DOI:
10.1039/D2RA07065K
(Paper)
RSC Adv., 2022,
12, 35221-35226
Visible light-induced photoredox catalyzed C–N coupling of amides with alcohols†
Received
7th November 2022
, Accepted 2nd December 2022
First published on 8th December 2022
Abstract
A visible-light-mediated method for the construction of N-monoalkylated products from easily available benzamides and benzyl alcohol in the presence of eosin Y has been developed. The reaction proceeded smoothly, for a wide range of derivatives of benzamides and benzyl alcohols, to give the desired products in good to excellent yields. Biological studies, such as those on drug-likeness and molecular docking, are carried out on the molecules.
1. Introduction
Organic compounds containing nitrogen are the most important in pharmaceutical molecules and agricultural chemicals. The basic building blocks of life, such as proteins, DNA, and RNA, are nitrogen-containing organic structural units. C–N bonds are found in more than 80% of commonly used medications. In current synthetic chemistry, green and mild methodology for preparing different C–N bonds to replace old harsh preparation protocols is always a hotspot.1 The technique of N-alkylation of amides is well-known, efficient, and essential in the synthesis of natural products, polymers, and peptides.2–4 Alcohols would be an appropriate option as alkylating reagents for the production of C–N bonds from a green chemistry perspective.5–13 When compared to other reagents used for N-alkylation of amides, alcohols are widely available and relatively inexpensive. N-Monoalkylated product is a versatile building block that is commonly utilized in the production of many bioactive compounds14–17 and pharmaceuticals (Fig. 1).
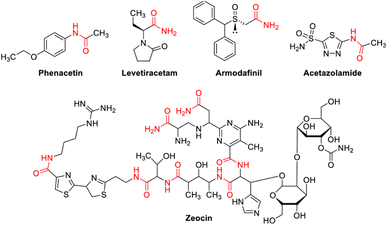 |
| Fig. 1 Some commercially available amide drugs. | |
They are also generally non- or less-toxic and easier to handle. Alcohols, on the other hand, have a poor electrophilicity, required either harsh conditions or further processes. These reactions usually required higher temperatures and/or catalyst loadings, because amides are less nucleophilic than amines. In 2009, Fujita and co-workers developed a method for N-alkylation of amides with alcohols using iridium catalysts.18 Watanabe and Jenner19,20 and some other researcher21,22 reported successful use of alcohols as alkylating reagents in the N-alkylation of amides using ruthenium and rhodium catalysts at high temperatures. Dean and co-workers reported N-alkylation of amides with alcohols utilizing heterogeneous Ag/Mo oxides in 2011.23 Alcohols were also reported as alkylating reagents in N-alkylation catalyzed by copper,24 ruthenium,25 and iridium catalysts26 in the same year. Sun and coworkers27 used ruthenium/iridium dual catalyst systems for the alkylation of aldoximes with alcohols to produce N-monoalkylated amide. Transition metal based photocatalysts, such as iridium, ruthenium, and polypyridyl complexes, on the other hand, have been associated with numerous of disadvantages, including high cost, low sustainability, and low toughness, possibility of toxicity, as well as being inconvenient during separation.28–30
In response to visible light stimulation, the photochemistry of eosin Y has been extensively studied, and it has been discovered that eosin Y undergoes rapid intersystem crossing to the lowest energy triplet state.31,32 Metal-free organic dyes, particularly eosin Y, were used instead of metal-based photo-redox catalysts because they are more cost-effective and environment friendly.33,34 Visible-light mediated photoredox catalysis for single electron transfer (SET) has emerged in recent years as a viable, versatile, cost-effective, and eco-friendly method for essential chemical transformations such as oxidation/reduction and C–C/C–X bond synthesis in heterocyclic compounds.35–37a–d In presence of visible light, an organo-photoredox catalyst has the unique ability to activate air oxygen, a green and natural oxidizing agent, for organic reactions involving SET.38,39On the basis of above mentioned previous work, related studies on C–N coupling and in continuation of our work40–58 on visible light induced photoredox catalysed synthesis, we have proposed eosin Y catalyzed visible-light-induced synthetic protocol for the formation C–N bond in N-monoalkylated product from easily available benzamides and benzyl alcohol (Scheme 1). To the best of our knowledge, this is the first example of photoredox catalysis for the synthesis of N-benzylbenzamide. This novel method utilizes visible light photoredox catalysis, which is mechanistically attractive for the production of N-monoalkylated product and its derivatives.
