DOI:
10.1039/D2RA07386B
(Paper)
RSC Adv., 2022,
12, 34790-34796
External environment sensitive circularly polarized luminescence properties of a chiral boron difluoride complex†
Received
21st November 2022
, Accepted 28th November 2022
First published on 6th December 2022
Abstract
A chiral Schiff-base boron difluoride complex bearing a diethylamino group was synthesized. Its photophysical properties were investigated and compared with those of its non-substituted analogue. The complex was found to exhibit solvatofluorochromism with bluish-white emission in moderately polar solvents and intense blue emission in nonpolar solvent. Circularly polarized luminescence (CPL) properties were also examined and it was found that the absolute value of the luminescence dissymmetry factor (glum) increases significantly in the KBr-dispersed pellet state compared to the solution state. Notably, CPL intensity of the complex enhanced approximately three times upon addition of CH3SO3H in CH2Cl2. Density functional theory (DFT) calculations were conducted to further understand the photophysical properties.
Introductions
Circularly polarized luminescence (CPL)1–4 which is defined as differential emission of left- versus right-circularly polarized light have attracted increasing attentions over the past decade as an important phenomenon with potential applications in 3D optical displays,5 biological probes,6 asymmetric synthesis,7 as well as CPL lasers.8 Among the various CPL-active materials, small organic molecules (SOMs)9 have drawn growing interest owing to the potential application for circularly polarized organic light-emitting diodes (CP-OLEDs).10 Numerous examples of CPL-SOMs with chiral frameworks such as helicenes,11 cyclophanes12 and binaphthyls13 have been developed and several studies have been reported to establish guidelines for designing molecules that exhibit high CPL efficiency.11d–g,12g,13f Controlling CPL characteristics of CPL-SOMs is an important subject in the development of advanced information technologies.14 One of the strategy to achieve CPL control of SOMs is to design molecules that are sensitive to conformational changes in response to the external environment. To date, a variety of SOMs have been developed in which CPL properties can be controlled depending on the external environment, such as solvent,15 dispersed matrix16 and pH.17
Organoboron complexes have received increasing attention in recent years due to their efficient and tunable luminescent properties.18 Such characteristics have led to their wide utilization in optical and optoelectronic devices, including organic light-emitting diodes (OLEDs).19 Boron difluoride complexes, one of the families of organoboron complexes, have been recognized as promising materials for CPL-SOMs due to their ease of preparation and modification.20 Various CPL-active boron difluoride complexes21 containing conjugated π-systems including axial chirality,21a,c,i helical chirality21b,d,h and planar chirality21e–g have been reported.
As part of our program aimed at the creation of novel functional materials with CPL-activities, we have developed organic and organometallic complexes bearing chiral Schiff-base ligands.16e,21j,22 Previously, we reported that boron difluoride complexes with chiral Schiff-base ligands exhibit multi-colour CPL properties in dilute solution and in the drop cast film state.21j In the present work, we aimed to develop novel boron difluoride complexes with controllable CPL properties depending on the external environment for further applications. To this purpose, complex 1a bearing a diethylamino group was newly designed and the photophysical properties of the complex were compared with the non-substituted analogue 1b (Fig. 1). As a result, it was found that intensity and colour of CPL can be controlled by varying solvent polarity, dispersed matrix and pH of the solution. Theoretical calculations revealed a relationship between their structures and the photophysical properties including CPL-activities. Herein we describe the synthesis, structure and photophysical properties of chiral Schiff-base boron difluoride complexes with a focus on its tunable CPL properties.
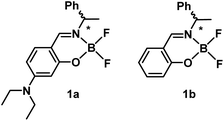 |
| Fig. 1 Structures of the boron difluoride complexes studied in this work. | |
Results and discussion
Synthesis and structures
The chiral boron difluoride complexes (R)-1a and (S)-1a were successfully synthesized by the reaction of BF3·OEt2 with optically pure Schiff-base ligands (R)-2a and (S)-2a bearing a diethylamino group in dry 1,2-dichloroethane (DCE) according to the reported procedure (Scheme 1).20d The non-substituted analogues (R)-1b and (S)-1b were also prepared as reference compounds from the corresponding optically pure Schiff-base ligands (R)-2b and (S)-2b by the same synthetic methods. The newly synthesized compounds 1a and 2a were successfully characterized by 1H and 13C nuclear magnetic resonance (NMR) spectroscopy (Fig. S1 and S3, ESI†), infra-red (IR) spectroscopy, high-resolution mass spectrometry (HRMS) and elemental analysis, respectively.
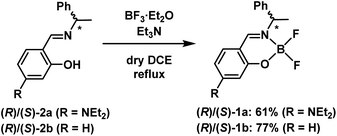 |
| Scheme 1 Synthesis of chiral boron difluoride complexes (R)/(S)-1a and 1b. | |
Single crystals of (S)-1a were obtained by recrystallization from a CH2Cl2/EtOH solution and the molecular structure was unequivocally established by X-ray diffraction (XRD) analysis at 113 K. The details of the crystal data and the structure refinement are presented in Table S1 (ESI†). ORTEP23 drawings of (S)-1a are presented in Fig. 2. The boron atoms in (S)-1a adopt a typical tetrahedral geometry to form a six-membered ring which is similar to the previously reported boron difluoride complexes.20 The packing structure and major interactions in the lattice are shown in Fig. S5 (ESI†). (S)-1a crystallizes in the chiral monoclinic space group P21. In the lattice, complex (S)-1a was fixed three-dimensional intermolecular H–F bonds.
