DOI:
10.1039/D1SC05070B
(Edge Article)
Chem. Sci., 2022,
13, 421-429
Modular synthesis of unsymmetrical [1]benzothieno[3,2-b][1]benzothiophene molecular semiconductors for organic transistors†
Received
13th September 2021
, Accepted 27th November 2021
First published on 29th November 2021
Abstract
A modular approach to underexplored, unsymmetrical [1]benzothieno[3,2-b][1]benzothiophene (BTBT) scaffolds delivers a library of BTBT materials from readily available coupling partners by combining a transition-metal free Pummerer CH–CH-type cross-coupling and a Newman–Kwart reaction. This effective approach to unsymmetrical BTBT materials has allowed their properties to be studied. In particular, tuning the functional groups on the BTBT scaffold allows the solid-state assembly and molecular orbital energy levels to be modulated. Investigation of the charge transport properties of BTBT-containing small-molecule:polymer blends revealed the importance of molecular ordering during phase segregation and matching the highest occupied molecular orbital energy level with that of the semiconducting polymer binder, polyindacenodithiophene-benzothiadiazole (PIDTBT). The hole mobilities extracted from transistors fabricated using blends of PIDTBT with phenyl or methoxy functionalized unsymmetrical BTBTs were double those measured for devices fabricated using pristine PIDTBT. This study underscores the value of the synthetic methodology in providing a platform from which to study structure–property relationships in an underrepresented family of unsymmetrical BTBT molecular semiconductors.
Introduction
Organic semiconductors (OSCs) are essential active components in a wide range of next-generation electronic and energy devices including field-effect transistors,1 solar energy convertors,2 and chemical/bio sensors.3 The charge transport in OSCs is strongly governed by their molecular organization in the solid-state (i.e. thin-film structure) and their electronic properties. In general, these properties can be tuned through molecular design of the OSC architecture (e.g. side-chain engineering, conjugated backbone structure),4 conjugation break spacers,5 and/or the type of thin-film deposition technique.6 Among the different OSCs, conjugated [1]benzothieno[3,2-b][1]benzothiophene (BTBT) scaffolds have been extensively used as a platform for the construction of high-performing small-molecule semiconductors.7 Their tendency to pack into highly ordered structures during film formation often results in favorable charge (hole) transport in transistors.8 Most studies have investigated the packing behavior of symmetrical 2,7-functionalized BTBTs and the relationship between transistor properties and variations in length (odd-even effect),9 functional end-groups on the aliphatic side-chains, and bulky substituent groups (Scheme 1A).10 A prototypical example is the widely used 2,7-dioctyl-BTBT (C8-BTBT) which has reported some of the highest hole mobilities (μHole); above 10 cm2 V−1 s−1 from solution-processed OFETs as single-crystals, binary blends with polymer insulators (e.g. polystyrene),11 and ternary blends with a polymer semiconductor and various p-dopants.12
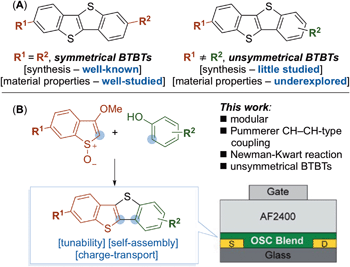 |
| Scheme 1 (A) Comparison of symmetrical and unsymmetrical BTBT compounds. (B) This work: the synthesis and properties of underexplored unsymmetrical BTBT compounds. OSC = organic semiconductor. | |
Unsymmetrical BTBT compounds have the potential to outperform symmetrical BTBTs; for example, Hanna and coworkers have reported mobilities as high as 14.7 cm2 V−1 s−1 for a liquid crystalline 2-decyl-7-phenyl-BTBT.13 Despite these promising results, the investigation of unsymmetrical BTBT derivatives lags behind that of their symmetrical counterparts (Scheme 1A).14 This is in part due to a lack of efficient methods for their synthesis. For example, most routes towards unsymmetrical BTBTs are limited in scope and/or use transition metals during the final step of the synthesis – leading to metallic impurities that are known to affect the performance of organic materials.15,16 As there are few general approaches for the synthesis of unsymmetrical BTBTs,17 we considered a modular synthesis, less reliant on the use of transition metals, that would grant access to a variety of compounds and allow facile exploration of their material properties (Scheme 1B).
Here we describe a versatile, modular synthesis of unsymmetrical BTBT compounds (Scheme 1B). Key to our approach is the application of a transition-metal free Pummerer CH–CH-type cross-coupling in conjunction with a Newman–Kwart rearrangement. Exploiting the new approach, we investigated the material properties of a selected series of unsymmetrical BTBT derivatives containing a decane aliphatic side chain at the C2 position (required for solubility), and a variety of electron donating and electron withdrawing groups at the C7 position. Tuning the substituent at the C7 position influenced the electronic properties and solid-state assembly of the BTBTs. The BTBT molecules were blended with a polymer semiconductor binder, PIDTBT to aid processing into thin-films in small-molecule:polymer (S-M:polymer) blend transistors. Preliminary investigations into the charge transport properties highlighted the importance of molecular ordering of the BTBT molecules during phase segregation and matching of the highest occupied molecular orbital (HOMO) level with that of the polymer semiconductor binder. Crucially, hole mobilities extracted from devices fabricated using phenyl and methoxy functionalized unsymmetrical BTBT molecules blended with PIDTBT were higher than those extracted from comparable devices fabricated using pristine PIDTBT or the symmetrical C8-BTBT in a S-M:polymer blend transistor.
Results and discussion
We have recently developed Pummerer CH–CH-type cross-coupling processes18 that exploit activation of the benzothiophene partner by convenient S-oxidation and deliver functionalized benzothiophenes.19 We reasoned that this new metal-free cross-coupling process could provide modular access to underexplored BTBT materials. Our investigation began with the metal-free coupling of benzothiophenes, activated as their S-oxides, 1 with phenols 2. Accordingly, cross-coupled products 3 bearing various functionalities were efficiently prepared (Scheme 2). Notably, bromo-substituents were tolerated on the biaryl scaffold (3ac, 3bb–3bg), thus allowing further transformations. Electron-withdrawing (3bg, 3eg) and electron-donating (3bf, 3ef, 3eh) substituents were compatible with the process, thus providing an opportunity to tune the electronic properties of the target BTBT materials.
