DOI:
10.1039/D1SC04779E
(Edge Article)
Chem. Sci., 2022,
13, 842-847
Multivariate sodalite zeolitic imidazolate frameworks: a direct solvent-free synthesis†
Received
30th August 2021
, Accepted 18th December 2021
First published on 24th December 2021
Abstract
Different mixed-ligand Zeolitic Imidazolate Frameworks (ZIFs) with sodalite topology, i.e. isoreticular to ZIF-8, unachievable by conventional synthetic routes, have been prepared using a solvent-free methodology. In particular, the versatility of this method is demonstrated with three different metal centres (Zn, Co and Fe) and binary combinations of three different ligands (2-methylimidazole, 2-ethylimidazole and 2-methylbenzimidazole). One combination of ligands, 2-ethylimidazole and 2-methylbenzimidazole, results in the formation of SOD frameworks for the three metal centres despite this topology not being obtained for the individual ligands. Theoretical calculations confirm that this topology is the lowest in energy upon ligand mixing.
Introduction
Multivariate materials composed of several distinct functional units are attracting interest due to their advanced versatility. Metal–organic frameworks (MOFs)1–3 offer a unique scenario for such materials due to their intrinsic chemical tunability.4–6 Zeolitic imidazolate frameworks (ZIFs) are a subclass of MOFs formed by tetracoordinated metal centres and imidazolate linkers, resulting in extended solids with zeolitic topologies.7,8 The most studied ZIF is arguably ZIF-8,7,8 formed by 2-methylimidazolate (mim) and Zn(II), presenting a sodalite (SOD) topology,9,10i.e. interconnected porous cages by six and four membered rings with small pore apertures. The SOD topology is not exclusive to ZIF-8, and can be obtained through direct synthesis with other metals, such as Co(II),11 Cd(II),12 Mg(II),13 Mn(II),14 and Fe(II);15 with substituted imidazole derivatives in the 2-position, such as 2-chloroimidazole,16 2-bromoimidazole,16 2-nitroimidazole,17 and 2-carbaldehydeimidazole;18 or in the 4- and 5-positions, such as benzimidazole.7,19 Very recently, this number of imidazole derivatives has been further expanded through the use of mechanochemistry.20
This versatility in both the metal and the ligand results in the modulation of properties such as the network flexibility, which has shown to be fundamental for key properties, as in catalytic applications,21,22 and indicates the possibility of fine-tuning the properties through a combination of ligands,23 as reported in other families of MOFs.24–29 However, other ligands that would normally form different structures are challenging to be incorporated into the SOD structure of ZIF-8 through a direct synthesis. In fact, the mixture of such ligands normally induces changes in the topology.30
In addition, the inclusion of a second ligand in the SOD topology is of interest to enhance the stability of the framework. In this sense, Friščić, Navrotsky and colleagues have recently suggested a thermodynamic approximation to enhance the ZIFs stability, focussing on the effects of different substituents of the linkers, contrary to the common approach of focussing on the kinetic effects.20,31,32 Thus, this idea can be extrapolated to obtain thermodynamic stable SOD ZIFs through the combination of several ligands.
Beyond the direct synthesis, alternative synthetic methodologies such as post-synthetic linker-exchange (PSLE) allow materials that are not available by common routes to be obtained.33 For example, partial exchange of the 2-methylimidazole by 5,6-dimethylbenzimidazole has been achieved through a shell–ligand-exchange-reaction (SLER),34 whereas anchoring of fluorinated molecules has been possible through thiol-ene click reaction.35 However, this approach is typically effective only at the surface. Vapour-phase ligand exchange (VPLE) has also been used to incorporate different imidazole derivatives, although it is limited to certain imidazoles, resulting in some cases in a transformation into dense phases.36 Nevertheless, a major limitation of post-synthetic strategies is the incorporation of additional synthetic steps, which hinders a scalable process and therefore a commercial use.