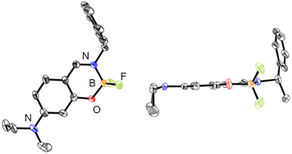 |
| Fig. 2 ORTEP representations of (S)-1a. Left figures: overhead views. Right figures: side views. | |
Photophysical properties
Circular dichroism (CD) and UV-vis absorption spectra of (R)-1a and (S)-1a were recorded in CH2Cl2 solution at room temperature (Fig. 3a and b). The spectra of complexes (R)-1b and (S)-1b under the same conditions are also shown for comparison.20d (R)-1a and (S)-1a showed mirror image CD spectra with their maxima matching the maxima of the UV-vis absorption spectra. The low energy band of 1a was increased and bathochromically shifted compared to that of 1b in both CD and UV-vis absorption spectra. This is attributed to the participation of n–π* transition character from the lone pair of the nitrogen atom of the diethylamino group. The |gabs| (=Δε/ε) values around the absorption maxima in the low energy region are calculated to be 1.4 × 10−4 (380 nm) for 1a and 1.0 × 10−3 (348 nm) for 1b, respectively. These results indicate that the participation of n–π* increases ε value, resulting in a decrease in the |gabs| value. Further consideration will be discussed in a later section with the results of the theoretical calculations.
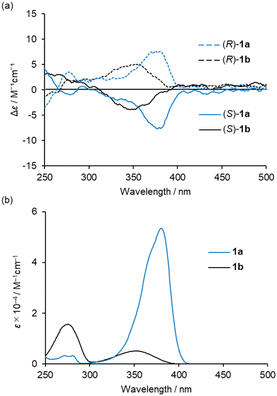 |
| Fig. 3 (a) CD and (b) UV-vis spectra of 2.0 × 10−4 M solutions of 1a and 1b in CH2Cl2 at 298 K. | |
Complex 1a exhibited blue to bluish-white luminescence with moderate emission quantum efficiencies (Φ) under UV excitation at room temperature in dilute CH2Cl2 solution, in the crystalline and in the KBr-dispersed pellet state, respectively (Fig. 4a). The photophysical data for complexes 1a and 1b are presented in Table 1. The emission spectra in each state are shown in Fig. 4b. In the solution state, complex 1a exhibited two emission bands around 420 and 540 nm, while 1b showed only one emission band around 430 nm under the same condition. The emission band around 540 nm in complex 1a is attributed to the para-quinoidal resonance structure in the excited state (Scheme 2).24 The first emission band in 1a showed a clear bathochromic shift in the solid state (λmax = 472 nm for crystal and λmax = 463 nm for crystal) compared to that of in solution state (λmax = 420 nm). The CIE colour coordinates plotted on the CIE1931 chromaticity chart25 (Fig. 4b, inset) indicate that the emission colour of 1a and 1b varies between the blue to white region in each state.
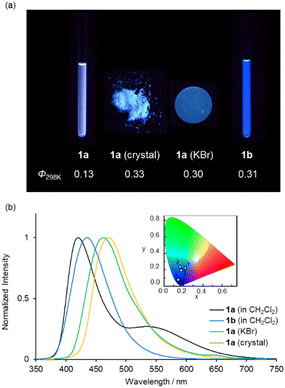 |
| Fig. 4 (a) Photographs under UV illumination at 365 nm and (b) normalized emission spectra of 1a and 1b in CH2Cl2 (2.0 × 10−4 M), crystalline and in the KBr-dispersed pellet state at 298 K (λex = 350 nm). The insets in (b) show the CIE colour coordinates of the emissions (1, 1a in CH2Cl2; 2, 1b in CH2Cl2; 3, 1a (KBr); 4, 1a (crystal)). | |
Table 1 Photophysical data for complexes 1a and 1ba
Compound |
Medium |
λabs [nm] |
λmaxb [nm] |
Φb,c |
|glum|d |
CIE (x, y)b |
Data were obtained from a 2.0 × 10−4 M solution, crystals or KBr-dispersed pellets at 298 K. λex = 350 nm. Luminescent quantum efficiencies measured using the absolute method with an integrating sphere. The |glum| values around emission peak maxima are listed. The data for 1b (reported in ref. 20d) are provided for comparison. |
1a |
CH2Cl2 |
281, 381 |
420, 539 |
0.13 |
2.8 × 10−4 (426 nm) |
0.23, 0.21 |
CH2Cl2 + CH3SO3H (10 eq.) |
267, 346 |
423 |
0.12 |
9.0 × 10−4 (426 nm) |
0.17, 0.09 |
Toluene |
279, 379 |
414 |
0.59 |
2.3 × 10−4 (423 nm) |
