepted 6th February 2022
First published on 10th February 2022
Abstract
The modulation of selectivity of highly reactive carbon radical cross-coupling for the construction of C–C bonds represents a challenging task in organic chemistry. N-Heterocyclic carbene (NHC) catalyzed radical transformations have opened a new avenue for acyl radical cross-coupling chemistry. With this method, highly selective cross-coupling of an acyl radical with an alkyl radical for efficient construction of C–C bonds was successfully realized. However, the cross-coupling reaction of acyl radicals with vinyl radicals has been much less investigated. We herein describe NHC and visible light-mediated photoredox co-catalyzed radical 1,4-sulfonylacylation of 1,3-enynes, providing structurally diversified valuable tetrasubstituted allenyl ketones. Mechanistic studies indicated that ketyl radicals are formed from aroyl fluorides via the oxidative quenching of the photocatalyst excited state, allenyl radicals are generated from chemo-specific sulfonyl radical addition to the 1,3-enynes, and finally, the key allenyl and ketyl radical cross-coupling provides tetrasubstituted allenyl ketones.
Introduction
Radical cross-coupling between two carbon radicals has emerged as a powerful platform for constructing C–C bonds and has received increasing attention.1 Since the radical–radical coupling reactions proceed in a diffusion-controlled manner, selectivity modulation is the critical challenge.1b Through radical addition to the unsaturated bond to form a C–C bond, acyl radicals have been utilized in preparing diverse carbonyl compounds.2 However, radical-coupling reactions between acyl and other carbon-centered radicals are rare. N-Heterocyclic carbene (NHC) catalysis has emerged as an attractive strategy in synthetic chemistry to access value-added organics via the formation of the key Breslow intermediate (BI).3 Recently, the single-electron-transfer (SET) of BI was found to provide ketyl-type radical species, which opens a new avenue for acyl radical chemistry.4–13 As a result, NHC catalyzed radical-coupling has attracted great attention after the pioneering work of Ohmiya in 2019.7a Alkyl radical sources such as redox-active esters,7 Katritzky pyridinium salts,8 Hantzsch ester,9 benzylic C–H bonds,6e alkylborates,10g olefins6c,10 and cyclopropanes6f could be used to perform cross-coupling reactions with ketyl radicals to form C–C bonds under thermal or photoredox conditions (Scheme 1a). Despite those innovative approaches, NHC catalyzed radical transformations have mainly been focused on coupling with alkyl radical species, while cross-coupling between highly active vinyl radicals and ketyl radicals though being extremely attractive is still largely underdeveloped.11
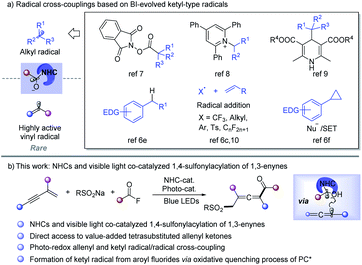 |
| Scheme 1 Radical C–C bond formation based on BI-derived ketyl-type radicals. | |
On the other hand, radical 1,4-difunctionalization14,15 of 1,3-enynes provides an elegant and versatile strategy for tetrasubstituted allenes from easily available feedstocks. In this regard, in situ generated allene radicals undergo cyanation,15a–d arylation,15e–i halogenation,15j alkynylation,15k trifluoromethylation,15l or intramolecular cyclization15m to afford functionalized allenes. Radical acylation of 1,3-enynes may provide straightforward access to value-added allenyl ketone units,11 which are a crucial core in important nature products16 and synthetic intermediates.17 However, radical acylation of 1,3-enynes has been much less developed and is limited to carboacylation,11 mainly due to the lack of an efficient acyl transfer approach. Recently, Studer et al. developed acylative difunctionalization of olefins6c/cyclopropanes6f and formal alkenyl6d/benzylic6e C–H acylation by employing aroyl fluorides as ketyl-type radical precursors via photo-induced SET. Inspired by those elegant approaches, we speculated that an NHC and visible light co-catalyzed system6c–f,9,12,13 might enable the generation of allenyl radicals and NHC stabilized ketyl radicals under extremely mild conditions, which may provide an opportunity for radical acylation of 1,3-enynes. Sulfone-containing compounds found widespread applications in organic synthesis, medicinal chemistry, and materials science.18 As part of our continued interest in radical chemistry19a–g and NHC catalysis,19h we now describe the development of NHC and photocatalysis co-catalyzed three-component radical 1,4-sulfonylacylation of 1,3-enynes, providing direct access to structurally diversified tetrasubstituted allenyl ketones (Scheme 1b).