Herein, we report a direct green synthetic route based on a solvent-free methodology37,38 to construct mixed-ligand SOD ZIFs materials unapproachable by pure ligand synthesis. The success of this approach is demonstrated with three different combinations of ligand mixtures and three different metals (Fig. 1). Specifically, we have used different combinations of 2-methylimidazolate (mi), 2-methylbenzimidazolate (mb) and 2-ethylimidazolate (ei), thus using bulkier substituents in the 2-position or in the 4–5-positions (see Fig. 1a). As metal centres, we have explored Zn(II), Co(II) and Fe(II), totalling eight compounds as phase pure crystalline materials, denoted ZIF-8-mimb, ZIF-67-mimb, MUV-3-mimb, ZIF-8-eimb, ZIF-67-eimb, MUV-3-eimb, ZIF-8-eimi and ZIF-67-eimi, where the initial part of the name indicates the “parent” ZIF with SOD topology (i.e.ZIF-8 for Zn(II), ZIF-67 for Co(II) and MUV-3 for Fe(II)) and the second part of the name indicates the mixture of ligands used (mi = 2-methylimidazole, mb = 2-methylbenzimidazole, ei = 2-ethylimidazole).
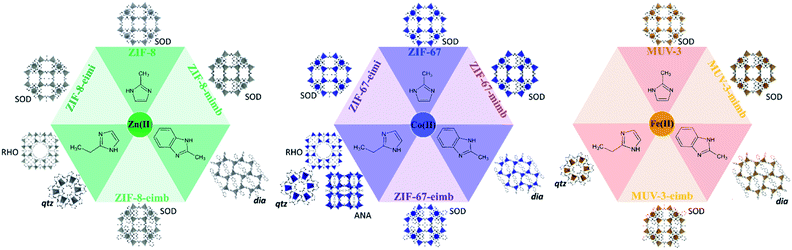 |
| Fig. 1 Scheme of the different achievable crystal structures by solvent-free synthesis combining M(II) and three imidazoles: 2-methylimidazole, 2-ethylimidazole and 2-methylbenzimidazole. The combination of two different imidazoles permits the formation of sodalite materials, in contrast to the dense coordination polymers formed with the pure ligand synthesis. | |
The pure ligand synthesis using either 2-methylimidazole or 2-ethylimidazole has been previously reported with Zn(II), Co(II) and Fe(II), yielding SOD frameworks in the case of the former (the well-known ZIF-8, ZIF-67 and MUV-3); or different polymorphs in the case of the latter, including RHO (MAF-6),8,39 ANA (MAF-5)8,39 and qtz (MAF-32 and MUV-7)15,39,40 topologies (see Fig. 1 and Section S2.1.2†). In the case of 2-methylbenzimidazole, only the Fe(II) analogue has been previously reported (MUV-6),15 although the Zn(II) and Co(II) analogues can also be prepared with this solvent-free methodology (see Section S2.1.2†).
Results and discussion
A 1
:
1 mixture of the three possible binary combination of ligands results in crystalline solids after 48 h upon reaction with ZnO, cobaltocene or ferrocene at 150 °C. The phase purity and the presence of only one polymorph was determined using X-ray powder diffraction (XRPD), confirming the homogeneity of the crystals obtained during the synthesis and discarding a possible segregation of phases, a common situation when using mixtures of ligands. In eight of the nine possible scenarios, we managed to obtain mixed-ligand structures with a SOD topology (Fig. 2b–d). Scanning electron micrographs (SEM) show the well-defined morphology of all of the mixed-ZIFs (see Fig. 3b and Section 2.7). In only one situation, when using a mixture of miH and eiH with Fe(II), a qtz framework with only one of the ligands (eiH) was obtained (see Fig. S1†). The presence of two ligands was confirmed by solid-state 13C NMR spectroscopy (Fig. 3a) in the diamagnetic samples (ZIF-8-mimb, ZIF-8-eimb and ZIF-8-eimi). In addition, all the eight mixed-ligand samples were digested in D2O and deuterated trifluoroacetic acid, and then evaluated by 1H NMR spectroscopy (see Section S2.4†). In all cases, we observed an approximate 2
:
1 ratio in the ligands, with a minor component of the bulkier imidazole derivative. Interestingly, it is possible to slightly modify the final ratio by adjusting the proportion in the synthesis. Nevertheless, we have found an upper limit of ca. 70% and a lower limit of ca. 50% for eiH and miH, respectively.