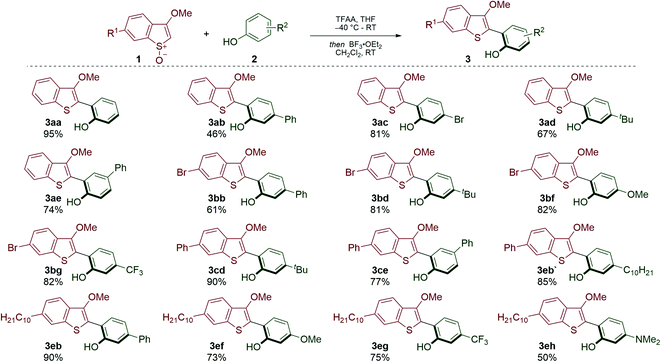 |
| Scheme 2 Pummerer CH–CH-type couplings of benzothiophene S-oxides and phenols. Reaction conditions: 1 (1.0 equiv.), 2 (1.5 equiv.), TFAA (1.5 equiv.), THF (0.1 M), −40 °C to RT, then BF3·OEt2 (0.2 equiv.), CH2Cl2, 0.1 M, RT. | |
The conversion of the coupling products 3 into the desired BTBT products 4 involved a Newmann–Kwart reaction, to give intermediates 5,20 followed by cyclization (Scheme 3A). While this method can deliver symmetrical products (e.g.4aa), we focused on preparing more elusive unsymmetrical BTBT materials. The position of the substituents around the BTBT core was easily altered by the choice of phenol coupling partner 2; for example, regioisomers 4ab and 4ae were obtained using the same synthetic route but selecting either meta- or para-substituted phenol partners. As a variety of substituted benzothiophene and phenol partners are commercially available, this flexibility will prove useful when planning the synthesis of target unsymmetrical BTBT materials. The adaptability of our approach was also demonstrated in the synthesis of 4eb (Scheme 3B). This BTBT material was prepared by parallel routes from either benzothiophene S-oxide 1e and phenol 2b, or 1c and 2i (via3eb or 3eb′ respectively, see Scheme 2). We were particularly attracted to the synthesis of BTBT 4eb as it has displayed high charge mobility (14.7 cm2 V−1 s−1).13 Therefore, we prepared a range of related derivatives (4ef–4eh) to investigate how substituents affect the properties of these unsymmetrical materials.
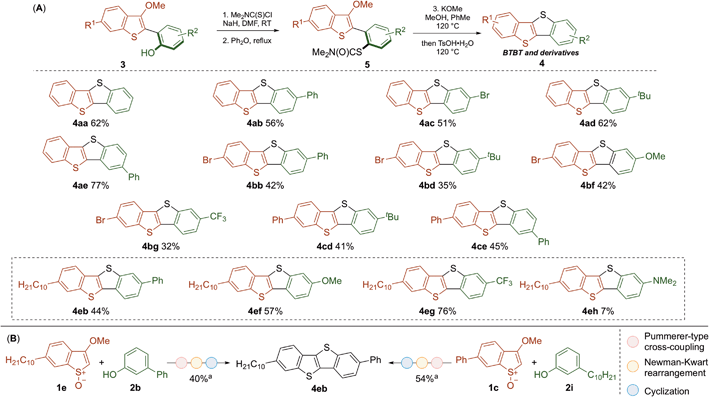 |
| Scheme 3 (A) Scope of the metal-free synthesis of BTBT materials by Newman–Kwart reaction of coupling products 3, followed by cyclization. Yields are of the overall process from 3 to 4. (B) Parallel synthesis of 4eb from 1e and 2b, and 1c and 2i. aIsolated yield for the overall process from 1 and 2. Reaction conditions for 3 to 5: 3 (1.0 equiv.), Me2NC(S)Cl (2.0 equiv.), NaH (3.0 equiv.), DMF (0.1 M), RT·Ph2O (0.1 M), reflux. Reaction conditions for 5 to 4: 5 (1 equiv.), KOMe (2.0 equiv.), MeOH/PhMe (1 : 1, 0.05 M), 120 °C, then TsOH·H2O (8.0 equiv.), 120 °C. | |
With a range of new BTBT derivatives in hand, we firstly examined the thermal properties of the selected BTBT derivatives (4eb, 4ef–4eh) using differential scanning calorimetry (DSC). As previously reported,134eb exhibited liquid crystal phase transitions of SmE at 143 °C and 79 °C, and SmA at 212 °C and 210 °C during the heating and cooling cycle, respectively (Fig. 1a). The typical smooth fan-shaped texture of the fluid SmA phase and the striated fan-like one of the soft crystal SmE phase were confirmed by polarized microscopy (POM) (ESI Fig. S1†). In contrast, 4ef, 4eg and 4eh showed typical behaviour of crystalline material in the DSC curves and this was supported by the POM images. BTBT 4ef showed multiple phase transitions with a large sharp transition enthalpy at 114 °C upon cooling from an isotropic phase, with a second smaller peak at 107 °C; this is most likely a 2nd polymorphic phase (Fig. 1b). On the other hand, the DSC curves for 4eg and 4eh only show sharp melting and crystallization peaks upon heating and cooling (Fig. 1c and d). Next, the energy levels of the selected unsymmetrical BTBT derivatives (4eb, 4ef–4eh) were investigated using cyclic voltammetry; Fig. 1e shows the respective energy levels. The highest occupied molecular orbital (HOMO) levels were estimated from the onset of the oxidation peak (Eoxdonset) in the cyclic voltammogram of the BTBT molecules (Fig. S2†). The HOMO level obtained for 4eb is similar to reported values; EHOMO = −5.43 eV.13 It is evident that the type of functional group at the C7 position influences the HOMO level of the unsymmetrical BTBT scaffold. The electron donating ability of the –NMe2 and –OMe groups resulted in higher HOMO levels where EHOMO = −5.37 and −4.94 eV for 4ef and 4eh, respectively, while the electron withdrawing group (–CF3) on 4eg decreases its HOMO level to EHOMO = −5.86 eV.21 The trend observed is qualitatively confirmed by values calculated using density functional theory (Fig. S2†).