Results and discussion
We commenced our investigation by employing a 1,3-enyne (1a), benzoyl fluoride (2a), and TolSO2Na (3a) as the prototype substrates, and PC-1 (1.5 mol%) and NHC-1 (15 mol%) as catalysts. Pleasingly, in dichloromethane (DCM), under irradiation with a blue LED at room temperature for 4 h, the expected allenyl ketone 4 was obtained in 10% yield in combination with competitive byproduct 5 (10%).20 Ir-based photocatalysts PC-2 and PC-3 improved the reactivity and selectivity (Table 1, entries 2 and 3), while PC-4 and PC-5 were inefficient for this reaction (Table 1, entries 4 and 5). The employment of other solvents such as CH3CN, PhCF3, or THF provided 4 in relatively lower yields (entries 6–8). The structure of NHCs was crucial for chemo-selectivity control (entries 11–15). NHC-2 and NHC-3 were unsatisfactory (entries 11 and 12). The N-2,6-diethyl phenyl substituted catalyst NHC-4 afforded 4 with a slightly diminished yield compared to NHC-1 (entry 13). For NHC-5 or NHC-6, decreased yield was observed (entries 14 and 15). Other bases, such as CsOAc and K2CO3, were applicable, with slightly lower yields (entries 9 and 10). To our delight, the yield could be further improved by running the reaction at lower concentration (Table 1, entries 16 and 17), affording 4 in 80% isolated yield with negligible yield of 5 in 4 mL DCM (entry 17). The desired 1,4-sulfonylacylation product 3aa was isolated in 75% yield when the reaction was run at 0.2 mmol scales (entries 18 and 19) by employing chiral or racemic NHC-1 as the catalyst, and these conditions were thus defined as the standard reaction conditions for subsequent investigations. Finally, benzoic anhydride was employed as an acyl radical precursor, and 3aa was obtained in 26% yield (entry 20).
Table 1 Conditions optimizationa,b

|
Entry |
NHCs (15 mol%) |
PCs (1.5 mol%) |
Solvent (mL) |
Yields (%) |
4
|
5
|
Unless otherwise noted, all the reactions were carried out with 1a (0.1 mmol), 2a (0.2 mmol), 3a (0.2 mmol), NHCs (0.015 mmol), Cs2CO3 (0.2 mmol), and PCs (0.0015 mmol) in anhydrous solvent, and irradiation with a blue LED (453.5 nm, 10 W) at room temperature for 4 h.
Isolated yields.
CsOAc (0.2 mmol) was used as a base.
K2CO3 (0.2 mmol) was used as a base.
0.2 mmol scale reaction was conducted.
Benzoic anhydride (0.4 mmol) was used instead of 2a.
|
1 |
NHC-1
|
PC-1
|
DCM (2) |
10 |
10 |
2 |
NHC-1
|
PC-2
|
DCM (2) |
45 |
14 |
3 |
NHC-1
|
PC-3
|
DCM (2) |
65 |
12 |
4 |
NHC-1
|
PC-4
|
DCM (2) |
16 |
15 |
5 |
NHC-1
|
PC-5
|
DCM (2) |
<5 |
<5 |
6 |
NHC-1
|
PC-3
|
CH3CN (2) |
22 |
17 |
7 |
NHC-1
|
PC-3
|
CF3Ph (2) |
56 |
8 |
8 |
NHC-1
|
PC-3
|
THF (2) |
36 |
12 |
9c |
NHC-1
|
PC-3
|
DCM (2) |
37 |
25 |
10d |
NHC-1
|
PC-3
|
DCM (2) |
51 |
20 |
11 |
NHC-2
|
PC-3
|
DCM (2) |
15 |
14 |
12 |
NHC-3
|
PC-3
|
DCM (2) |
<5 |
20 |
13 |
NHC-4
|
PC-3
|
DCM (2) |
60 |
17 |
14 |
NHC-5
|
PC-3
|
DCM (2) |
40 |
12 |
15 |
NHC-6
|
PC-3
|
DCM (2) |
53 |
6 |
16 |
NHC-1
|
PC-3
|
DCM (1) |
29 |
9 |
17 |
NHC-1
|
PC-3
|
DCM (4) |
80 |
<5 |
18e |
NHC-1
|
PC-3
|
DCM (8) |
75 |
<5 |
19
|
rac-NHC-1 |
PC-3
|
DCM (8)
|
75 |
<5 |
20f |
rac-NHC-1 |
PC-3
|
DCM (8) |
26 |
8 |
|
With the optimized reaction conditions, the scope of 1,3-enynes was explored. As shown in Scheme 2a, 1,3-enynes bearing various electron-donating or -withdrawing substituents at the ortho (6–9), meta (10 and 11), or para (12–16) positions of the 2-phenyl rings, such as alkyl, methoxyl, halogen, methoxycarbonyl, trifluoromethyl, and trifluoromethoxy groups, were fully tolerated affording the corresponding products 6–16 smoothly. 1,3-Enynes bearing naphthalene, fluorene, and pyridine were also compatible with the transformation, and corresponding products 17–19 were formed in 50–93% yields. The functional groups linked to the alkyne triple bond could also be diversified. As shown in Scheme 2a, 1,3-enynes with n-hexyl (4–21), cyclohexyl (25), cyclopropyl (27), and chloroalkyl (26) groups were tolerated for this transformation. Moreover, good coupling efficiencies were maintained for 2,4-diaryl substituted 1,3-enynes (23 and 24). It should be noted that the vulnerable Bpin (24), insular alkyne (20), and olefin (21) units have been preserved after transformation. Furthermore, internal 1,3-enynes and 2-alkyl substituted 1,3-enynes were applicable, affording 22 and 28 in 66% (3
:
1 dr) and 71% yields, respectively. The structure of 28 was confirmed by X-ray single-crystal diffraction (CCDC 2090996).21 Next, we turned our attention to the scope of the sulfonyl radical source; various β-sulfonated allenyl ketones 29–40 could be obtained in good yields (Scheme 2b). Sodium arylsulfinates with methyl substituents in ortho- and meta-positions were well compatible under the reaction conditions, delivering 30 and 31 in 80 and 86% yields, respectively. The functional group tolerances and electronic effects were next investigated based on para-substituted sodium arylsulfinates. An array of electron-donating (t-Bu), -withdrawing (cyano, trifluoromethyl, and carbonyl), and halogen groups were tolerated under the standard conditions, affording 32–36 in 72–90% yields. Sodium arylsulfinates containing naphthalene (37), pyridine (38), and thiophene (39) proved to be viable substrates. Notably, methyl, ethyl and cyclopropyl substituted sodium sulfite could also deliver difunctionalization products 40–42 in 70–80% yield.