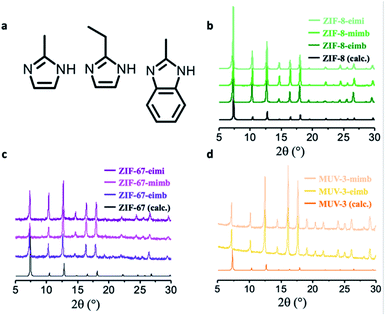 |
| Fig. 2 (a) ZIF-8, ZIF-67 and MUV-3 are constructed by 2-methylimidazole (mi), which is increased in size with bulkier ligands 2-ethylimidazole (ei) and 2-methylbenzimidazole (mb). (b–d) X-ray powder diffractograms of ZIF-mimb, ZIF-eimb and ZIF-eimi (b, c and d for the different metallic centres, Zn(II), Co(II) and Fe(II), respectively), showing the isoreticularity between them. | |
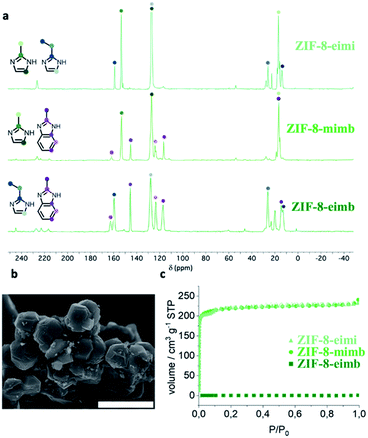 |
| Fig. 3 (a) Solid-state 13C NMR spectra of ZIF-8-eimi, ZIF-8-mimb and ZIF-8-eimb. (b) Scanning electron micrograph of typical ZIF-8 mixed-ligand compounds. (c) N2 sorption (solid symbols) and desorption (open symbols) of ZIF-8 mixed-ligand materials at 77 K. | |
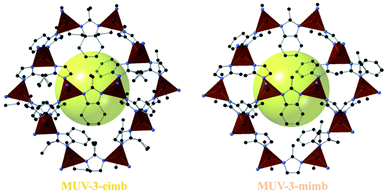 |
| Fig. 4 Crystal structures of MUV-3-eimb and MUV-3-mimb. Color key: Fe, orange tetrahedra; C, black balls; N, blue balls. Hydrogen atoms are omitted for clarity. The yellow sphere (diameter = 12 Å) is included for better visualization of the void. | |
Importantly, large crystals suitable for X-ray single crystal diffraction were obtained for two compounds, namely MUV-3-mimb and MUV-3-eimb (see Fig. 4). Structural analysis reveals that both compounds are isoreticular with MUV-3 (and ZIF-8 and ZIF-67), with similar crystal cell parameters and Fe–N distances (see Table S2†), being slightly larger than MUV-3. In addition, the angle θ between the imidazoles and the 4MR plane, as previously defined,41,42 are similar in the three structures: 74.4°, 75.9° and 77.4° for MUV-3,21MUV-3-mimb and MUV-3-eimb, respectively. Interestingly, despite starting with a 1
:
1 ratio between the two ligands, their occupancy was included in the refinement, resulting in both cases in a 70
:
30 ratio (mi
:
mb or ei
:
mb for MUV-3-mimb and MUV-3-eimb, respectively), as also found by 1H NMR spectroscopy (see Section S2.4†). Several single crystals were analysed, with similar results for each of them. The confirmed mixed-ligand Fe(II) structures present higher thermal stability (Fig. S12†) compared to MUV-315 as a result of the incorporation of mb in the structure.