0.17, 0.07 |
CHCl3 |
282, 381 |
417, 534 |
0.49 |
2.6 × 10−4 (418 nm) |
0.18, 0.12 |
DCE |
281, 380 |
421, 546 |
0.07 |
2.0 × 10−4 (423 nm) |
0.25, 0.22 |
THF |
279, 375 |
417, 550 |
0.04 |
2.4 × 10−4 (424 nm) |
0.22, 0.19 |
Crystal |
— |
472 |
0.33 |
— |
0.16, 0.23 |
KBr pellet |
— |
463 |
0.30 |
2.4 × 10−3 (461 nm) |
0.17, 0.20 |
1b |
CH2Cl2 |
354e |
433e |
0.32e |
1.0 × 10−3 (433 nm) |
0.15, 0.08 |
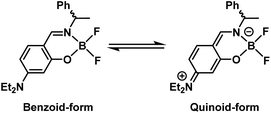 |
| Scheme 2 Plausible equilibrium of the resonance structures of complex 1a in the excited state. | |
While it has been reported that 1b exhibits identical fluorescent properties in any solvent,20d 1a exhibits solvatofluorochromism depending on the polarity of the solvents. Fig. 5a shows photographs of 1a in various organic solvents under UV irradiation. Bluish-white emissions were observed in moderately polar solvents such as CHCl3, DCE and THF as well as CH2Cl2. In nonpolar solvents like toluene, 1a showed intense blue emission with a high Φ value (0.59) (Fig. 5a). In highly polar solvents (CH3CN, acetone, DMF and MeOH), 1a exhibited weak blue emission with a low Φ value (0.01) (Fig. S6 and Table S2, ESI†). The UV-vis absorption spectra of 1a in various organic solvents are shown in Fig. S7 (ESI†), where identical absorption spectra were observed in all solutions. From these results, we conclude that the polarity of the solvents affects the stability of the resonance structures (benzoid- and quinoid-form) in the excited state which is the key to the dual emission properties of 1a.
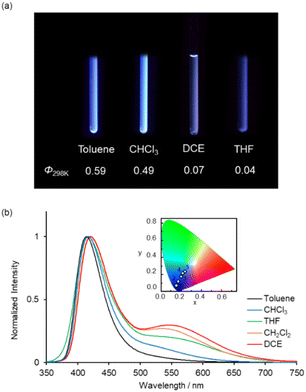 |
| Fig. 5 (a) Photographs under UV illumination at 365 nm and (b) normalized emission spectra of 2.0 × 10−4 M solutions of (S)-1a in various organic solvents at 298 K (λex = 350 nm). The insets in (b) show the CIE colour coordinates of the emissions (solvents: 1, toluene; 2, CHCl3; 3, THF; 4, CH2Cl2; 5, DCE). | |
The CPL spectra of the enantiomeric samples 1a and 1b in dilute organic solutions show mirror image spectra (Fig. 6 and S8, ESI†), and the emission peak maxima of the CPL signals correspond well to the emission spectra taken under the same measurement conditions (Fig. 4b and 5b). In general, the efficiency of CPL is usually quantified by means of the luminescence dissymmetry factor (glum = 2ΔI/I = 2(IL − IR)/(IL + IR), in which IL and IR are the intensity of left- and right-circularly polarized luminescence).26 The |glum| values around the maximum emission wavelength in CH2Cl2 solution are calculated to be 2.8 × 10−4 (426 nm) for 1a and 1.0 × 10−3 (433 nm) for 1b, respectively, which are typical values for small organic and organometallic molecules.27 The CPL spectra of (R)-1a and (S)-1a in toluene, CHCl3, DCE and THF also showed glum values of the 10−4 order (Table 1 and Fig. S8, ESI†). The decrease in the |glum| value caused by the introduction of a diethylamino group corresponds to the decrease in the |gabs| value calculated from the CD spectra, which will be also discussed with the results of theoretical calculations described below. The CPL spectra of (R)-1a and (S)-1a were also recorded in the KBr-dispersed pellet state, showing clear mirror image signals. Their maximum emission |glum| values are calculated to be 2.4 × 10−3 (461 nm), which is approximately 9 times higher than the value measured in solution (Fig. 7). This improvement of CPL chirality in the KBr-dispersed pellet is considered to be due to the emergence of supramolecular chirality in the aggregated state.