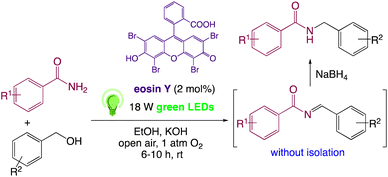 |
| Scheme 1 Photoredox catalyzed C–N coupling of amides with alcohols. | |
2. Results and discussion
In order to realize our idea and optimize the reaction conditions, the key reaction of benzamide (1a) and benzyl alcohol (2a), in presence of base with a catalytic amount of eosin Y in a solvent under irradiation with green LEDs [18 W] was carried out (Table 1). By applying several catalysts and bases, we first focused on optimizing the reaction conditions. We were delighted to obtain the desired substituted N-monoalkylated product 3a, in 96% yield (Table 1, entries 1 and 2). Then, the control experiments were carried out, which show that eosin Y, base and visible light are essential for the reaction, because in the absence of any of the reagents/reaction parameters either the product was not detected (n.d.) or was formed in traces (Table 1, entries 2 versus 13, 14 and 16). Further, the reaction was carried out at room temperature (rt) in EtOH with 0.5 equivalents of a base and using 0.2 mol% of eosin Y for 6 hours (Table 1, entries 1 and 8–10). The use of KOH produced the N-monoalkylated product in the highest isolated yield (Table 1, entry 1). The quantity of KOH could be decreased to 0.2 equivalents, without any significant loss in yield (Table 1, entry 2). In any case, the product yields were unaffected by the longer reaction times. EtOH as a solvent was found to be superior for increasing yield and accelerating reaction times for the comparable product. In this approach, we reduce waste, tedious workup, and process costs.
Table 1 Optimization of reaction conditionsa,g
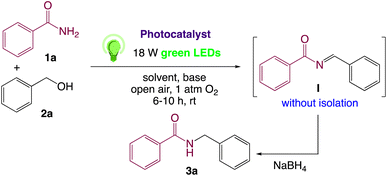
|
Entry |
Catalyst (mol%) |
Solvent |
Base (equiv.) |
Time (h) |
Yieldb (%) |
Reaction conditions: 1a (1.0 mmol), 2a (1.0 mmol), catalyst (mol%), in 3 mL solvent irradiated using Luxeon Rebel high power green LEDs [18 W] at rt for 6–10 h. Isolated yield of the pure product 3a. Reaction was performed in the dark. Reaction was carried out without the catalyst. 18 W CFL (compact fluorescent lamp, Philips) was used. Reaction was carried out without the base. After synthesis of I, further, reactions were carried out in presence of NaBH4 without isolation. |
1 |
Eosin Y (2) |
EtOH |
KOH (0.5) |
6 |
96 |
2 |
Eosin Y (2) |
EtOH |
KOH (0.2) |
6 |
96 |
3 |
Rose bengal (2) |
EtOH |
KOH (0.2) |
6 |
76 |
4 |
Fluorescein (2) |
EtOH |
KOH (0.2) |
6 |
74 |
5 |
Nile red (2) |
EtOH |
KOH (0.2) |
6 |
78 |
6 |
Perylene (2) |
EtOH |
KOH (0.2) |
6 |
80 |
7 |
Rhodamine B (2) |
EtOH |
KOH (0.2) |
6 |
83 |
8 |
Eosin Y (2) |
DMSO |
NaHCO3 (0.5) |
6 |
40 |
9 |
Eosin Y (2) |
DMF |
K2CO3 (0.5) |
6 |
82 |
10 |
Eosin Y (2) |
Toluene |
NaOH (0.5) |
6 |
86 |
11 |
Eosin Y (1) |
EtOH |
KOH (0.2) |
6 |
65 |
12 |
Eosin Y (3) |
EtOH |
KOH (0.2) |
6 |
96 |
13 |
Eosin Y (2) |
EtOH |
KOH (0.2) |
10 |
Tracesc |
14 |
— |
EtOH |
KOH (0.2) |
10 |
n.dd |
15 |
Eosin Y (2) |
EtOH |
KOH (0.2) |
10 |
60e |
16 |
Eosin Y (2) |
EtOH |
— |
10 |
n.df |
We then turned our focus to investigating the adaptability of various substrates or anticipated reaction conditions as we continued to search for the best reaction conditions for our model reaction (Scheme 2). The scope of the present protocol across a range of benzamide and benzyl alcohol incorporating various substituents were studied. One important insight was that at the reaction conditions used, benzyl alcohol and benzamide with strong electron withdrawing groups as well as electron donating groups were well tolerated, and N-monoalkylated products were produced in good to excellent yields (Table 2). However, it was observed that benzyl alcohol and benzamide with electron-donating groups like methoxy and methyl at various positions exhibited improved reactivity and produced the corresponding products in good to excellent yields (3e, 3f and 3k). On the other hand, substrate containing electron-withdrawing groups, such as bromo and fluoro provided the corresponding products in good yields (3c, 3d and 3j). Further, it was also observed that the condensation reaction carried out between alkyl amide and alkyl alcohol then the yield of resultant product was found very low (3m).