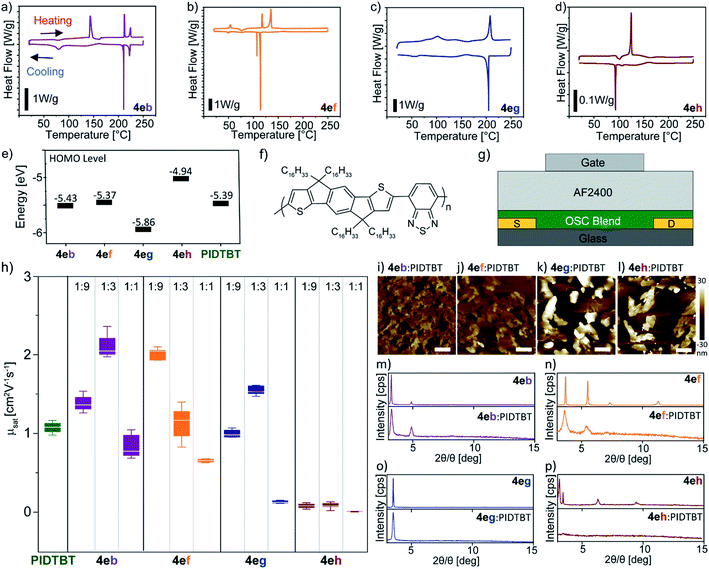 |
| Fig. 1 Thermal and electrochemical properties of the BTBT molecules: DSC thermograms taken from 2nd heating and cooling cycle: (a) 4eb (R2 = Ph); (b) 4ef (R2 = OMe); (c) 4eg (R2 = CF3); (d) 4eh (R2 = NMe2) at 5 °C min−1; (e) energy level diagram indicating HOMO levels including PIDTBT; (f) molecular structure of the polymer semiconductor PIDTBT; (g) top-gated OFET device structure with an aluminium gate electrode, AF2400 as the gate dielectric, and gold source-drain electrodes with a channel width and length of 1000 mm and 60 mm, respectively; (h) box and whiskers plot comparing the saturated mobility (μsat) from measured devices containing pristine PIDTBT and blends of 4e(b,f,g,h):PIDTBT at a ratio of 1 : 9, 1 : 3, 1 : 1; atomic force microscopy (AFM) topography images of the 4e(b,f,g,h):PIDTBT blend films at 1 : 3 ratio processed from tetralin:chlorobenzene (i–l). The white scale bar indicates a length of 2 μm; grazing incidence X-ray diffraction of thin films based on individual BTBT derivatives (top) and as a blend with PIDTBT (bottom): (m) 4eb; (n) 4ef; (o) 4eg; (p) 4eh. | |
Initial attempts at reproducing transistor performance using adapted reported conditions for 4eb resulted in low μsat = 1.59 cm2 V−1 s−1, and we were unable to reproduce the reported high mobilities of 14.7 cm2 V−1 s−1 on bottom-gate/top-contact transistors (Si/SiO2/4eb/Au).13 Fig. S3† shows the relevant transistor characteristics. Furthermore, solution processing the remaining BTBT derivatives resulted in largely non-uniform films (Fig. S4†) that led to inconsistent transistor behaviour. To improve the film forming properties of the unsymmetrical BTBT derivatives, and to investigate their charge-carrier properties, a polymer semiconductor PIDTBT was used as a binder (Fig. 1f). This approach is based on recent reports of S-M:polymer blend transistors of symmetrical C8-BTBT:PIDTBT that exploit the highly ordered nature of small-molecules for efficient charge transport and the superior film forming properties of the polymer binder.12,22 Top-gated OFETs using poly[4,5-difluoro-2,2bis(trifluoromethyl)-1,3-dioxole-co-tetrafluoroethylene] (Teflon AF2400) as a dielectric were fabricated (Fig. 1g) to assess the charge transport properties of OSC blends of 4e(b,f,g,h):PIDTBT. We performed preliminary optimization of the blend ratio at 1
:
9, 1
:
3, and 1
:
1. As controls, transistors based on pristine PIDTBT and its blend with the prototypical symmetrical C8-BTBT were fabricated, and their performance evaluated. The BTBT molecules and PIDTBT were dissolved in tetralin and chlorobenzene separately before mixing into a blend solution at the respective ratios for thin-film processing. The calculated mobility values μsat for all devices were taken in the saturation regime at VGS = −60 V from the corresponding mobility dependence on applied gate voltage plot extracted from the transfer and output characteristics (Fig. S5–S10†). The overall transistor parameters (Vth and Ion/Ioff) are summarized in Table S1 (see the ESI† for further details of device fabrication).
A summary of the μsat values extracted from devices containing pristine PIDTBT and 4e(b,f,g,h):PIDTBT are shown in Fig. 1h, and the mobility values for C8-BTBT:PIDTBT are highlighted in Table S1.† In general, all devices for blends at higher amounts of the unsymmetrical and symmetrical BTBT molecules – i.e. 1
:
1 ratio – had the lowest mobility (<0.8 cm2 V−1 s−1) (Table S1†). A similar μsat trend in the blend ratio was also reported in earlier work for C8-BTBT:PIDTBT.12e Amongst the unsymmetrical BTBT molecules, blends using 4eh gave the lowest mobilities (<0.08 cm2 V−1 s−1) across all ratios. On the other hand, blends with 4eb and 4ef at a ratio of 1
:
3 and 1
:
9 achieved the highest mobility of 1.89 ± 0.3 cm2 V−1 s−1 and 1.87 ± 0.2 cm2 V−1 s−1, respectively. Devices from pristine PIDTBT obtained mobilities of 1.02 ± 0.1 cm2 V−1 s−1 which indicate that any improvements are due to the presence of unsymmetrical BTBT molecules in the OSC blends. Furthermore, the mobility of the best performing unsymmetrical BTBT blends were higher than comparable devices containing the symmetrical C8-BTBT which obtained a mobility of 1.19 ± 0.3 cm2 V−1 s−1 at a 1
:
3 blend ratio (Table S1†). Substantial improvements in the measured mobilities could be achieved by the use of secondary dopants in ternary blend devices, an approach that has been previously reported for blends of PIDTBT with the symmetrical C8-BTBT semiconductor.12,22
To gain insights into the differences in device performance, atomic force microscopy (AFM) topography analysis (Fig. 1i–l) and grazing incidence X-ray diffraction (GIXD) experiments (Fig. 1m–p) were performed. AFM analysis showed aggregates forming on the top-layer of the blend film with varying morphologies depending on the BTBT molecule. The size and shape of these aggregates are consistent with the morphology of pristine BTBT films and are distinct from the amorphous topography of pristine PIDTBT (Fig. S11†). This indicates that the BTBT molecules vertically phase segregate from the polymer during thin-film formation. As highlighted by reports on C8-BTBT:PIDTBT, a vertically phase separated blend morphology consisting of highly ordered domains of the small-molecules on the upper surface of the film, interfacing with the dielectric layer (i.e. the conduction channel) in a top-gate device, is crucial for efficient charge transport.12,22
AFM images of 4eb/4ef:PIDTBT (Fig. 1i and j) show a connected terrace-like morphology which is in line with previous reports on unsymmetrical BTBT molecules.13,15d GIXD of 4eb/4ef:PIDTBT films revealed crystalline peaks at 2θ/θ = 3.3° and 4.9° for 4eb, and 2θ/θ = 3.6° and 5.4° for 4ef which were similarly observed in the diffraction of their respective pristine films (Fig. 1k and l). This indicates that the BTBT molecules form ordered connected domains in the blend. On the other hand, the BTBT molecules in films of 4eg/4eh:PIDTBT (Fig. 1o and p) lead to large disconnected aggregates of the BTBT molecules. Here, the GIXD for 4eg has the same peak at 2θ/θ = 3.5° in the pristine and blend films indicating that the aggregates of 4eg are ordered but disconnected, while no clear peaks were observed in the diffraction of blends with 4eh suggesting the formation of large, disordered aggregates. Based on this observation, the higher μsat values in 4eb/4ef:PIDTBT devices are a result of better charge transport within the connected, ordered domains of the BTBT molecules on the top surface of the blend films. In addition, the HOMO levels of 4eb and 4ef closely match that of PIDTBT (Fig. 1e); this is crucial in minimizing energetic disorder for hole transport between the crystalline domains of small-molecules and the amorphous polymer.12e,23
Conclusions
In summary, we have developed a modular synthetic approach to the unsymmetrical BTBT scaffold; an underexplored architecture in molecular semiconductors. The BTBT materials are prepared from readily available partners using a metal-free, Pummerer CH–CH-type cross-coupling followed by a Newman–Kwart reaction of the coupling products. Access to unsymmetric BTBT structures permitted the study of their material properties; varying the functional groups attached to the conjugated core of the BTBT scaffold modulated the molecular orbital energy levels and self-assembly properties. Preliminary investigation into the structure–property relationships of the unsymmetrical BTBT molecules in S-M:polymer blend transistors highlighted the influence of molecular structure on the charge transport ability in thin-films. We showed that devices fabricated using the phenyl and methoxy functionalized unsymmetrical BTBT molecules show higher hole mobilities than comparable devices fabricated using pristine PIDTBT or blends with the symmetrical C8-BTBT. Improved access to unsymmetrical BTBT molecular semiconductors using a modular synthetic approach provides a foundation for future studies targeting improved transistor performance, for example, through the use of secondary dopants such as fluorinated fullerene derivative, C60F48 or molecular Lewis acids, B(C6F5)3 and [Zn(C6F5)2] in ternary blends that have been widely studied in PIDTBT blends containing the symmetrical C8-BTBT.12,22
Data availability
Crystallographic data for 4ef has been deposited at the CCDC under 2103832.