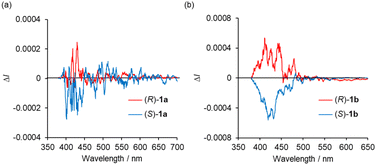 |
| Fig. 6 CPL spectra of (R)-and (S)- (a) 1a and (b) 1b in CH2Cl2 (2.0 × 10−4 M) at 298 K (λex = 350 nm). | |
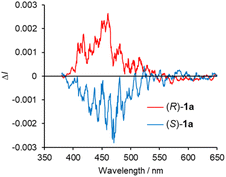 |
| Fig. 7 CPL spectra of (R)-and (S)-1a in the KBr-dispersed pellet state at 298 K (λex = 350 nm). | |
One of the most important photophysical properties of complex 1a is the acid-induced CPL enhancement, observed in CH2Cl2 upon addition of an excess CH3SO3H. As shown in Fig. 8a, the addition of excess amounts of CH3SO3H (10 equiv.) to a bluish-white emissive solution of complex 1a in CH2Cl2 typically causes the solution to exhibit blue fluorescence at 298 K. 1H NMR spectrum of complex 1a with CH3SO3H in CDCl3 suggests that 1a is stable under low concentrated acidic solution (Fig. S10†). Fig. 8b and c show changes in the CPL and total emission spectra of 2.0 × 10−4 M solutions of 1a in CH2Cl2. The addition of CH3SO3H resulted in an increase in CPL intensity around 420 nm (Fig. 8b). In the total emission spectrum, the emission band around 540 nm disappeared (Fig. 8c). The maximum emission |glum| values in the CPL spectra were calculated to be 9.0 × 10−4 (426 nm), which is approximately 4 times higher than the value measured in the original solution. The UV-vis spectra of 1a in CH2Cl2 with varying equivalents of CH3SO3H are shown in Fig. S9 (ESI†), where the decrease of n–π* transition band around 380 nm was observed. Hence, the increase in CPL upon acid addition can be attributed to the decrease of n–π* character in the luminescence process.
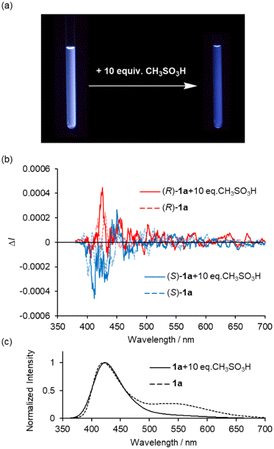 |
| Fig. 8 (a) Photographs under UV illumination at 365 nm, (b) CPL and (c) normalized total emission spectra of (R)- and (S)-1a in CH2Cl2 (2.0 × 10−4 M) with and without CH3SO3H at 298 K (λex = 350 nm). | |
Theoretical calculations
To get more insight into the photophysical properties of the present chiral boron complexes, we performed density functional theory (DFT) and time-dependent (TD) DFT calculations on the B3LYP/6-31+G(d,p) level, using the Gaussian 16 program. The optimized structures and frontier orbitals of (S)-1a and (S)-1b in the S0 (ground state) and S1 (excited state) states were estimated using DFT calculations on the basis of the X-ray structures (Fig. 9). The HOMOs are principally π orbitals of the ligand, including the non-bonding orbital of the diethylamino group of (S)-1a, whereas the LUMOs are in the ligand (π*). The energy levels and electronic configurations of the singlet states of these complexes were estimated from TD-DFT calculations (B3LYP/6-31+G(d,p)) (Tables S3 and S4, ESI†). The major contribution of the electronic configuration of the S1 states is the HOMO-to-LUMO transition, which implies that the present fluorescence is principally attributable to a mixture of n–π* and π–π* transitions of (S)-1a and the π–π* transition of (S)-1b. The S1-to-S0 transition energies for S1 states were calculated to be 2.98 eV (416 nm) for (S)-1a and 2.96 eV (419 nm) for (S)-1b, which is consistent with the emission peak maxima of the first emission band of the experimental spectra (λmax = 420 nm for (S)-1a and λmax = 433 nm for (S)-1b).
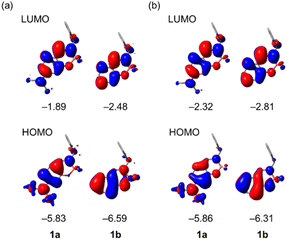 |
| Fig. 9 Molecular orbitals (overhead views) and eigenvalues [eV] for the frontier orbitals of (S)-1a and (S)-1b estimated from DFT calculations (B3LYP/6-31+G(d,p)) on the basis of the optimized geometries in the (a) S0 ground and (b) S1 excited states. Hydrogen atoms are omitted for clarity. | |
We considered the variation of chiroptical properties of (S)-1a and (S)-1b from the viewpoint of transition dipole moments using TD-DFT calculations. The dissymmetry factors gabs for CD and glum for CPL are calculated with the following equation g = 4(|μe||μm|cos
θe,m)/(|μe|2 + |μm|2), where |μe|, |μm| and θe,m are the electric transition dipole moments, magnetic transition dipole moments and the angles between the two vectors μe and μm, respectively.28 In the case of CPL-SOMs, |μm| is basically much smaller compared with |μe| and can be neglected. Thus, the equation of g can be replaced as follows: g = 4(|μm|cos
θe,m)/|μe|, in which the g value is directly proportional to |μm| and inversely proportional to |μe|. Fig. 10 shows the electric and magnetic dipole moments calculated for the upward S0-to-S1 and the downward S1-to-S0 transitions of (S)-1a and (S)-1b in the optimized geometries. For the upward S0-to-S1 transition, gabs values were calculated to be −2.8 × 10−4 for (S)-1a and −1.0 × 10−3 for (S)-1b (Fig. 10a), which is consistent with the results from experimental CD spectra (−1.4 × 10−4 for (S)-1a and −1.0 × 10−3 for (S)-1b, Table 1). The scalar values |μe| of (S)-1a is more than 2 times higher than that of (S)-1b, whereas |μm| was less than half compared to that of (S)-1b. Following the equation of the g value, the decrease of the gabs value for (S)-1a compared to that of (S)-1b is attributed to the changes in the scalar values |μe| and |μm|. For the downward S1-to-S0 transition, glum values were calculated to be −2.8 × 10−4 for (S)-1a and −1.1 × 10−3 for (S)-1b (Fig. 10b) which is consistent with the result from experimental CPL spectra (−2.8 × 10−4 for (S)-1a and −1.0 × 10−3 for (S)-1b, Table 1). The small glum for (S)-1a can be traced back to the nearly orthogonal electric and magnetic dipole moments: θe,m = 91°. The angle θe,m for the downward transition of (S)-1b is θe,m = 94°. This apparently small change in θe,m alone would influence the glum by a factor of 4 (as cos
94°/cos
91° = 0.07/0.0017). Given the calculation results, we can be certain that the orientation of dipole moments in the downward S1-to-S0 transitions is a key for the decrease in glum for (S)-1a compare to that of (S)-1b. The enhancement of the CPL intensity with the addition of CH3SO3H was also attributed to the changes in orientation of dipole moments, as the protonation of the diethylamino group reduces the contribution of the n–π* transition character.