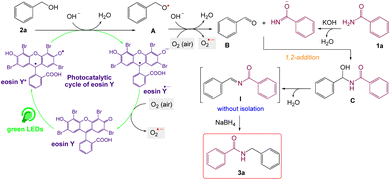 |
| Scheme 2 Plausible mechanism for C–N coupling of amides with alcohols.60,61 | |
Table 2 Scope of the reaction
On the basis of the observed reactivity and above investigations, even though the exact reaction mechanism is not clear, a plausible mechanistic pathway is depicted in Scheme 2. On absorption of visible light irradiation, the photo-excited eosin Y would induce an event of electron abstraction from benzyl alcohol 2a, whose oxidation potential might be significantly decreased under alkaline conditions, and thus gave rise to the highly active radical intermediate A and radical anion eosin Y. This radical anion eosin Y was able to be expediently oxidized by the molecular oxygen to go back to the ground state. The key intermediate, benzaldehyde B, would then be produced by further oxidizing and deprotonating the radical intermediate species A under the synergistic interactions of KOH and oxygen. Then, it went through a sequence of nucleophilic addition and dehydration reactions to produce the intermediate I without isolation, which further reduced59 by NaBH4 to give the desired product 3a.
3. Molecular docking
\It is usually known that drug design plays an important role in the pharma industry. This compound, referred to as a ligand, interacts with the appropriate protein that has been chosen using online drug target prediction Swiss ADME-Target prediction. By using Chimera 1.14 (ref. 62) and Autodock vina63 software, N-benzylbenzamide molecule is docked with 1U3U protein belongs to oxidoreductase domain.64 The best binding energy is shown −7.3 kcal mol−1 is obtained as shown in Fig. 2 and the values are shown in Table 3. The low value of the binding energy indicates the bioactive nature of the molecule. The H-bond distance between bonded residue and ligand is 2.39 Å indicating the suitability of this ligand with given protein. The number of residue that contain by the protein 1U3U are 3 and KI value is 4.46.
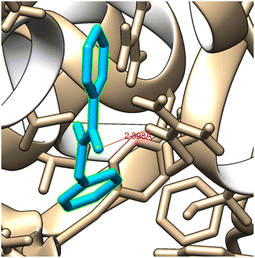 |
| Fig. 2 Ligand N-benzylbenzamide embedded in the active site of 1U3U protein. | |
Table 3 Molecular docking and hydrogen bonding with centromere related protein inhibitors protein targets
Target molecule |
N-Benzylbenzamide |
Protein (PDB ID) |
1U3U |
No. of residues |
3 |
Bond distance (Å) |
2.39 |
Inhibition constant (micromolar) |
4.46 |
Binding energy (kcal mol−1) |
−7.3 |
Reference RMSD (Å) |
9.34 |
4. Drug-likeness
In order to produce effective and efficient results in drug development, the structural property of the ligands plays a significant role for guidelines, described as drug-likeness. The calculations are based on a variety of rules, including QED, Lipinski's rule, MDDR-like rule, Veber rule, Ghose filter, and BBB rule, CMC-50 rule.65 N-Benzylbenzamide and its derivatives demonstrate a wide range of activities that are applied to the drug-likeness rule and efficiency is obtained. For the title compound and its derivatives, the essential ADME parameters such as Hydrogen bond donors (HBD), Hydrogen bond acceptors (HBA), molar refractivity (MR), topological polar surface area (TPSA), blood–brain barrier penetration (BBB), log
kp, and bioavailability score are calculated as given in Table 4. The values of HBD and HBA should be less than 10. Here, all of the molecule's values are less than 3. The maximum TPSA value is 140 A2. For all target molecules, this value is between 29 and 39. The molar refractivity should also range from 40 to 130.66,67 The MR value of N-Benzylbenzamide is 63.93 and the MR value for N-(4-methoxybenzyl)benzamide and N-[(4-fluorophenyl)methyl]benzamide is 70.42 and 63.88 respectively. Table 4 reveals that the GI absorption is high for all target molecules, the skin's permeability (log
kp) is between −6.01 and −6.21. The BBB is permeant available for all the target molecules, and the bioavailability score of N-benzylbenzamide and its derivative is the same as 0.55. The above comparison shows that the title compound (N-benzylbenzamide) and its derivatives are useful building blocks, frequently used in the synthesis of numerous bioactive components and medicines.