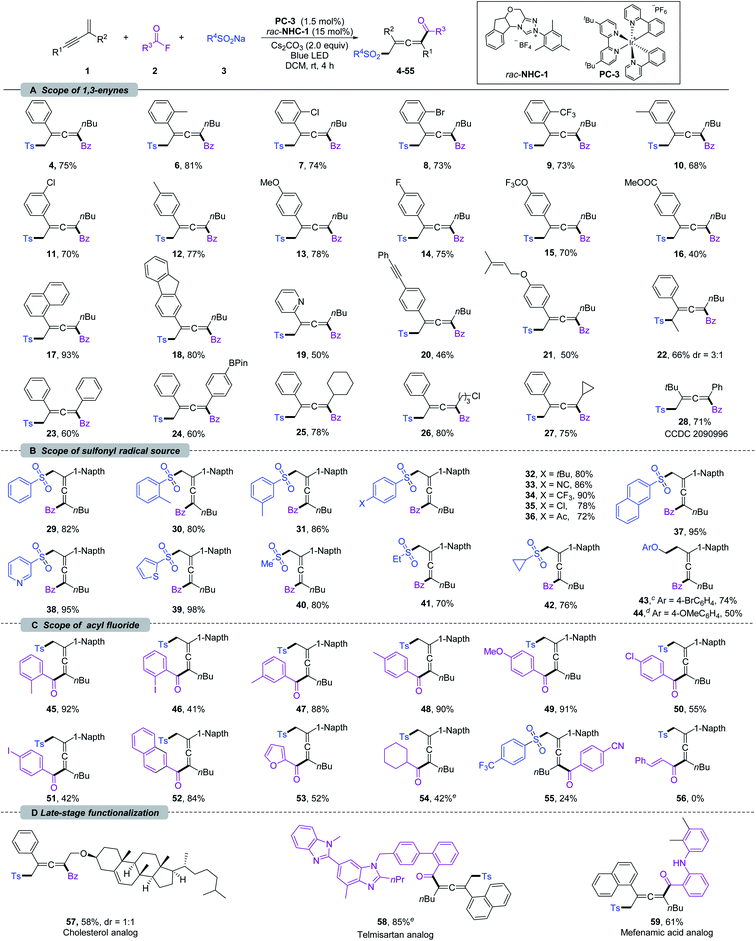 |
| Scheme 2 Substrate scope for 1,4-sulfonylacylation of 1,3-enynes.a,b a Reaction conditions: unless otherwise noted, all the reactions were carried out with 1 (0.2 mmol), 2 (0.4 mmol), 3 (0.4 mmol), rac-NHC-1 (0.03 mmol), PC-3 (0.003 mmol) and Cs2CO3 (0.4 mmol) in DCM (8 mL) at rt under N2, and irradiation with a blue LED (453.5 nm, 10 W) for 4 h. b Isolated yield. c 4-BrC6H4OCH2BF4K was used as a radical source. d 4-OMeC6H4OCH2BF4K was used as a radical source. e Reactions were carried out with in situ generated acyl fluoride; see the ESI† for detailed reaction conditions. | |
Very recently, the Du11a and Huang11b groups developed 1,4-alkylacylation of 1,3-enynes under thermal conditions by employing an electrophilic alkyl radical precursor. It should be noted that our NHC and PC co-catalyzed system could be extended to alkyl trifluoroborates. By employing Scheidt's aryloxymethyl trifluoroborates,10h the desired 1,4-alkylacylation products 43 and 44 were obtained in 74 and 50% yields, respectively. These exciting results encouraged us to evaluate the scope of acyl fluorides (Scheme 2c). This sulfonylacylation reaction was insensitive to the steric hindrance of benzoyl fluoride (45–53). The electron-donating aryl acyl fluorides showed excellent reactivities (45 and 48–50), while the presence of strong electron-deficient groups (55) led to low efficiency. Remarkably, the iodine group, which is sensitive in most metal-catalyzed coupling reactions, did not inhibit the reaction (46 and 51), providing an opportunity for further transformations. The aryl groups have been extended to naphthalene and heterocycles, providing 52 and 53 in acceptable yields. Importantly, an alkyl acyl fluoride could be used as well in this transformation, affording the corresponding allene 54 in 42% yield. Unfortunately, cinnamoyl fluoride (56) was not suitable for this conversion. Taking advantage of the mild reaction conditions as well as broad functional group tolerances, the 1,4-sulfonylacylation of enynes could be applied at a late-stage functionalization. As shown in Scheme 2d, the 1,3-enynes derived from cholesterol could participate in this reaction, delivering 57 in 58% (1
:
1 dr) yield. Furthermore, the fluorides derived from natural products such as telmisartan and mefenamic acid were successfully converted into 58 and 59 in 85% and 61% yields, respectively.