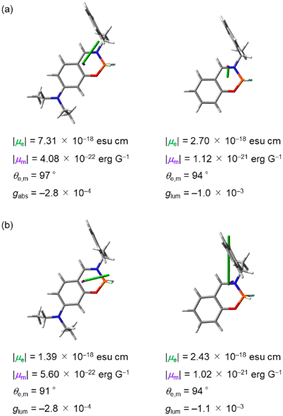 |
| Fig. 10 Electric (μe, orange) and magnetic (μm, purple) dipole moments of the (a) S0 → S1 transition and (b) S1 → S0 transition for (S)-1a (left) and (S)-1b (right) calculated at the B3LYP/6-31+G(d,p) level. Calculated values of transition dipole moments (|μe|, |μm| and θe,m) and glum are given under each structure. | |
Conclusions
In summary, we have demonstrated external environment sensitive circularly polarized luminescence based on Schiff-base difluoride boron complexes. These chiral compounds exhibited solvatofluorochromism and acid-induced CPL enhancement in the solution state. The CPL intensity was also enhanced in the KBr-dispersed pellet state with a glum value of 2.4 × 10−3. DFT and TD-DFT calculations of the structures and electronic configurations of (S)-1a and (S)-1b revealed a relationship between molecular structure and photophysical properties. Theoretical consideration of the effect of solvent polarity for solvatofluorochromic behavior observed in 1a is now in progress.
Author contributions
The project was conceived by Masahiro Ikeshita, who also directed all experiment work, theoretical calculation and wrote the manuscript. Hongxi He performed experimental works except for CPL measurements. Maho Kitahara measured CPL spectra. Yoshitane Imai and Takashi Tsuno gave constructive guidance for this study.
Conflicts of interest
There are no conflicts to declare.
Acknowledgements
This work was supported by JSPS KAKENHI (Grant Numbers JP21K20541 (M. I.), JP21K18940 (Y. I.), and JP21K05234 (T. T.)), JST, CREST (Grant Number JPMJCR2001 (Y. I.)), JGC-S Scholarship Foundation (M. I.), and Nihon University Industrial Technology Fund for Supporting Young Scholars (M. I.). We gratefully acknowledge Prof. Dr Henri Brunner (Universität Regensburg) for helpful discussion and comments. We also acknowledge Prof. Dr Takayoshi Fujii (Nihon University) for measurements of emission properties, and Prof. Dr Takeshi Naota (Osaka University) and Assoc. Prof. Dr Shuichi Suzuki (Osaka University) for measurements of HRMS spectrometry.
Notes and references
- J. P. Riehl and F. S. Richardson, Chem. Rev., 1986, 86, 1–16 CrossRef CAS.
- F. Zinna and L. Di Bari, Chirality, 2015, 27, 1–13 CrossRef CAS PubMed.
- J. P. Riehl and G. Muller, in Comprehensive Chiroptical Spectroscopy: Instrumentation, Methodologies, and Theoretical Simulations, John Wiley & Sons, Inc., 2012, vol. 1, pp. 65–90 Search PubMed.
- T. Mori, Circularly Polarized Luminescence of Isolated Small Organic Molecules, Springer, 2020 Search PubMed.
- D.-Y. Kim, J. Korean Phys. Soc., 2006, 49, S505–S508 Search PubMed.
- M. C. Heffern, L. M. Matosziuk and T. J. Meade, Chem. Rev., 2014, 114, 4496–4539 CrossRef CAS.
- Y. Inoue, Chem. Rev., 1992, 92, 741–770 CrossRef CAS.
-
(a) J. Jiménez, L. Cerdán, F. Moreno, B. L. Maroto, I. García-Moreno, J. L. Lunkley, G. Muller and S. de la Moya, J. Phys. Chem. C, 2017, 121, 5287–5292 CrossRef;
(b) D. Qu, M. Archimi, A. Camposeo, D. Pisignao and E. Zussman, ACS Nano, 2021, 15, 8753–8760 CrossRef CAS PubMed.