The magnetic behaviour of polycrystalline bulk samples of MUV-3-mimb, MUV-3-eimb, ZIF-67-mimb, ZIF-67-eimb and ZIF-67-eimi was investigated by SQUID measurements in the range 2–300 K. Variable-temperature direct current (dc) magnetic susceptibility measurements (χ vs. T) show an increase upon cooling down until a maximum is reached (Fig. S5 and S6†), indicating the presence of strong antiferromagnetic metal–metal interactions between the M(II), as also observed in MUV-3.15 The maximum in χ is followed by a sharp decrease at lower temperature, which agrees with an antiferromagnetic ordered structure. The presence of a single maximum in the magnetic measurements suggests a single phase transition, related to the presence of only one crystalline phase (Fig. S5 and S6†), discarding the combination of two different phases or pure ligand domains. Moreover, the Neél temperatures (TN) for the mixed-ligand ZIFs (13.9–17.8 K) are close to the pure ligand SOD materials (17.2–20.6 K), in contrast to the TN found for dia and qtz topologies (TN = 32.2 and 35.9 K, respectively, see Section S2.3†).
The porous nature of these mixed-ligand materials has been analysed using N2 sorption measurements, revealing some differences depending on the combination of ligands. Thus, whereas ZIF-8-mimb and ZIF-8-eimi show BET values of 875 and 845 m2 g−1, respectively (Fig. 3c), in agreement with previous reports,43ZIF-8-eimb shows a negligible N2 sorption capacity. On the contrary, for the Co(II) compounds, only ZIF-67-eimi presents remarkable porosity, albeit lower than the Zn(II) analogue (BET of 402 m2 g−1, Fig. S21†), whereas the two mixed-ligand Fe(II) materials present a negligible N2 sorption capacity. This seems to be the result of a smaller window caused by the bulkier ligand combined with a different gate-opening capacity depending on the metal, as previously hypothesized.22,44 These textural properties are also accompanied by a higher stability towards water, improving their chemical stability and hydrophobicity (see Section S4 in the ESI†).
From the above results, the most counterintuitive outcome is that the mixture of mbH and eiH leads to a material with SOD topology in any of the three metals evaluated. As shown in Fig. 5a, qtz is the most stable topology for the exclusive use of eiH, whereas the most favourable topology for the use of only mbH is the dia topology. In fact, previous attempts to combine mbH with another ligand has resulted in the formation of JUC-16045 and Zn(2mbim)0.28(5mbim)1.72 (ref. 30) with GIS and ykh topologies, respectively. Thus, we further investigated this from a theoretical point of view. Density functional theory calculations were performed for ZIF-8, ZIF-67 and MUV-3 in several ligand compositions to assess phase stability (see Section S3 in the ESI† for computational details). Minimum-energy crystal structures for the pure- and mixed-ligand MOFs at the PBEsol level present cell parameters in good accord with the experimental data (Tables S1, S4 and S5†). Theoretical calculations predict the most stable phases for each pure-ligand ZIF to be SOD for mi, dia for mb, and qtz for ei (Table S4†), in agreement with the polymorphs found experimentally for the zeolitic framework series.46
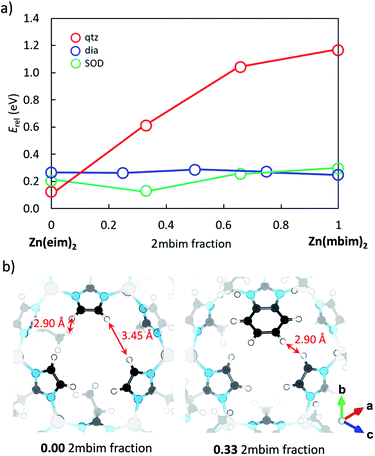 |
| Fig. 5 (a) Phase stability diagram calculated at the PBEsol level for mixed eim:mbim Zn-based ZIF-8 with respect to the most stable pure-mim ZIF-8 (the corresponding diagrams for Co and Fe are shown in Fig. S24 and S25†). Lines are drawn to guide the eye. (b) Crystal structure calculated for pure-eim ZIF-8 (left) and ZIF-8-eimb at a 2 : 1 ei : mb composition (right). Relevant H⋯H distances are indicated. | |
Fig. 5a displays the relative phase energy as a function of the ei
:
mb ligand mixing ratio in the case of ZIF-8 (see Fig. S24† for Co-based ZIF-67 and Fig. S25† for Fe-based MUV-3). Upon increasing the mb fraction, the qtz topology rapidly destabilizes up to an energy difference of >1 eV when reaching 100% mb with respect to pure-ei ZIF-8. This destabilization can be rationalized by the large steric hindrance promoted through the introduction of bulkier mb ligands with respect to ei in the relatively small qtz unit cell, which suffers a 45% cell expansion in going from pure-ei to pure-mb (Table S4†). In contrast, ligand substitution barely affects phase stability in dia topology, which is computed more stable than qtz for ei:mb ratios of >0.25 (Fig. 5a). Interestingly, the SOD topology, which is not the most stable phase either in pure-ei or pure-mb ZIFs, is predicted the lowest in energy upon ligand mixing, especially at a 2
:
1 ei
:
mb composition, in the three ZIFs (Fig. 5a, S24 and S25†). A careful inspection of the crystal structure evidences for the 33% mb fraction an effective match of the two ei and one mb ligands at the SOD six-membered ring gate (Fig. 5b), along with additional ethyl⋯benzene CH⋯π interactions between ei and mb (Fig. S28†). On the other hand, the addition of further mb leads to an increased steric hindrance between benzene rings that destabilizes the SOD topology in favour of dia, for 100% mb (Fig. 5a, S24 and S25†).
Conclusions
In summary, the solvent-free synthesis offers a platform with high versatility and applicability, which allows the preparation of multivariate ZIFs, enabling the incorporation of bulkier ligands retaining the sodalite topology. We have demonstrated the versatility of this approach with three different binary combinations of ligands and three different metal centres, resulting in eight novel ZIFs with different response towards N2 sorption. In this sense, recent studies have shown the importance of the metal centres in lowering the pressure at which the gate opening occurs in ZIF-8,21 but we demonstrate that the use of mixed-ligand frameworks is an alternative approach to modify the physical properties of ZIFs. Theoretical calculations confirm the preference of a SOD topology when using mixture of ligands, which is rationalized by invoking inter-ligand steric hindrance effects. This solvent-free approach allows to design multivariate ZIFs with network complexity, thus opening the door to also combine different metals in the same framework, and paving the way to reach similar complexity in ZIFs as that observed in other promising MOFs.5 In addition, a further advantage that presents this methodology concerns its facile applicability, involving a solvent-free approach that has proven useful for its processing.38
Data availability
All experimental and crystallographic data is available in the ESI.† Crystallographic data for MUV-3-eimb and MUV-3-mimb has been deposited at the CCDC (codes 2095300 and 2095301) and can be obtained from http://www.ccdc.cam.ac.uk/conts/retrieving.html.
Author contributions
J .L. C. synthetized the materials. E. M. C. performed the NMR analysis. E. A. G. conducted the adsorption studies. I .J. V. Y. contributed to solution and refinement of the structures from single crystal data. M. E.-R. and J. C. have performed the theoretical calculations and analyzed the computational data. G. M. E. conceived and designed the experiments. J. L. C. and G. M. E. prepared the manuscript. All authors commented on the manuscript.
Conflicts of interest
There are no conflicts to declare.
Acknowledgements
The work has been supported by the European Union (ERC-2016-CoG 724681-S-CAGE), grants PID2020-117177GB-I00, PID2020-119748GA-I00 and CEX2019-000919-M, funded by MCIN/AEI/10.13039/501100011033, and the Generalitat Valenciana (PROMETEU/2019/066 and GV/2021/027). J. L.-C. acknowledges the Universitat de València for an “Atracció de Talent” grant. E. M.-C. and E. A.-G thank MICINN for a PhD fellowship (BES-2017-082451) and a Juan de la Cierva Formación fellowship (FJC2019-039015-I), respectively. J. C. is grateful to the Generalitat Valenciana (APOSTD/2017/081).