Author contributions
Experiments were conceived and designed by M. T., A. R., G. J. P. P., M. L. T. and D. J. P. and executed by all co-authors. M. T. and G. J. P. P. synthesised and characterised the BTBT molecules. D. J. T. and R. M.-H. synthesised and characterised the polymer semiconductor PIDTBT. A. R., R. U. K., S. A., A. Z. performed the material characterization (DSC, CV, AFM) and the transistor fabrication and analysis. Y. S. and I. D. performed the POM experiment and analysis of the BTBT molecules. Y. J. performed the DFT calculations. I. V.-Y. acquired the XRD and single crystal measurements. M. T., A. R., G. J. P. P., M. L. T. and D. J. P. wrote the paper. M. L. T. and D. J. P. supervised the work. All authors contributed to the finalization of the paper.
Conflicts of interest
There are no conflicts to declare.
Acknowledgements
The authors gratefully acknowledge financial support from The Uehara Memorial Foundation (Fellowship to M. T.) and the Engineering and Physical Sciences Research Council (EPSRC) through the Centre for Innovative Manufacturing in Large Area Electronics (CIMLAE, program grant EP/K03099X/1) and the iUK grant PlasticARMPIT (103390). R. M.-H. acknowledges the support of a Consejo Nacional de Ciencia y Tecnología (CONACYT) Mexico Scholarship (478743) and R. U. K. an EPSRC Doctoral Training Program Health Care Technology Studentship. We thank the University of Manchester (Lectureship to G. J. P. P.) for their generous support.
Notes and references
- A. F. Paterson, S. Singh, K. J. Fallon, T. Hodsden, Y. Han, B. C. Schroeder, H. Bronstein, M. Heeney, I. McCulloch and T. D. Anthopoulos, Recent Progress in High-Mobility Organic Transistors: A Reality Check, Adv. Mater., 2018, 30, 1801079 CrossRef PubMed.
-
(a) O. Inganäs, Organic Photovoltaics over Three Decades, Adv. Mater., 2018, 30, 1800388 CrossRef PubMed;
(b) L. Yao, A. Rahmanudin, N. Guijarro and K. Sivula, Organic Semiconductor Based Devices for Solar Water Splitting, Adv. Energy Mater., 2018, 8, 1802585 CrossRef.
- J. Borges-González, C. J. Kousseff and C. B. Nielsen, Organic Semiconductors for Biological Sensing, J. Mater. Chem. C, 2019, 7, 1111–1130 RSC.
- H. Bronstein, C. B. Nielsen, B. C. Schroeder and I. McCulloch, The Role of Chemical Design in the Performance of Organic Semiconductors, Nat. Rev. Chem., 2020, 4, 66–77 CrossRef CAS.
- A. Rahmanudin, L. Yao and K. Sivula, Conjugation Break Spacers and Flexible Linkers as Tools to Engineer the Properties of Semiconducting Polymers, Polym. J., 2018, 50, 725–736 CrossRef CAS.
- Y. Diao, L. Shaw, Z. Bao and S. C. B. Mannsfeld, Morphology Control Strategies for Solution-Processed Organic Semiconductor Thin Films, Energy Environ. Sci., 2014, 7, 2145–2159 RSC.
- K. Takimiya, I. Osaka, T. Mori and M. Nakano, Organic Semiconductors Based on [1]Benzothieno[3,2-b][1]Benzothiophene Substructure, Acc. Chem. Res., 2014, 47, 1493–1502 CrossRef CAS PubMed.
- Z. Ma, H. Geng, D. Wang and Z. Shuai, Influence of Alkyl Side-Chain Length on the Carrier Mobility in Organic Semiconductors: Herringbone vs. Pi–Pi Stacking, J. Mater. Chem. C, 2016, 4, 4546–4555 RSC.
- H. Ebata, T. Izawa, E. Miyazaki, K. Takimiya, M. Ikeda, H. Kuwabara and T. Yui, Highly Soluble [1]Benzothieno[3,2-b]Benzothiophene (BTBT) Derivatives for High-Performance, Solution-Processed Organic Field-Effect Transistors, J. Am. Chem. Soc., 2007, 129, 15732–15733 CrossRef CAS PubMed.
- K. Takimiya, H. Ebata, K. Sakamoto, T. Izawa, T. Otsubo and Y. Kunugi, 2,7-Diphenyl[1]Benzothieno[3,2-b]Benzothiophene, A New Organic Semiconductor for Air-Stable Organic Field-Effect Transistors with Mobilities up to 2.0 cm2 V−1 s−1, J. Am. Chem. Soc., 2006, 128, 12604–12605 CrossRef CAS PubMed.
- Y. Yuan, G. Giri, A. L. Ayzner, A. P. Zoombelt, S. C. B. Mannsfeld, J. Chen, D. Nordlund, M. F. Toney, J. Huang and Z. Bao, Ultra-High Mobility Transparent Organic Thin Film Transistors Grown by an off-Centre Spin-Coating Method, Nat. Commun., 2014, 5, 3005 CrossRef PubMed.