-
(a) E. M. Sánchez-Carnerero, A. R. Agarrabeitia, F. Moreno, B. L. Maroto, G. Muller, M. J. Ortiz and S. de La Moya, Chem.–Eur. J., 2015, 21, 13488–13500 CrossRef;
(b) N. Chen and B. Yan, Molecules, 2018, 23, 3376 CrossRef PubMed.
-
(a) J. R. Brandt, F. Salerno and M. J. Fuchter, Nat. Rev. Chem., 2017, 1, 0045 CrossRef CAS;
(b) M. Li, S.-H. Li, D. Zhang, M. Cai, L. Duan, M.-K. Fung and C.-F. Chen, Angew. Chem., Int. Ed., 2018, 57, 2889–2893 CrossRef CAS;
(c) P. Xue, X. Wang, W. Wang, J. Zhang, Z. Wang, J. Jin, C. Zheng, P. Li, G. Xie and R. Chen, ACS Appl. Mater. Interfaces, 2021, 13, 47826–47834 CrossRef CAS PubMed.
- Helicenes:
(a) J. E. Field, G. Muller, J. P. Riehl and D. Venkataraman, J. Am. Chem. Soc., 2003, 125, 11808–11809 CrossRef CAS PubMed;
(b) T. Kaseyama, S. Furumi, X. Zhang and K. Tanaka, Angew. Chem., Int. Ed., 2011, 50, 3684–3687 CrossRef CAS;
(c) K. Nakamura, S. Furumi, M. Takeuchi, T. Shibuya and K. Tanaka, J. Am. Chem. Soc., 2014, 136, 5555–5558 CrossRef CAS;
(d) H. Tanaka, M. Ikenosako, Y. Kato, M. Fujiki, Y. Inoue and T. Mori, Commun. Chem., 2018, 1, 38 Search PubMed;
(e) H. Kubo, D. Shimizu, T. Hirose and K. Matsuda, Org. Lett., 2020, 22, 9276–9281 CrossRef CAS;
(f) H. Kubo and T. Hirose, Chem. Lett., 2021, 50, 804–807 Search PubMed;
(g) H. Kubo, T. Hirose, T. Nakashima, T. Kawai, J. Hasegawa and K. Matsuda, J. Phys. Chem. Lett., 2021, 12, 686–695 CrossRef CAS PubMed;
(h) T. Mori, Chem. Rev., 2021, 121, 2373–2412 Search PubMed.
- Cyclophanes:
(a) Y. Morisaki, M. Gon, T. Sasamori, N. Tokitoh and Y. Chujo, J. Am. Chem. Soc., 2014, 136, 3350–3353 CrossRef CAS;
(b) M. Gon, Y. Morisaki and Y. Chujo, J. Mater. Chem. C, 2015, 3, 521–529 Search PubMed;
(c) S. P. Morcillo, D. Miguel, L. Alvarez de Cienfuegos, J. Justicia, S. Abbate, E. Castiglioni, C. Bour, M. Ribagorda, D. J. Cardenas, J. M. Paredes, L. Crovetto, D. Choquesillo-Lazarte, A. J. Mota, M. C. Carreno, G. Longhi and J. M. Cuerva, Chem. Sci., 2016, 7, 5663–5670 RSC;
(d) S. Sato, A. Yoshii, S. Takahashi, S. Furumi, M. Takeuchi and H. Isobe, Proc. Natl. Acad. Sci. U. S. A., 2017, 114, 13097–13101 CrossRef CAS;
(e) P. Renie, A. G. Campaña, L. A. de Cienfuegos, V. Blanco, S. Abbate, A. J. Mota, G. Longhi, D. Miguel and J. M. Cuerva, Chem. Commun., 2019, 55, 10685–10688 RSC;
(f) Y. Morisaki and Y. Chujo, Bull. Chem. Soc. Jpn., 2019, 92, 265–274 Search PubMed;
(g) N. Miki, R. Inoue and Y. Morisaki, Bull. Chem. Soc. Jpn., 2022, 95, 110–115 Search PubMed.
-
(a) T. Kawai, K. Kawamura, H. Tsumatori, M. Ishikawa, M. Naito, M. Fujiki and T. Nakashima, ChemPhysChem, 2007, 8, 1465–1468 Search PubMed;
(b) T. Kimoto, N. Tajima, M. Fujiki and Y. Imai, Chem.–Asian J., 2012, 7, 2836–2841 Search PubMed;
(c) K. Takaishi, R. Takehara and T. Ema, Chem. Commun., 2018, 54, 1449–1452 Search PubMed;
(d) K. Takaishi, K. Iwachido, R. Takehana, M. Uchiyama and T. Ema, J. Am. Chem. Soc., 2019, 141, 6185–6190 CrossRef CAS PubMed;
(e) Y. Nojima, M. Hasegawa, N. Hara, Y. Imai and Y. Mazaki, Chem. Commun., 2019, 55, 2749–2752 Search PubMed;
(f) Y. Nojima, M. Hasegawa, N. Hara, Y. Imai and Y. Mazaki, Chem.–Eur. J., 2021, 27, 5923–5929 Search PubMed.