Notes and references
- O. M. Yaghi, M. O'Keeffe, N. W. Ockwig, H. K. Chae, M. Eddaoudi and J. Kim, Nature, 2003, 423, 705–714 CrossRef CAS PubMed
.
- N. W. Ockwig, O. Delgado-Friedrichs, M. O'Keeffe and O. M. Yaghi, Acc. Chem. Res., 2005, 38, 176–182 CrossRef CAS PubMed
.
- S. Kitagawa, R. Kitaura and S. Noro, Angew. Chem., Int. Ed., 2004, 43, 2334–2375 CrossRef CAS PubMed
.
- H. Deng, C. J. Doonan, H. Furukawa, R. B. Ferreira, J. Towne, C. B. Knobler, B. Wang and O. M. Yaghi, Science, 2010, 327, 846–850 CrossRef CAS PubMed
.
- Z. Ji, T. Li and O. M. Yaghi, Science, 2020, 369, 674–680 CrossRef CAS PubMed
.
- J. Jiao, W. Gong, X. Wu, S. Yang and Y. Cui, Coord. Chem. Rev., 2019, 385, 174–190 CrossRef CAS
.
- K. S. Park, Z. Ni, A. P. Côté, J. Y. Choi, R. Huang, F. J. Uribe-Romo, H. K. Chae, M. O'Keeffe and O. M. Yaghi, Proc. Natl. Acad. Sci. U. S. A., 2006, 103, 10186–10191 CrossRef CAS PubMed
.
- X.-C. Huang, Y.-Y. Lin, J.-P. Zhang and X.-M. Chen, Angew. Chem., Int. Ed., 2006, 45, 1557–1559 CrossRef CAS PubMed
.
- Q. Qian, P. A. Asinger, M. J. Lee, G. Han, K. Mizrahi Rodriguez, S. Lin, F. M. Benedetti, A. X. Wu, W. S. Chi and Z. P. Smith, Chem. Rev., 2020, 120, 8161–8266 CrossRef CAS PubMed
.
- L. Yang, S. Qian, X. Wang, X. Cui, B. Chen and H. Xing, Chem. Soc. Rev., 2020, 5359–5406 RSC
.
- R. Banerjee, A. Phan, B. Wang, C. Knobler, H. Furukawa, M. O'Keeffe and O. M. Yaghi, Science, 2008, 319, 939–943 CrossRef CAS PubMed
.
- Y.-Q. Tian, S.-Y. Yao, D. Gu, K.-H. Cui, D.-W. Guo, G. Zhang, Z.-X. Chen and D.-Y. Zhao, Chem.–Eur. J., 2010, 16, 1137–1141 CrossRef CAS PubMed
.
- S. Horike, K. Kadota, T. Itakura, M. Inukai and S. Kitagawa, Dalton Trans., 2015, 44, 15107–15110 RSC
.
- K. Kadota, E. Sivaniah, S. Bureekaew, S. Kitagawa and S. Horike, Inorg. Chem., 2017, 52334, 8744–8747 CrossRef PubMed
.
- J. López-Cabrelles, J. Romero, G. Abellán, M. Giménez-Marqués, M. Palomino, S. Valencia, F. Rey and G. Mínguez Espallargas, J. Am. Chem. Soc., 2019, 141, 7173–7180 CrossRef PubMed
.
- K. Li, D. H. Olson, J. Seidel, T. J. Emge, H. Gong, H. Zeng and J. Li, J. Am. Chem. Soc., 2009, 131, 10368–10369 CrossRef CAS PubMed
.
- S. Diring, D. O. Wang, C. Kim, M. Kondo, Y. Chen, S. Kitagawa, K. Kamei and S. Furukawa, Nat. Commun., 2013, 4, 2684 CrossRef PubMed
.