-
(a) A. D. Scaccabarozzi, F. Scuratti, A. J. Barker, A. Basu, A. F. Paterson, Z. Fei, O. Solomeshch, A. Petrozza, N. Tessler, M. Heeney, T. D. Anthopoulos and M. Caironi, Understanding Charge Transport in High-Mobility P-Doped Multicomponent Blend Organic Transistors, Adv. Electron. Mater., 2020, 6, 2000539 CrossRef CAS;
(b) A. F. Paterson, L. Tsetseris, R. Li, A. Basu, H. Faber, A.-H. Emwas, J. Panidi, Z. Fei, M. R. Niazi, D. H. Anjum, M. Heeney and T. D. Anthopoulos, Addition of the Lewis Acid Zn(C6F5)2 Enables Organic Transistors with a Maximum Hole Mobility in Excess of 20 cm2 V−1 s−1, Adv. Mater., 2019, 31, 1900871 CrossRef PubMed;
(c) J. Panidi, A. F. Paterson, D. Khim, Z. Fei, Y. Han, L. Tsetseris, G. Vourlias, P. A. Patsalas, M. Heeney and T. D. Anthopoulos, Remarkable Enhancement of the Hole Mobility in Several Organic Small-Molecules, Polymers, and Small-Molecule:Polymer Blend Transistors by Simple Admixing of the Lewis Acid p-Dopant B(C6F5)3, Adv. Sci., 2018, 5, 1700290 CrossRef PubMed;
(d) A. M. Zeidell, C. Tyznik, L. Jennings, C. Zhang, H. Lee, M. Guthold, Z. V. Vardeny and O. D. Jurchescu, Enhanced Charge Transport in Hybrid Perovskite Field-Effect Transistors via Microstructure Control, Adv. Electron. Mater., 2018, 4, 1800316 CrossRef;
(e) A. F. Paterson, N. D. Treat, W. Zhang, Z. Fei, G. Wyatt-Moon, H. Faber, G. Vourlias, P. A. Patsalas, O. Solomeshch, N. Tessler, M. Heeney and T. D. Anthopoulos, Small Molecule/Polymer Blend Organic Transistors with Hole Mobility Exceeding 13 cm2 V−1 s−1, Adv. Mater., 2016, 28, 7791–7798 CrossRef CAS PubMed.
- H. Iino, T. Usui and J. Hanna, Liquid Crystals for Organic Thin-Film Transistors, Nat. Commun., 2015, 6, 6828 CrossRef CAS PubMed.
- For select reports on the material properties of symmetrical BTBTs, see ref. 9 and:
(a) M.-C. Um, J. Kwak, J.-P. Hong, J. Kang, D. Y. Yoon, S. H. Lee, C. Lee and J.-I. Hong, High-Performance Organic Semiconductors for Thin-Film Transistors Based on 2,7-Divinyl[1]Benzothieno[3,2-b]Benzothiophene, J. Mater. Chem., 2008, 18, 4698–4703 RSC;
(b) T. Izawa, H. Mori, Y. Shinmura, M. Iwatani, E. Miyazaki, K. Takimiya, H.-W. Hung, M. Yahiro and C. Adachi, Molecular Modification of 2,7-Diphenyl[1]Benzothieno[3,2-b]Benzothiophene (DPh-BTBT) with Diarylamino Substituents: From Crystalline Order to Amorphous State in Evaporated Thin Films, Chem. Lett., 2009, 38, 420–421 CrossRef CAS;
(c) K. H. Jung, K. H. Kim, D. H. Lee, D. S. Jung, C. E. Park and D. H. Choi, Liquid Crystalline Dialkyl-Substituted Thienylethenyl [1]Benzothieno[3,2-b]Benzothiophene Derivatives for Organic Thin Film Transistors, Org. Electron., 2010, 11, 1584–1593 CrossRef CAS;
(d) A. Kim, K.-S. Jang, J. Kim, J. C. Won, M. H. Yi, H. Kim, D. K. Yoon, T. J. Shin, M.-H. Lee, J.-W. Ka and Y. H. Kim, Solvent-Free Directed Patterning of a Highly Ordered Liquid Crystalline Organic Semiconductor via Template-Assisted Self-Assembly for Organic Transistors, Adv. Mater., 2013, 25, 6219–6225 CrossRef CAS PubMed;
(e) V. S. Vyas, R. Gutzler, J. Nuss, K. Kern and B. V. Lotsch, Optical Gap in Herringbone and π-Stacked Crystals of [1]Benzothieno[3,2-b]Benzothiophene and its Brominated Derivative, CrystEngComm, 2014, 16, 7389–7392 RSC;
(f) C. Niebel, Y. Kim, C. Ruzié, J. Karpinska, B. Chattopadhyay, G. Schweicher, A. Richard, V. Lemaur, Y. Olivier, J. Cornil, A. R. Kennedy, Y. Diao, W.-Y. Lee, S. Mannsfeld, Z. Bao and Y. H. Geerts, Thienoacene Dimers Based on the Thieno[3,2-b]Thiophene Moiety: Synthesis, Characterization and Electronic Properties, J. Mater. Chem. C, 2015, 3, 674–685 RSC;
(g) J. Tsutsumi, S. Matsuoka, S. Inoue, H. Minemawari, T. Yamada and T. Hasegawa,
N-Type Field-Effect Transistors Based on Layered Crystalline Donor–Acceptor Semiconductors with Dialkylated Benzothienobenzothiophenes as Electron Donors, J. Mater. Chem. C, 2015, 3, 1976–1981 RSC;
(h) T. Higashino, M. Dogishi, T. Kadoya, R. Sato, T. Kawamoto and T. Mori, Air-Stable n-Channel Organic Field-Effect Transistors Based on Charge-Transfer Complexes Including Dimethoxybenzothienobenzothiophene and Tetracyanoquinodimethane Derivatives, J. Mater. Chem. C, 2016, 4, 5981–5987 RSC;
(i) Y. Kiyota, T. Kadoya, K. Yamamoto, K. Iijima, T. Higashino, T. Kawamoto, K. Takimiya and T. Mori, Benzothienobenzothiophene-Based Molecular Conductors: High Conductivity, Large Thermoelectric Power Factor, and One-Dimensional Instability, J. Am. Chem. Soc., 2016, 138, 3920–3925 CrossRef CAS PubMed;
(j) C. Ruzié, J. Karpinska, A. Laurent, L. Sanguinet, S. Hunter, T. D. Anthopoulos, V. Lemaur, J. Cornil, A. R. Kennedy, O. Fenwick, P. Samorì, G. Schweicher, B. Chattopadhyay and Y. H. Geerts, Design, Synthesis, Chemical Stability, Packing, Cyclic Voltammetry, Ionisation Potential, and Charge Transport of [1]Benzothieno[3,2-b][1]Benzothiophene Derivatives, J. Mater. Chem. C, 2016, 4, 4863–4879 RSC;