Considering the mild reaction conditions as well as tolerance with chiral NHC catalysts, we attempted the challenging chiral allene synthesis. Unfortunately, unsatisfactory enantioselectivity was observed for both chiral NHC-1 and NHC-6 (Scheme 3).
 |
| Scheme 3 Attempts at asymmetric 1,4-sulfonylacylation of 1,3-enynes. | |
Large-scale synthesis and derivatization reactions were performed to showcase the synthetic applications (Scheme 4). Scale-up synthesis of 17 has been achieved at a 2.0 mmol scale, and a comparable yield was obtained (Scheme 4a). When employing PhLi as a base, the tetrasubstituted allenyl ketone 4 could isomerize to diene product 60 in 78% yield. 4 could undergo reduction of the ketone unit with NaBH4. The allenyl ketone 4 could easily be transformed into conjugated viny selenyl ether 62 in 50% yield with excellent Z/E selectivity. When treated with concentrated H2SO4, Nazarov cyclization product 63 was isolated in 86% yield.
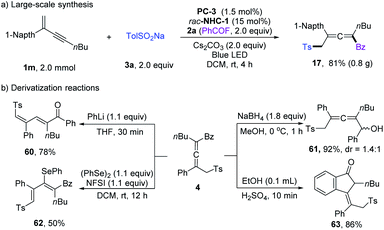 |
| Scheme 4 Large-scale synthesis and derivatization reactions. | |
A series of control experiments were performed to unravel the reaction mechanism. Light, NHCs, and photoredox catalysis were indispensable for this 1,4-sulfonylacylation reaction (Scheme 5a). When the radical scavenger 2,2,6,6-tetramethylpiperidine 1-oxyl (TEMPO) was added, the reaction was suppressed, and TEMPO-trapped product 64 was separated in 55% yield (Scheme 5b), thus suggesting the formation of ketyl radicals. Furthermore, a trace amount of 4,4′-dimethyl-1,1′-biphenyl (66) was isolated under standard conditions, indicating the involvement of a sulfonyl radical. The intermediacy of acyl azoliums has been confirmed by coupling of acyl azolium ion 65 with 1,3-enyne 1a and sodium benzenesulfinate 3a in the absence of NHCs (Scheme 5c). The radical chain process could be ruled out based on light/dark experiments (Fig. S4, see the ESI†). Then Stern–Volmer quenching studies were conducted to clarify the plausible photoredox mechanism (Scheme 6). 1,3-Enynes 1a and sodium benzenesulfinate 3a do not show a significant luminescence quenching effect on the excited state of Ir*(III). In contrast, the Ir*-complex was effectively quenched by acyl azolium ion 65, pointing to the oxidative quenching process.
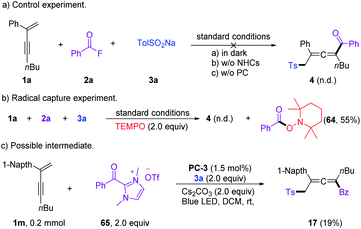 |
| Scheme 5 Mechanism investigation. | |
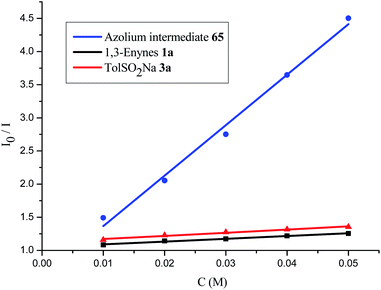 |
| Scheme 6 Stern–Volmer quenching studies. | |
Based on a series of experimental studies and previous reports, a plausible catalytic cycle for the 1,4-sulfonylacylation is proposed in Scheme 7. The acyl fluoride or in situ generated bisacyl carbonate intermediate6f could react with NHCs providing acylazolium intermediate I. Upon visible light irradiation, the excited state of [Ir(ppy)2(dtbbpy)]PF6 undergoes an oxidative quenching22 by I to yield the IrIV-complex and ketyl radical II. Single-electron transfer between the IrIV-complex and aryl sulfinate provides an aryl sulfonyl radical III while regenerating the ground-state photocatalyst (IrIII), closing the photoredox cycle. The sulfonyl radical then adds to the olefin unit of the 1,3-enyne 1 delivering the propargyl radical IV, which could undergo reversible resonance to generate trisubstituted allenyl radical V.15 Subsequently, chemo-specific radical/radical cross-coupling between the persistent ketyl radical II and transient allenyl radical V affords NHC-bound intermediate VI. The exclusive coupling selectivity might be regulated by the persistent radical effect1b as well as the steric exclusion of propargyl radical IV with ketyl radical II. VI disintegrates to give rise to the final product 4, while the NHC is regenerated for the next NHC cycle. Meanwhile, SO2 fragments of the sulfonyl radical produced aryl radicals, which undergo homocoupling affording biaryl 66. Radical–radical cross-coupling of V and IV affords the byproduct 5.1b,20 Meanwhile, direct homo-coupling of V or IV was not detected in our reaction system, which might be due to the persistent radical effect.1b
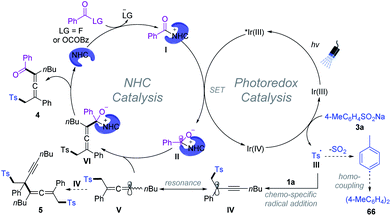 |
| Scheme 7 Proposed catalytic cycle. | |
Conclusions
In summary, we have realized an efficient 1,4-sulfonylacylation of 1,3-enynes by merging photocatalysis with NHCs. This transformation provided a facile and direct access to tetrasubstituted allenyl ketones under mild conditions with broad functional group tolerance and excellent chemo- and regioselectivity. Mechanistic studies indicated that the key step of the transformation is allenyl and ketyl radical cross-coupling, proving a new avenue for NHC catalyzed radical chemistry. The ketyl radical was formed from aroyl fluorides via the oxidative quenching of the photocatalyst excited state. Further extension of this cross-coupling system to other destabilized transient radicals is ongoing in our laboratory.