- O. M. Yaghi, H. Furukawa, W. Morris, C. J. Doonan and R. Banerjee, J. Am. Chem. Soc., 2008, 130, 12626–12627 CrossRef PubMed
.
- X. HUANG, J. Zhang and X. Chen, Chin. Sci. Bull., 2003, 48, 1531–1534 CrossRef CAS
.
- N. Novendra, J. M. Marrett, A. D. Katsenis, H. M. Titi, M. Arhangelskis, T. Friščić and A. Navrotsky, J. Am. Chem. Soc., 2020, 142, 21720–21729 CrossRef CAS PubMed
.
- J. G. Vitillo and L. Gagliardi, Chem. Mater., 2021, 33, 4465–4473 CrossRef CAS
.
- C. M. McGuirk, T. Runčevski, J. Oktawiec, A. Turkiewicz, M. K. Taylor and J. R. Long, J. Am. Chem. Soc., 2018, 140, 15924–15933 CrossRef CAS PubMed
.
- J. A. Thompson, C. R. Blad, N. A. Brunelli, M. E. Lydon, R. P. Lively, C. W. Jones and S. Nair, Chem. Mater., 2012, 24, 1930–1936 CrossRef CAS
.
- S. Wang, N. Xhaferaj, M. Wahiduzzaman, K. Oyekan, X. Li, K. Wei, B. Zheng, A. Tissot, J. Marrot, W. Shepard, C. Martineau-Corcos, Y. Filinchuk, K. Tan, G. Maurin and C. Serre, J. Am. Chem. Soc., 2019, 141, 17207–17216 CrossRef CAS PubMed
.
- W. J. Newsome, S. Ayad, J. Cordova, E. W. Reinheimer, A. D. Campiglia, J. K. Harper, K. Hanson and F. J. Uribe-Romo, J. Am. Chem. Soc., 2019, 141, 11298–11303 CrossRef CAS PubMed
.
- B. Lerma-Berlanga, C. R. Ganivet, N. Almora-Barrios, S. Tatay, Y. Peng, J. Albero, O. Fabelo, J. González-Platas, H. García, N. M. Padial and C. Martí-Gastaldo, J. Am. Chem. Soc., 2021, 143, 1798–1806 CrossRef CAS PubMed
.
- C.-C. Cao, C.-X. Chen, Z.-W. Wei, Q.-F. Qiu, N.-X. Zhu, Y.-Y. Xiong, J.-J. Jiang, D. Wang and C.-Y. Su, J. Am. Chem. Soc., 2019, 141, 2589–2593 CrossRef CAS PubMed
.
- T. Y. Luo, C. Liu, X. Y. Gan, P. F. Muldoon, N. A. Diemler, J. E. Millstone and N. L. Rosi, J. Am. Chem. Soc., 2019, 141, 2161–2168 CrossRef CAS PubMed
.
- Z. M. Schulte, Y. H. Kwon, Y. Han, C. Liu, L. Li, Y. Yang, A. G. Jarvi, S. Saxena, G. Veser, J. K. Johnson and N. L. Rosi, Chem. Sci., 2020, 11, 12807–12815 RSC
.
- J. Yang, Y. B. Zhang, Q. Liu, C. A. Trickett, E. Gutiérrez-Puebla, M. Á. Monge, H. Cong, A. Aldossary, H. Deng and O. M. Yaghi, J. Am. Chem. Soc., 2017, 139, 6448–6455 CrossRef CAS PubMed
.
- Z. Akimbekov, A. D. Katsenis, G. P. Nagabhushana, G. Ayoub, M. Arhangelskis, A. J. Morris, T. Friščić and A. Navrotsky, J. Am. Chem. Soc., 2017, 139, 7952–7957 CrossRef CAS PubMed
.
- M. Arhangelskis, A. D. Katsenis, N. Novendra, Z. Akimbekov, D. Gandrath, J. M. Marrett, G. Ayoub, A. J. Morris, O. K. Farha, T. Friščić and A. Navrotsky, Chem. Mater., 2019, 31, 3777–3783 CrossRef CAS
.