(k) D. E. Martínez-Tong, G. Gbabode, C. Ruzié, B. Chattopadhyay, G. Schweicher, A. R. Kennedy, Y. H. Geerts and M. Sferrazza, Self-Assembled π-Conjugated Organic Nanoplates: From Hexagonal to Triangular Motifs, RSC Adv., 2016, 6, 44921–44931 RSC;
(l) M. Matsumura, A. Muranaka, R. Kurihara, M. Kanai, K. Yoshida, N. Kakusawa, D. Hashizume, M. Uchiyama and S. Yasuike, General Synthesis, Structure, and Optical Properties of Benzothiophene-Fused Benzoheteroles Containing Group 15 and 16 Elements, Tetrahedron, 2016, 72, 8085–8090 CrossRef CAS;
(m) G. H. Roche, Y.-T. Tsai, S. Clevers, D. Thuau, F. Castet, Y. H. Geerts, J. J. E. Moreau, G. Wantz and O. J. Dautel, The Role of H-Bonds in the Solid State Organization of [1]Benzothieno[3,2-b][1]Benzothiophene (BTBT) Structures: Bis(Hydroxy-Hexyl)-BTBT, as a Functional Derivative Offering Efficient Air Stable Organic Field Effect Transistors (OFETs), J. Mater. Chem. C, 2016, 4, 6742–6749 RSC;
(n) P. Tisovský, A. Gáplovský, K. Gmucová, M. Novota, M. Pavúk and M. Weis, Synthesis and Characterization of New [1]Benzothieno[3,2-b]Benzothiophene Derivatives with Alkyl-Thiophene Core for Application in Organic Field-Effect Transistors, Org. Electron., 2019, 68, 121–128 CrossRef;
(o) Y. Wang, M. Chang, X. Ke, X. Wan and G. Yang, A New Medium-Bandgap Fused-[1]Benzothieno[3,2-b][1]Benzo-Thiophene (BTBT) Nonfullerene Acceptor for Organic Solar Cells with High Open-Circuit Voltage, Polymer, 2019, 185, 121976 CrossRef CAS;
(p) G. Cai, J. Zhu, Y. Xiao, M. Li, K. Liu, J. Wang, W. Wang, X. Lu, Z. Tang, J. Lian, P. Zeng, Y. Wang and X. Zhan, Fused Octacyclic Electron Acceptor Isomers for Organic Solar Cells, J. Mater. Chem. A, 2019, 7, 21432–21437 RSC;
(q) M. Mohankumar, B. Chattopadhyay, R. Hadji, L. Sanguinet, A. R. Kennedy, V. Lemaur, J. Cornil, O. Fenwick, P. Samorì and Y. Geerts, Oxacycle-Fused [1]Benzothieno[3,2-b][1]Benzothiophene Derivatives: Synthesis, Electronic Structure, Electrochemical Properties, Ionisation Potential, and Crystal Structure, ChemPlusChem, 2019, 84, 1263–1269 CrossRef CAS PubMed;
(r) H. Usta, D. Kim, R. Ozdemir, Y. Zorlu, S. Kim, M. C. Ruiz Delgado, A. Harbuzaru, S. Kim, G. Demirel, J. Hong, Y.-G. Ha, K. Cho, A. Facchetti and M.-G. Kim, High Electron Mobility in [1]Benzothieno[3,2-b][1]Benzothiophene-Based Field-Effect Transistors: Toward n-Type BTBTs, Chem. Mater., 2019, 31, 5254–5263 CrossRef CAS.
- For select reports on the synthesis and material properties of unsymmetrical BTBTs, see:
(a) M. Kienle, A. Unsinn and P. Knochel, Synthesis of Dibenzothiophenes and Related Classes of Heterocycles by Using Functionalized Dithiocarbamates, Angew. Chem., Int. Ed., 2010, 49, 4751–4754 CrossRef CAS PubMed;
(b) K. Chernichenko, N. Emelyanov, I. Gridnev and V. G. Nenajdenko, Unusual Thiophilic Ring-Opening of Fused Oligothiophenes with Organolithium Reagents, Tetrahedron, 2011, 67, 6812–6818 CrossRef CAS;
(c) T. Mori, T. Nishimura, T. Yamamoto, I. Doi, E. Miyazaki, I. Osaka and K. Takimiya, Consecutive Thiophene-Annulation Approach to π-Extended Thienoacene-Based Organic Semiconductors with [1]Benzothieno[3,2-b][1]Benzothiophene (BTBT) Substructure, J. Am. Chem. Soc., 2013, 135, 13900–13913 CrossRef CAS PubMed;
(d) S. Minami, K. Hirano, T. Satoh and M. Miura, Synthesis of [1]Benzothieno[3,2-b][1]Benzothiophene (BTBT) and Its Higher Homologs through Palladium-Catalyzed Intramolecular Decarboxylative Arylation, Tetrahedron Lett., 2014, 55, 4175–4177 CrossRef CAS;
(e) C. M. S. Combe, L. Biniek, B. C. Schroeder and I. McCulloch, Synthesis of [1]Benzothieno[3,2-b][1]Benzothiophene Pendant and Norbornene Random Co-Polymers via Ring Opening Metathesis, J. Mater. Chem. C, 2014, 2, 538–541 RSC;
(f) G. Schweicher, V. Lemaur, C. Niebel, C. Ruzié, Y. Diao, O. Goto, W.-Y. Lee, Y. Kim, J.-B. Arlin, J. Karpinska, A. R. Kennedy, S. R. Parkin, Y. Olivier, S. C. B. Mannsfeld, J. Cornil, Y. H. Geerts and Z. Bao, Bulky End-Capped [1]Benzothieno[3,2-b]Benzothiophenes: Reaching High-Mobility Organic Semiconductors by Fine Tuning of the Crystalline Solid-State Order, Adv. Mater., 2015, 27, 3066–3072 CrossRef CAS PubMed;
(g) S. Vásquez-Céspedes, A. Ferry, L. Candish and F. Glorius, Heterogeneously Catalyzed Direct C-H Thiolation of Heteroarenes, Angew. Chem., Int. Ed., 2015, 54, 5772–5776 CrossRef PubMed;
(h) Y. He, W. Xu, I. Murtaza, D. Zhang, C. He, Y. Zhu and H. Meng, Molecular Phase Engineering of Organic Semiconductors Based on a [1]Benzothieno[3,2-b][1]Benzothiophene Core, RSC Adv., 2016, 6, 95149–95155 RSC;
(i) A. L. Capodilupo, E. Fabiano, L. De Marco, G. Ciccarella, G. Gigli, C. Martinelli and A. Cardone, [1]Benzothieno[3,2-b]Benzothiophene-Based Organic Dyes for Dye-Sensitized Solar Cells, J. Org. Chem., 2016, 81, 3235–3245 CrossRef CAS PubMed;