Data availability
Data for all compounds in this manuscript are available in the ESI,† which includes experimental details, characterization and copies of 1H and 13C NMR spectra. Crystallographic data for compound 28 has been deposited at the CCDC under CCDC 2090996.
Author contributions
L. W., R. M., and J. S. performed the experiments. G. Z. and Q. Z. conceived the concept, directed the project and wrote the paper.
Conflicts of interest
There are no conflicts to declare.
Acknowledgements
We acknowledge the NSFC (21831002, 22001157, and 22193012), Ten Thousand Talents Program, the Fundamental Research Funds for the Central Universities (2412021QD007), and the Natural Science Foundation of Shaanxi Province (2020JQ-404) for generous financial support.
Notes and references
-
(a) J. Xie, H. Jin and A. S. K. Hashmi, Chem. Soc. Rev., 2017, 46, 5193–5203 RSC;
(b) D. Leifert and A. Studer, Angew. Chem., Int. Ed., 2020, 59, 74–108 CrossRef CAS PubMed;
(c) A. Bhunia and A. Studer, Chem, 2021, 7, 1–41 CrossRef;
(d) J. D. Bell and J. A. Murphy, Chem. Soc. Rev., 2021, 50, 9540–9685 RSC;
(e) Y. Sohtome, K. Kanomata and M. Sodeoka, Bull. Chem. Soc. Jpn., 2021, 94, 1066–1079 CrossRef CAS;
(f) Y. Yuan, J. Yanga and A. Lei, Chem. Soc. Rev., 2021, 50, 10058–10086 RSC.
-
(a) C. Chatgilialoglu, D. Crich, M. Komatsu and I. Ryu, Chem. Rev., 1999, 99, 1991–2069 CrossRef CAS PubMed;
(b) A. Banerjee, Z. Lei and M. Ngai, Synthesis, 2019, 303–333 CAS;
(c) Y. Liu, Y. Ouyang, H. Zheng, H. Liu and W. Wei, Chem. Commun., 2021, 57, 6111–6120 RSC.
-
(a) D. Enders, O. Niemeier and A. Henseler, Chem. Rev., 2008, 107, 5606–5655 CrossRef PubMed;
(b) X. Bugaut and F. Glorius, Chem. Soc. Rev., 2012, 41, 3511–3522 RSC;
(c) M. N. Hopkinson, C. Richter, M. Schedler and F. Glorius, Nature, 2014, 510, 485–496 CrossRef CAS PubMed;
(d) J. Mahatthananchai and J. W. Bode, Acc. Chem. Res., 2014, 47, 696–707 CrossRef CAS PubMed;
(e) R. S. Menon, A. T. Biju and V. Nair, Chem. Soc. Rev., 2015, 44, 5040–5052 RSC;
(f) D. M. Flanigan, F. Romanov-Michailidis, N. A. White and T. Rovis, Chem. Rev., 2015, 115, 9307–9387 CrossRef CAS PubMed;
(g) C. Zhang, J. F. Hooper and D. W. Lupton, ACS Catal., 2017, 7, 2583–2596 CrossRef CAS;
(h) X.-Y. Chen, Q. Liu, P. Chauhan and D. Enders, Angew. Chem., Int. Ed., 2018, 57, 3862–3873 CrossRef CAS PubMed;
(i) K. J. R. Murauski, A. A. Jaworski and K. A. Scheidt, Chem. Soc. Rev., 2018, 47, 1773–1782 RSC;
(j) S. Mondal, S. R. Yetra, S. Mukherjee and A. T. Biju, Acc. Chem. Res., 2019, 52, 425–436 CrossRef CAS PubMed;
(k) X. Chen, Z. Gao and S. Ye, Acc. Chem. Res., 2020, 53, 690–702 CrossRef CAS PubMed;
(l) P. Bellotti, M. Koy, M. N. Hopkinson and F. Glorius, Nat. Rev. Chem., 2021, 5, 711–725 CrossRef CAS.