- O. Karagiaridi, M. B. Lalonde, W. Bury, A. a. Sarjeant, O. K. Farha and J. T. Hupp, J. Am. Chem. Soc., 2012, 134, 18790–18796 CrossRef CAS PubMed
.
- X. Liu, Y. Li, Y. Ban, Y. Peng, H. Jin, H. Bux, L. Xu, J. Caro and W. Yang, Chem. Commun., 2013, 49, 9140–9142 RSC
.
- Q. Sun, H. He, W. Y. Gao, B. Aguila, L. Wojtas, Z. Dai, J. Li, Y. S. Chen, F. S. Xiao and S. Ma, Nat. Commun., 2016, 7, 1–7 Search PubMed
.
- J. Marreiros, L. Van Dommelen, G. Fleury, R. Oliveira-Silva, T. Stassin, P. Iacomi, S. Furukawa, D. Sakellariou, P. L. Llewellyn, M. Roeffaers and R. Ameloot, Angew. Chem., 2019, 131, 18642–18646 CrossRef
.
- J. López-Cabrelles, S. Mañas-Valero, I. J. Vitórica-Yrezábal, P. J. Bereciartua, J. A. Rodríguez-Velamazán, J. C. Waerenborgh, B. J. C. Vieira, D. Davidovikj, P. G. Steeneken, H. S. J. van der Zant, G. Mínguez Espallargas and E. Coronado, Nat. Chem., 2018, 10, 1001–1007 CrossRef PubMed
.
- I. Stassen, M. Styles, G. Grenci, H. Van Gorp, W. Vanderlinden, S. De Feyter, P. Falcaro, D. De Vos, P. Vereecken and R. Ameloot, Nat. Mater., 2016, 15, 304–310 CrossRef CAS PubMed
.
- B. N. Bhadra, P. W. Seo, N. A. Khan and S. H. Jhung, Inorg. Chem., 2016, 55, 11362–11371 CrossRef CAS PubMed
.
- P. J. Beldon, L. Fábián, R. S. Stein, A. Thirumurugan, A. K. Cheetham and T. Friščić, Angew. Chem., Int. Ed., 2010, 49, 9640–9643 CrossRef CAS PubMed
.
- C. L. Hobday, T. D. Bennett, D. Fairen-Jimenez, A. J. Graham, C. A. Morrison, D. R. Allan, T. Düren and S. A. Moggach, J. Am. Chem. Soc., 2018, 140, 382–387 CrossRef CAS PubMed
.
- C. L. Hobday, C. H. Woodall, M. J. Lennox, M. Frost, K. Kamenev, T. Düren, C. A. Morrison and S. A. Moggach, Nat. Commun., 2018, 9, 1–9 CrossRef CAS PubMed
.
- H. M. Titi, J. L. Do, A. J. Howarth, K. Nagapudi and T. Friščić, Chem. Sci., 2020, 11, 7578–7584 RSC
.
- E. Andres-Garcia, J. López-Cabrelles, L. Oar-Arteta, B. Roldan-Martinez, M. Cano-Padilla, J. Gascon, G. Mínguez Espallargas and F. Kapteijn, Chem. Eng. J., 2019, 371, 848–856 CrossRef CAS
.
- L. Huang, M. Xue, Q. Song, S. Chen, Y. Pan and S. Qiu, Inorg. Chem. Commun., 2014, 46, 9–12 CrossRef CAS
.
- Note that RHO and ANA topologies are not considered as (i) they are only detected experimentally for pure-eim ZIFs of Zn(II) and Co(II), (ii) they are found higher in energy compared to qtz polymorph (see ref. 31), and (iii) they are highly computationally demanding..
Footnote |
† Electronic supplementary information (ESI) available. CCDC 20953002095301. For ESI and crystallographic data in CIF or other electronic format see DOI: 10.1039/d1sc04779e |
|
This journal is © The Royal Society of Chemistry 2022 |