(j) Y. He, M. Sezen, D. Zhang, A. Li, L. Yan, H. Yu, C. He, O. Goto, Y.-L. Loo and H. Meng, High Performance OTFTs Fabricated Using a Calamitic Liquid Crystalline Material of 2-(4-Dodecyl Phenyl)[1]Benzothieno[3,2-b][1]Benzothiophene, Adv. Electron. Mater., 2016, 2, 1600179 CrossRef;
(k) M. Wang, J. Wei, Q. Fan and X. Jiang, Cu(II)-Catalyzed Sulfide Construction: Both Aryl Groups Utilization of Intermolecular and Intramolecular Diaryliodonium Salt, Chem. Commun., 2017, 53, 2918–2921 RSC;
(l) T. Higashino, A. Ueda, J. Yoshida and H. Mori, Improved Stability of a Metallic State in Benzothienobenzothiophene-Based Molecular Conductors: An Effective Increase of Dimensionality with Hydrogen Bonds, Chem. Commun., 2017, 53, 3426–3429 RSC;
(m) O. V. Borshchev, A. S. Sizov, E. V. Agina, A. A. Bessonov and S. A. Ponomarenko, Synthesis of Organosilicon Derivatives of [1]Benzothieno[3,2-b][1]-Benzothiophene for Efficient Monolayer Langmuir–Blodgett Organic Field Effect Transistors, Chem. Commun., 2017, 53, 885–888 RSC;
(n) H. Monobe, L. An, P. Hu, B.-Q. Wang, K.-Q. Zhao and Y. Shimizu, Charge Transport Property of Asymmetric Alkyl-BTBT LC Semiconductor Possessing a Fluorophenyl Group, Mol. Cryst. Liq. Cryst., 2017, 647, 119–126 CrossRef CAS;
(o) C. Yao, X. Chen, Y. He, Y. Guo, I. Murtaza and H. Meng, Design and Characterization of Methoxy Modified Organic Semiconductors Based on Phenyl[1]Benzothieno[3,2-b][1]Benzothiophene, RSC Adv., 2017, 7, 5514–5518 RSC;
(p) Y. He, S. Guo, Y. He, I. Murtaza, A. Li, X. Zeng, Y. Guo, Y. Zhao, X. Chen and H. Meng, Investigating the Thermal Stability of Organic Thin-Film Transistors and Phototransistors Based on [1]-Benzothieno-[3,2-b]-[1]-Benzothiophene Dimeric Derivatives, Chem.–Eur. J., 2018, 24, 16595–16602 CrossRef CAS PubMed;
(q) K. He, W. Li, H. Tian, J. Zhang, D. Yan, Y. Geng and F. Wang, Asymmetric Conjugated Oligomers Based on Polycyclic Aromatics as High Mobility Semiconductors: The Influence of Chalcogens, Org. Electron., 2018, 57, 359–366 CrossRef CAS;
(r) S. Chen, M. Wang and X. Jiang, Pd-Catalyzed C-S Cyclization via C-H Functionalization Strategy: Access to Sulfur-Containing Benzoheterocyclics, Chin. J. Chem., 2018, 36, 921–924 CrossRef CAS;
(s) K. Li, A. Yu and X. Meng, Synthesis of Dibenzothiophene and 1,4-Dihydrodibenzothiophene Derivatives via Allylic Phosphonium Salt Initiated Domino Reactions, Org. Lett., 2018, 20, 1106–1109 CrossRef CAS PubMed;
(t) M. R. Reddy, H. Kim, C. Kim and S. Seo, 2-Thiopene[1]Benzothieno[3,2-b]Benzothiophene Derivatives as Solution-Processable Organic Semiconductors for Organic Thin-Film Transistors, Synth. Met., 2018, 235, 153–159 CrossRef CAS;
(u) A. Sanzone, S. Mattiello, G. M. Garavaglia, A. M. Calascibetta, C. Ceriani, M. Sassi and L. Beverina, Efficient Synthesis of Organic Semiconductors by Suzuki–Miyaura Coupling in an Aromatic Micellar Medium, Green Chem., 2019, 21, 4400–4405 RSC;
(v) G. Zanotti, N. Angelini, G. Mattioli, A. M. Paoletti, G. Pennesi, D. Caschera, A. P. Sobolev, L. Beverina, A. M. Calascibetta, A. Sanzone, A. Di Carlo, B. Berionni Berna, S. Pescetelli and A. Agresti, [1]Benzothieno[3,2-b][1]Benzothiophene-Phthalocyanine Derivatives: A Subclass of Solution-Processable Electron-Rich Hole Transport Materials, ChemPlusChem, 2020, 85, 2376–2386 CrossRef CAS PubMed;
(w) R. Ozdemir, K. Ahn, İ. Deneme, Y. Zorlu, D. Kim, M.-G. Kim and H. Usta, Engineering Functionalized Low LUMO [1]Benzothieno[3,2-b][1]Benzothiophenes (BTBTs): Unusual Molecular and Charge Transport Properties, J. Mater. Chem. C, 2020, 8, 15253–15267 RSC;
(x) A. M. Calascibetta, S. Mattiello, A. Sanzone, I. Facchinetti, M. Sassi and L. Beverina, Sustainable Access to π-Conjugated Molecular Materials via Direct (Hetero)Arylation Reactions in Water and under Air, Molecules, 2020, 25, 3717 CrossRef CAS PubMed;
(y) K. Mitsudo, N. Habara, Y. Kobashi, Y. Kurimoto, H. Mandai and S. Suga, Integrated Synthesis of Thienyl Thioethers and Thieno[3,2-b]Thiophenes via 1-Benzothiophen-3(2H)-Ones, Synlett, 2020, 31, 1947–1952 CrossRef CAS.
- Ö. Usluer, M. Abbas, G. Wantz, L. Vignau, L. Hirsch, E. Grana, C. Brochon, E. Cloutet and G. Hadziioannou, Metal Residues in Semiconducting Polymers: Impact on the Performance of Organic Electronic Devices, ACS Macro Lett., 2014, 3, 1134–1138 CrossRef.
-
(a) T. Kitamura, K. Morita, H. Nakamori and J. Oyamada, Synthesis of [1]Benzothieno[3,2-b][1]Benzothiophene Derivatives via Successive Iodocyclization/Photocyclization of Alkynes, J. Org. Chem., 2019, 84, 4191–4199 CrossRef CAS PubMed;
(b) K. Mitsudo, R. Matsuo, T. Yonezawa, H. Inoue, H. Mandai and S. Suga, Electrochemical Synthesis of Thienoacene Derivatives: Transition-Metal-Free Dehydrogenative C–S Coupling Promoted by a Halogen Mediator, Angew. Chem., Int. Ed., 2020, 59, 7803–7807 CrossRef CAS PubMed.