-
(a) I. Nakanishi, S. Itoh, T. Suenobu and S. Fukuzumi, Chem. Commun., 1997, 1927–1928 RSC;
(b) J. K. Mahoney, D. Martin, C. E. Moore, A. L. Rheingold and G. Bertrand, J. Am. Chem. Soc., 2013, 135, 18766–18769 CrossRef CAS PubMed;
(c) V. Regnier, E. A. Romero, F. Molton, R. Jazzar, G. Bertrand and D. Martin, J. Am. Chem. Soc., 2019, 141, 1109–1117 CrossRef CAS PubMed.
-
(a) K. Zhao and D. Enders, Angew. Chem., Int. Ed., 2017, 56, 3754–3756 CrossRef CAS PubMed;
(b) R. Song and Y. R. Chi, Angew. Chem., Int. Ed., 2019, 58, 8628–8630 CrossRef CAS;
(c) T. Ishii, K. Nagao and H. Ohmiya, Chem. Sci., 2020, 11, 5630–5636 RSC;
(d) Q. Liu and X.-Y. Chen, Org. Chem. Front., 2020, 7, 2082–2087 RSC;
(e) H. Ohmiya, ACS Catal., 2020, 10, 6862–6869 CrossRef CASK.-Q. Chen, H. Sheng, Q. Liu, P.-L. Shao and X.-Y. Chen, Sci. China: Chem., 2021, 64, 7–16 CrossRef CAS;
(f) Q.-Z. Li, R. Zeng, B. Han and J.-L. Li, Chem.–Eur. J., 2021, 27, 3238–3250 CrossRef CAS PubMed;
(g) Y. Sumoda and H. Ohmiya, Chem. Soc. Rev., 2021, 50, 6320–6332 RSC.
-
(a) J. Guin, S. D. Sarkar, S. Grimme and A. Studer, Angew. Chem., Int. Ed., 2008, 47, 8727–8730 CrossRef CAS PubMed;
(b) J. Zhao, C. Mück-Lichtenfeld and A. Studer, Adv. Synth. Catal., 2013, 355, 1098–1106 CrossRef CAS;
(c) Q.-Y. Meng, N. Döben and A. Studer, Angew. Chem., Int. Ed., 2020, 59, 19956–19960 CrossRef CAS PubMed;
(d) K. Liu and A. Studer, J. Am. Chem. Soc., 2021, 143, 4903–4909 CrossRef CAS PubMed;
(e) Q.-Y. Meng, L. Lezius and A. Studer, Nat. Commun., 2021, 12, 2068 CrossRef CAS PubMed;
(f) Z. Zuo, C. G. Daniliuc and A. Studer, Angew. Chem., Int. Ed., 2021, 60, 25252–25257 CrossRef CAS PubMed.
-
(a) T. Ishii, Y. Kakeno, K. Nagao and H. Ohmiya, J. Am. Chem. Soc., 2019, 141, 3854–3858 CrossRef CAS PubMed;
(b) Y. Kakeno, M. Kusakabe, K. Nagao and H. Ohmiya, ACS Catal., 2020, 10, 8524–8529 CrossRef CAS.
- L. Kim, H. Im, H. Lee and S. Hong, Chem. Sci., 2020, 11, 3192–3197 RSC.
-
(a) A. V. Davies, K. P. Fitzpatrick, R. C. Betori and K. A. Scheidt, Angew. Chem., Int. Ed., 2020, 59, 9143–9148 CrossRef PubMed;
(b) A. A. Bayly, B. R. McDonald, M. Mrksich and K. A. Scheidt, Proc. Natl. Acad. Sci. U. S. A., 2020, 117, 13261–13266 CrossRef CAS PubMed;
(c) A. V. Bay, K. P. Fitzpatrick, G. A. González-Montiel, A. O. Farah, P. H. Cheong and K. A. Scheidt, Angew. Chem., Int. Ed., 2021, 60, 17925–17931 CrossRef CAS PubMed;
(d) S.-C. Ren, W.-X. Lv, X. Yang, J.-L. Yan, J. Xu, F.-X. Wang, L. Hao, H. Chai, Z. Jin and Y. R. Chi, ACS Catal., 2021, 11, 2925–2934 CrossRef CAS.
-
(a) T. Ishii, K. Ota, K. Nagao and H. Ohmiya, J. Am. Chem. Soc., 2019, 141, 14073–14077 CrossRef CAS PubMed;
(b) K. Ota, K. Nagao and H. Ohmiya, Org. Lett., 2020, 22, 3922–3925 CrossRef CAS PubMed;
(c) Y. Matsuki, N. Ohnishi, Y. Kakeno, S. Takemoto, T. Ishii, K. Nagao and H. Ohmiya, Nat. Commun., 2021, 12, 3848 CrossRef CAS PubMed;
(d) H.-B. Yang, Z.-H. Wang, J.-M. Li and C. Wu, Chem. Commun., 2020, 56, 3801–3804 RSC;
(e) J.-L. Li, Y.-Q. Liu, W.-L. Zou, R. Zeng, X. Zhang, Y. Liu, B. Han, Y. He, H.-J. Leng and Q.-Z. Li, Angew. Chem., Int. Ed., 2020, 59, 1863–1870 CrossRef CAS PubMed;
(f) B. Zhang, Q. Peng, D. Guo and J. Wang, Org. Lett., 2020, 22, 443–447 CrossRef CAS PubMed;
(g) Y. Sato, Y. Goto, K. Nakamura, Y. Miyamoto, Y. Sumida and H. Ohmiya, ACS Catal., 2021, 11, 12886–12892 CrossRef CAS;
(h) P. Wang, K. P. Fitzpatrick and K. A. Scheidt, Adv. Synth. Catal., 2022, 364, 518–524 CrossRef CAS.