-
(a) J. Kumar, T. Nakashima and T. Kawai, J. Phys. Chem. Lett., 2015, 6, 3445–3452 CrossRef CAS;
(b) J.-L. Ma, Q. Peng and C.-H. Zhao, Chem.–Eur. J., 2019, 25, 15441–15454 CrossRef CAS;
(c) F. Song, Z. Zhao, Z. Liu, J. W. Y. Lam and B. Z. Tang, J. Mater. Chem. C, 2020, 8, 3284–3301 Search PubMed;
(d) Y. Gao, C. Ren, X. Lin and T. He, Front. Chem., 2020, 8, 458 Search PubMed;
(e) Y. Imai, Chem. Lett., 2021, 50, 1131–1141 CrossRef CAS.
-
(a) A. Statrijio, S. C. J. Meskers and T. M. Swager, J. Am. Chem. Soc., 2006, 128, 9030–9031 CrossRef PubMed;
(b) Y. Nagata, T. Nishikawa and M. Suginome, J. Am. Chem. Soc., 2014, 136, 15901–15904 CrossRef CAS;
(c) Y. Mimura, S. Kitamura, M. Shizuma, M. Kitamatsu, M. Fujiki and Y. Imai, ChemistrySelect, 2017, 2, 7759–7764 CrossRef CAS;
(d) S. Nakanishi, N. Hara, N. Kuroda, N. Tajima, M. Fujiki and Y. Imai, Org. Biomol. Chem., 2018, 16, 1093–1100 RSC;
(e) K. Takaishi, K. Iwachido and T. Ema, J. Am. Chem. Soc., 2020, 142, 1774–1779 Search PubMed;
(f) L. Ji, Y. Liu, Z. Li, G. Ouyang and M. Liu, Chem. Commun., 2021, 57, 11314–11317 Search PubMed.
-
(a) T. Kimoto, T. Amako, N. Tajima, R. Kuroda, M. Fujiki and Y. Imai, Asian J. Org. Chem., 2013, 2, 404–410 CrossRef CAS;
(b) K. Nakabayashi, T. Amako, N. Tajima, M. Fujiki and Y. Imai, Chem. Commun., 2014, 50, 13228–13230 Search PubMed;
(c) M. Gon, R. Sawada, Y. Morisaki and Y. Chujo, Macromolecules, 2017, 50, 1790–1802 CrossRef CAS;
(d) G. Albano, G. Pescitelli and L. Di Bari, Chem. Rev., 2020, 120, 10145–10243 Search PubMed;
(e) M. Ikeshita, M. Mizugaki, T. Ishikawa, K. Matsudaira, M. Kitahara, Y. Imai and T. Tsuno, Chem. Commun., 2022, 58, 7503–7506 Search PubMed.
-
(a) N. Saleh, B. Moore II, M. Srebro, N. Vanthuyne, L. Toupet, J. A. Gareth Williams, C. Roussel, K. K. Deol, G. Muller, J. Autschbach and J. Crassous, Chem.–Eur. J., 2015, 21, 1673–1681 CrossRef CAS;
(b) S. Pascal, C. Besnard, F. Zinna, L. D. Rari, B. Le Guennic, D. Jacquemin and J. Lacour, Org. Biomol. Chem., 2016, 14, 4590–4594 Search PubMed;
(c) H. Sakai, T. Kubota, J. Yuasa, Y. Araki, T. Sakanoue, T. Takenobu, T. Wada, T. Kawai and T. Hasobe, Org. Biomol. Chem., 2016, 14, 6738–6743 RSC;
(d) T. Otani, A. Tsuyuki, T. Iwachi, S. Someya, K. Tateno, H. Kawai, T. Saito, K. S. Kanyiva and T. Shibata, Angew. Chem., Int. Ed., 2017, 56, 3906–3910 CrossRef CAS;
(e) K. Takaishi, M. Yasui and T. Ema, J. Am. Chem. Soc., 2018, 140, 5334–5338 CrossRef CAS;
(f) A. H. G. David, R. Casares, J. M. Cuerva, A. G. Campaña and V. Blanco, J. Am. Chem. Soc., 2019, 141, 18064–18074 Search PubMed;
(g) E. Yen-Pon, F. Buttard, L. Frédéric, P. Thuéry, F. Taran, G. Pieters, P. A. Champagne and D. Audisio, JACS Au, 2021, 1, 807–818 CrossRef CAS PubMed.
-
(a) F. Jäkle, Chem. Rev., 2010, 110, 3985–4022 CrossRef;
(b) Y.-L. Rao and S. Wang, Inorg. Chem., 2011, 50, 12263–12274 Search PubMed;
(c) G. Wesela-Bauman, P. Ciećwierz, K. Durka, S. Luliński, J. Serwatowski and K. Woźniak, Inorg. Chem., 2013, 52, 10846–10859 CrossRef CAS PubMed;
(d) D. Frath, J. Massue, G. Ulrich and R. Ziessel, Angew. Chem., Int. Ed., 2014, 53, 2290–2310 CrossRef CAS PubMed;
(e) M. Gon, K. Tanaka and Y. Chujo, Bull. Chem. Soc. Jpn., 2019, 92, 7–18 Search PubMed.