- For selected recent, additional examples of interrupted Pummerer reactions in cross-coupling, see:
(a) X. Huang and N. Maulide, Sulfoxide-Mediated α-Arylation of Carbonyl Compounds, J. Am. Chem. Soc., 2011, 133, 8510–8513 CrossRef CAS PubMed;
(b) P. Cowper, Y. Jin, M. D. Turton, G. Kociok-Köhn and S. E. Lewis, Azulenesulfonium Salts: Accessible, Stable, and Versatile Reagents for Cross-Coupling, Angew. Chem., Int. Ed., 2016, 55, 2564–2568 CrossRef CAS PubMed;
(c) B. Waldecker, F. Kraft, C. Golz and M. Alcarazo, 5-(Alkynyl)dibenzothiophenium Triflates: Sulfur-Based Reagents for Electrophilic Alkynylation, Angew. Chem., Int. Ed., 2018, 57, 12538–12542 CrossRef CAS PubMed . For our previous studies, see:;
(d) A. J. Eberhart, H. Shrives, Y. Zhang, A. Carrër, A. V. S. Parry, D. J. Tate, M. L. Turner and D. J. Procter, Sulfoxide-Directed Metal-Free Cross-Couplings in the Expedient Synthesis of Benzothiophene-Based Components of Materials, Chem. Sci., 2016, 7, 1281–1285 RSC;
(e) J. Yan, A. P. Pulis, G. J. P. Perry and D. J. Procter, Metal-Free Synthesis of Benzothiophenes by Twofold C–H Functionalization: Direct Access to Materials-Oriented Heteroaromatics, Angew. Chem., Int. Ed., 2019, 58, 15675–15679 CrossRef CAS PubMed.
-
(a) H. J. Shrives, J. A. Fernández-Salas, C. Hedtke, A. P. Pulis and D. J. Procter, Regioselective Synthesis of C3 Alkylated and Arylated Benzothiophenes, Nat. Commun., 2017, 8, 14801 CrossRef PubMed;
(b) Z. He, H. J. Shrives, J. A. Fernández-Salas, A. Abengózar, J. Neufeld, K. Yang, A. P. Pulis and D. J. Procter, Synthesis of C2 Substituted Benzothiophenes via an Interrupted Pummerer/[3,3]-Sigmatropic/1,2-Migration Cascade of Benzothiophene S-Oxides, Angew. Chem., Int. Ed., 2018, 57, 5759–5764 CrossRef CAS PubMed;
(c) K. Yang, A. P. Pulis, G. J. P. Perry and D. J. Procter, Transition-Metal-Free Synthesis of C3-Arylated Benzofurans from Benzothiophenes and Phenols, Org. Lett., 2018, 20, 7498–7503 CrossRef CAS PubMed.
-
(a) H. Kwart and E. R. Evans, The Vapor Phase Rearrangement of Thioncarbonates and Thioncarbamates, J. Org. Chem., 1966, 31, 410–413 CrossRef CAS;
(b) M. S. Newman and H. A. Karnes, The Conversion of Phenols to Thiophenols via Dialkylthiocarbamates 1, J. Org. Chem., 1966, 31, 3980–3984 CrossRef CAS;
(c) J. D. Moseley, R. F. Sankey, O. N. Tang and J. P. Gilday, The Newman–Kwart Rearrangement Re-Evaluated by Microwave Synthesis, Tetrahedron, 2006, 62, 4685–4689 CrossRef CAS;
(d) J. D. Moseley and P. Lenden, A High Temperature Investigation Using Microwave Synthesis for Electronically and Sterically Disfavoured Substrates of the Newman–Kwart Rearrangement, Tetrahedron, 2007, 63, 4120–4125 CrossRef CAS;
(e) G. C. Lloyd-Jones, J. D. Moseley and J. S. Renny, Mechanism and Application of the Newman-Kwart O→S Rearrangement of O-Aryl Thiocarbamates, Synthesis, 2008, 2008, 661–689 CrossRef;
(f) J. N. Harvey, J. Jover, G. C. Lloyd-Jones, J. D. Moseley, P. Murray and J. S. Renny, The Newman-Kwart Rearrangement of O-Aryl Thiocarbamates: Substantial Reduction in Reaction Temperatures through Palladium Catalysis, Angew. Chem., Int. Ed., 2009, 48, 7612–7615 CrossRef CAS PubMed;
(g) A. J. Perkowski, C. L. Cruz and D. A. Nicewicz, Ambient-Temperature Newman–Kwart Rearrangement Mediated by Organic Photoredox Catalysis, J. Am. Chem. Soc., 2015, 137, 15684–15687 CrossRef CAS PubMed;
(h) S. K. Pedersen, A. Ulfkjær, M. N. Newman, S. Yogarasa, A. U. Petersen, T. I. Sølling and M. Pittelkow, Inverting the Selectivity of the Newman–Kwart Rearrangement via One Electron Oxidation at Room Temperature, J. Org. Chem., 2018, 83, 12000–12006 CrossRef CAS PubMed;
(i) T. Broese, A. F. Roesel, A. Prudlik and R. Francke, An Electrocatalytic Newman–Kwart-Type Rearrangement, Org. Lett., 2018, 20, 7483–7487 CrossRef CAS PubMed;
(j) C. L. Cruz and D. A. Nicewicz, Mechanistic Investigations into the Cation Radical Newman–Kwart
Rearrangement, ACS Catal., 2019, 9, 3926–3935 CrossRef CAS.
- T. Higashino, A. Ueda and H. Mori, Di- and Tetramethoxy Benzothienobenzothiophenes: Substitution Position Effects on the Intermolecular Interactions, Crystal Packing and Transistor Properties, New J. Chem., 2019, 43, 884–892 RSC.
-
(a) A. Basu, M. R. Niazi, A. D. Scaccabarozzi, H. Faber, Z. Fei, D. H. Anjum, A. F. Paterson, O. Boltalina, M. Heeney and T. D. Anthopoulos, Impact of P-Type Doping on Charge Transport in Blade-Coated Small-Molecule:Polymer Blend Transistors, J. Mater. Chem. C, 2020, 8, 15368–15376 RSC;
(b) A. F. Paterson, A. D. Mottram, H. Faber, M. R. Niazi, Z. Fei, M. Heeney and T. D. Anthopoulos, Impact of the Gate Dielectric on Contact Resistance in High-Mobility Organic Transistors, Adv. Electron. Mater., 2019, 5, 1800723 CrossRef.
- J. Smith, W. Zhang, R. Sougrat, K. Zhao, R. Li, D. Cha, A. Amassian, M. Heeney, I. McCulloch and T. D. Anthopoulos, Solution-Processed Small Molecule-Polymer Blend Organic Thin-Film Transistors with Hole Mobility Greater than 5 cm2/Vs, Adv. Mater., 2012, 24, 2441–2446 CrossRef CAS PubMed.
Footnotes |
† Electronic supplementary information (ESI) available. See DOI: 10.1039/d1sc05070b |
‡ These authors contributed equally. |
|
This journal is © The Royal Society of Chemistry 2022 |