-
(a) C. Lei, C. Lin, S. Zhang, X. Zhang, J. Zhang, L. Xing, Y. Guo, J. Feng, J. Gao and D. Du, ACS Catal., 2021, 11, 13363–13373 CrossRef (During our preparation of this manuscript, Feng, Du and co-workers reported 1,4-alkylacylation of 1,3-enynes under thermal conditions);
(b) Y. Cai, J. Chen and Y. Huang, Org. Lett, 2021, 23, 9251–9255 CrossRef CAS PubMed (During our submission, Chen, Huang and co-workers reported 1,4-alkylacylation of 1,3-enynes under thermal conditions);
(c) L. Wang, R. Ma, J. Sun, G. Zheng and Q. Zhang, ChemRxiv, 2021 DOI:10.33774/chemrxiv-2021-5c17 (preprint).
-
(a) A. Mavroskoufis, M. Jakob and M. N. Hopkinson, ChemPhotoChem, 2020, 4, 5147–5153 CrossRef CAS;
(b) J. Liu, X.-N. Xing, J.-H. Huang, L.-Q. Lu and W.-J. Xiao, Chem. Sci., 2020, 11, 10605–10613 RSC.
-
(a) D. A. DiRocco and T. Rovis, J. Am. Chem. Soc., 2012, 134, 8094–8097 CrossRef CAS PubMed;
(b) L. Dai, Z.-H. Xia, Y.-Y. Gao, Z.-H. Gao and S. Ye, Angew. Chem., Int. Ed., 2019, 58, 18124–18130 CrossRef CAS PubMed;
(c) A. Mavroskoufis, K. Rajes, P. Golz, A. Agrawal, V. Ruß, J. P. Götze and M. N. Hopkinson, Angew. Chem., Int. Ed., 2020, 59, 3190–3194 CrossRef CAS PubMed.
- L. Fu, S. Greßies, P. Chen and G. Liu, Chin. J. Chem., 2020, 38, 91–100 CrossRef CAS.
-
(a) F. Wang, D. Wang, Y. Zhou, L. Liang, R. Lu, P. Chen, Z. Lin and G. Liu, Angew. Chem., Int. Ed., 2018, 57, 7140–7145 CrossRef CAS PubMed;
(b) X. Zhu, W. Deng, M.-F. Chiou, C. Ye, W. Jian, Y. Zeng, Y. Jiao, L. Ge, Y. Li, X. Zhang and H. Bao, J. Am. Chem. Soc., 2019, 141, 548–559 CrossRef CAS PubMed;
(c) Y. Zeng, M.-F. Chiou, X. Zhu, J. Cao, D. Lv, W. Jian, Y. Li, X. Zhang and H. Bao, J. Am. Chem. Soc., 2020, 142, 18014–18021 CrossRef CAS PubMed;
(d) Y. Chen, J. Wang and Y. Lu, Chem. Sci., 2021, 12, 11316–11321 RSC;
(e) J. Terao, F. Bando and N. Kambe, Chem. Commun., 2009, 7336–7338 RSC;
(f) K.-F. Zhang, K.-J. Bian, C. Li, J. Sheng, Y. Li and X.-S. Wang, Angew. Chem., Int. Ed., 2019, 58, 5069–5074 CrossRef CAS PubMed;
(g) C. Ye, Y. Li, X. Zhu, S. Hu, D. Yuan and H. Bao, Chem. Sci., 2019, 10, 3632–3636 RSC;
(h) Y. Chen, K. Zhu, Q. Huang and Y. Lu, Chem. Sci., 2021, 12, 13564–13571 RSC;
(i) T. Xu, S. Wu, Q.-N. Zhang, Y. Wu, M. Hu and J.-H. Li, Org. Lett., 2021, 23, 8455–8459 CrossRef CAS PubMed;
(j) Y. Song, S. Song, X. Duan, X. Wu, F. Jiang, Y. Zhang, J. Fan, X. Huang, C. Fu and S. Ma, Chem. Commun., 2019, 55, 11774–11777 RSC;
(k) X.-Y. Dong, T.-Y. Zhan, S.-P. Jiang, X.-D. Liu, L. Ye, Z.-L. Li, Q.-S. Gu and X.-Y. Liu, Angew. Chem., Int. Ed., 2021, 60, 2160–2164 CrossRef CAS PubMed;
(l) H. Shen, H. Xiao, L. Zhu and C. Li, Synlett, 2020, 31, 41–44 CrossRef CAS;
(m) C. Alameda-Angulo, B. Quiclet-Sire and S. Z. Zard, Tetrahedron Lett., 2006, 47, 913–916 CrossRef CAS;
(n) H.-M. Huang, P. Bellotti, C. Daniliuc and F. Glorius, Angew. Chem., Int. Ed., 2021, 60, 2464–2471 CrossRef CAS PubMed.