-
(a) H. Yersin, Highly Efficient OLEDs with Phosphorescent Materials, Wiley-VCH, Weinheim, 2008 Search PubMed;
(b) L. Xiao, Z. Chen, B. Qu, J. Luo, S. Kong, Q. Gong and J. Kido, Adv. Mater., 2011, 23, 926–952 CrossRef CAS PubMed;
(c) D. Li, H. Zhang and Y. Wang, Chem. Soc. Rev., 2013, 42, 8416–8433 Search PubMed.
-
(a) D. Frath, S. Azizi, G. Ulrich, P. Retailleau and R. Ziessel, Org. Lett., 2011, 13, 3414–3417 CrossRef CAS PubMed;
(b) Y.-W. Chen, Y.-C. Lin, H.-M. Kuo and C. K. Lai, J. Mater. Chem. C, 2017, 5, 5465−–5477 RSC;
(c) M. Urban, K. Durka, P. Jankowski and S. Luliński, J. Org. Chem., 2017, 82, 8234–8241 CrossRef CAS PubMed;
(d) P. A. A. M. Vaz, J. Rocha, A. M. S. Silva and S. Guieu, New J. Chem., 2018, 42, 18166–18171 Search PubMed.
-
(a) F. Zinna, T. Bruhn, C. A. Guido, J. Ahrens, M. Bröring, L. Di Bari and G. Pescitelli, Chem.–Eur. J., 2016, 22, 16089–16098 Search PubMed;
(b) Y. Gobo, M. Yamamura, T. Nakamura and T. Nabeshima, Org. Lett., 2016, 18, 2719–2721 Search PubMed;
(c) Z. Jiang, X. Wang, J. Ma and Z. Liu, Sci. China: Chem., 2019, 62, 355–362 CrossRef CAS;
(d) C. Maeda, K. Nagahata, T. Shirakawa and T. Ema, Angew. Chem., Int. Ed., 2020, 59, 7813–7817 CrossRef CAS PubMed;
(e) C.-H. Chen and W.-H. Zheng, Org. Chem. Front., 2021, 8, 6622–6627 Search PubMed;
(f) K. Li, H. Ji, Z. Yang, W. Duan, Y. Ma, H. Liu, H. Wang and S. Gong, J. Org. Chem., 2021, 86, 16707–16715 CrossRef CAS PubMed;
(g) W. Duan, H. Ji, Z. Yang, Q. Yao, Y. Huo, X. Ren, J. Zhao and S. Gong, Dalton Trans., 2021, 50, 12963–12969 RSC;
(h) A. Macé, K. Hamrouni, E. S. Gauthier, M. Jean, N. Vanthuyne, L. Fréderic, G. Pieters, E. Caytan, T. Roisnel, F. Aloui, M. Srebro-Hooper, B. Carboni, F. Berrée and J. Crassous, Chem.–Eur. J., 2021, 27, 7959–7967 CrossRef;
(i) L. Cui, H. Shinjo, T. Ichiki, K. Deyama, T. Harada, K. Ishibashi, T. Ehara, K. Miyata, K. Onda, Y. Hisaeda and T. Ono, Angew. Chem., Int. Ed., 2022, 61, e202204358 Search PubMed;
(j) M. Ikeshita, T. Suzuki, K. Matsudaira, M. Kitahara, Y. Imai and T. Tsuno, Phys. Chem. Chem. Phys., 2022, 24, 15502–15510 Search PubMed.
-
(a) M. Ikeshita, S. Furukawa, T. Ishikawa, K. Matsudaira, Y. Imai and T. Tsuno, ChemistryOpen, 2022, 11, e202100277 Search PubMed;
(b) M. Ikeshita, T. Yamamoto, S. Watanabe, M. Kitahara, Y. Imai and T. Tsuno, Chem. Lett., 2022, 51, 832–835 Search PubMed.
- M. N. Burnett and C. K. Johnson, ORTEP-III: Oak Ridge Thermal Ellipsoid Plot-Program for Crystal Structure Illustrations, Report ORNL-6895, Oak Ridge National Laboratory, Oak Ridge, 1996 Search PubMed.
- H. H. T. Al-Sharif, R. Ziessel, P. G. Waddell, C. Dixon and A. Harriman, J. Phys. Chem. A, 2020, 124, 2160–2172 Search PubMed.
- T. Smith and J. Guild, Trans. Opt. Soc., 1931, 33, 73–134 CrossRef.
- F. S. Richardson and J. P. Riehl, Chem. Rev., 1977, 77, 773–792 Search PubMed.
- H. Tanaka, Y. Inoue and T. Mori, ChemPhotoChem, 2018, 2, 386–402 Search PubMed.
- L. Rosenfeld, Z. Phys., 1929, 52, 161–174 CrossRef.
|
This journal is © The Royal Society of Chemistry 2022 |