-
(a) A. Hoffmann-Roder and N. Krause, Angew. Chem., Int. Ed., 2004, 43, 1196–1216 CrossRef PubMed;
(b) L. U. Dzhemileva, V. A. D'Yakonov, A. A. Makarov, E. K. Makarova, E. N. Andreev and U. M. Dzhemilev, J. Nat. Prod., 2020, 83, 2399–2409 CrossRef CAS PubMed.
-
(a) A. S. Dudnik and V. Gevorgyan, Angew. Chem., Int. Ed., 2007, 46, 5195–5197 CrossRef CAS PubMed;
(b) C. Xue, X. Huang, S. Wu, J. Zhou, J. Dai, C. Fu and S. Ma, Chem. Commun., 2015, 51, 17112–17115 RSC;
(c) M. Miao, Y. Luo, H. Xu, Z. Chen, J. Xu and H. Ren, Org. Lett., 2016, 18, 4292–4295 CrossRef CAS PubMed;
(d) M. Miao, H. Xu, Y. Luo, M. Jin, Z. Chen, J. Xu and H. Ren, Org. Chem. Front., 2017, 4, 1824–1828 RSC;
(e) J. Teske and B. Plietker, Org. Lett., 2018, 20, 2257–2260 CrossRef CAS PubMed.
-
(a)
G. H. Whitham, Organosulfur Chemistry, Oxford University Press, Oxford, 1995 Search PubMed;
(b) M. Feng, B. Tang, S. H. Liang and X. Jiang, Curr. Top. Med. Chem., 2016, 16, 1200 CrossRef CAS PubMed;
(c)
X. Jiang, Sulfur Chemistry, Springer, Berlin, 2019 CrossRef;
(d) X. Chu, D. Ge, Y. Cui, Z. Shen and C. Li, Chem. Rev., 2021, 121, 12548–12680 CrossRef CAS PubMed.
-
(a) H. Zhang, W. Pu, T. Xiong, Y. Li, X. Zhou, K. Sun, Q. Liu and Q. Zhang, Angew. Chem., Int. Ed., 2013, 52, 2529–2533 CrossRef CAS PubMed;
(b) H. Zhang, Y. Song, J. Zhao, J. Zhang and Q. Zhang, Angew. Chem., Int. Ed., 2014, 53, 11079–11083 CrossRef CAS PubMed;
(c) G. Zhang, T. Xiong, Z. Wang, G. Xu, X. Wang and Q. Zhang, Angew. Chem., Int. Ed., 2015, 54, 12649–12653 CrossRef CAS PubMed;
(d) G. Zheng, Y. Li, J. Han, T. Xiong and Q. Zhang, Nat. Commun., 2015, 6, 7011 CrossRef CAS PubMed;
(e) J. Sun, G. Zheng, T. Xiong, Q. Zhang, J. Zhao, Y. Li and Q. Zhang, ACS Catal., 2016, 6, 3674–3678 CrossRef CAS;
(f) S. Yang, L. Wang, H. Zhang, C. Liu, L. Zhang, X. Wang, G. Zhang, Y. Li and Q. Zhang, ACS Catal., 2019, 9, 716–721 CrossRef CAS;
(g) T. Qin, G. Lv, Q. Meng, G. Zhang, T. Xiong and Q. Zhang, Angew. Chem., Int. Ed., 2021, 60, 25949–25957 CrossRef CAS PubMed;
(h) Y. Wu, M. Li, J. Sun, G. Zheng and Q. Zhang, Angew. Chem., Int. Ed., 2022, 61 DOI:10.1002/anie.202117340.
-
(a) F. Yang, G. Zhao, Y. Ding, Z. Zhao and Y. Zheng, Tetrahedron Lett, 2002, 43, 1289–1293 CrossRef CAS;
(b) G. V. Karunakar and M. Periasamy, Tetrahedron Lett., 2006, 47, 3549–3552 CrossRef CAS.
- CCDC 2090996 (28) contains the supplementary crystallographic data for this paper.†.
-
(a) J. Xuan and W. Xiao, Angew. Chem., Int. Ed., 2012, 51, 6828–6838 CrossRef CAS PubMed;
(b) C. K. Prier, D. A. Rankic and D. W. C. MacMillan, Chem. Rev., 2013, 113, 5322–5363 CrossRef CAS PubMed;
(c) J. D. Slinker, A. A. Gorodetsky, M. S. Lowry, J. Wang, S. Parker, R. Rohl, S. Bernhard and G. G. Malliaras, J. Am. Chem. Soc., 2004, 126, 2763–2767 CrossRef CAS PubMed.
Footnote |
† Electronic supplementary information (ESI) available. CCDC 2090996. For ESI and crystallographic data in CIF or other electronic format see DOI: 10.1039/d1sc06100c |
|
This journal is © The Royal Society of Chemistry 2022 |