Table 4 ADME properties of N-benzylbenzamide and its derivativesa
ADME properties |
Target molecule |
N-Benzylben-zamide |
N-(4-Methoxybenz-yl)benzamide |
N-[(4-Fluorophenyl)-methyl]benza-mide |
HBD – hydrogen bond donor, HBA – hydrogen bond acceptor, MR – molar refractivity, TPSA – topological polar surface area, GI – gastrointestinal, BBB – blood–brain barrier penetration and log kp – skin permeability. |
HBD |
1 |
1 |
1 |
HBA |
1 |
2 |
2 |
MR |
63.93 |
70.42 |
63.88 |
TPSA A2 |
29.10 |
38.33 |
29.10 |
GI absorption |
High |
High |
High |
BBB permeant |
Yes |
Yes |
Yes |
CYP1A2 inhibitor |
Yes |
Yes |
Yes |
log kp (cm s−1) |
−6.01 |
−6.21 |
−6.04 |
Lipinski violations |
0 |
0 |
0 |
Bioavailability score |
0.55 |
0.55 |
0.55 |
5. Conclusions
In conclusion, we have established an effective synthetic protocol for N-monoalkylated product and its derivatives, via C–N bond formation by interaction of benzamide and benzyl alcohol with high reactivity and good selectivity. A variety of substrate amides were converted to the desired product with excellent yield along with low catalyst loading. This green synthetic pathway is superior to other synthetic process that uses harmful and corrosive chemicals. The range of substrates for visible light photoredox reaction is expanded by this synthesis. The proposed method also offers other benefits of green chemistry such high atom economy, shortened reaction time, and high efficiency. Molecular docking studies carried out on the 1U3U protein associated with the centromere protein inhibitor exhibited binding energy of −7.3 kcal mol−1, resulting it's application in the medical field.
Conflicts of interest
There are no conflicts to declare.
References
- D. Ma, S. Zhai, Y. Wang, A. Liu and C. Chen, Front. Chem., 2019, 7, 1–8 CrossRef PubMed.
- C. L. Allen and J. M. J. Williams, Chem. Soc. Rev., 2011, 40, 3405–3415 RSC.
- J. M. Humphrey and A. R. Chamberlin, Chem. Rev., 1997, 97, 2243–2266 CrossRef CAS PubMed.
- V. R. Pattabiraman and J. W. Bode, Nature, 2011, 480, 471–479 CrossRef CAS PubMed.
- R. H. Crabtree, Organometallics, 2011, 30, 17–19 CrossRef CAS.
- E. Emer, R. Sinisi, M. G. Capdevila, D. Petruzziello, F. D. Vincentiis and P. G. Cozzi, Eur. J. Org. Chem., 2011, 2011, 647–666 CrossRef.
- G. Guillena, D. J. Ramón and M. Yus, Chem. Rev., 2010, 110, 1611–1641 CrossRef CAS PubMed.
- G. Guillena, D. J. Ramón and M. Yus, Angew. Chem., Int. Ed., 2007, 46, 2358–2364 CrossRef CAS PubMed.
- M. H. S. A. Hamid, P. A. Slatford and J. M. J. Williams, Adv. Synth. Catal., 2007, 349, 1555–1575 CrossRef CAS.
- M. Noji, T. Ohno, K. Fuji, N. Futaba, H. Tajima and K. Ishii, J. Org. Chem., 2003, 68, 9340–9347 CrossRef CAS PubMed.
- H. Qin, N. Yamagiwa, S. Matsunaga and M. Shibasaki, Angew. Chem., Int. Ed., 2007, 46, 409–413 CrossRef CAS PubMed.
- S. Bojja, P. S. Reddy, M. A. Reddy and B. Neelima, Tetrahedron Lett., 2007, 48, 8174–8177 CrossRef.
- A. J. A. Watso and J. M. J. Williams, Science, 2010, 329, 635–636 CrossRef PubMed.
- R. Blocher, C. Lamers, S. K. Wittmann, D. Merk, M. Hartmann, L. Weizel, O. Diehl, A. Bruggerhoff, M. Bo, A. Kaiser, T. Schader, T. G. Bel, M. Grundmann, C. Angioni, J. Heering, G. Geisslinger, M. Wurglics, E. Kostenis, B. B. Ne, D. Steinhilbe, M. S. Zsilavecz, A. S. Kahnt and E. Proschak, J. Med. Chem., 2016, 59(1), 61–81 CrossRef PubMed.
- H. S. Baek, Y. D. Hong, C. S. Lee, H. S. Rho, S. S. Shin, Y. H. Park and Y. H. Joo, ACS Med. Chem. Lett., 2012, 22, 2110–2113 CrossRef CAS PubMed.
- H. Zhu, W. Li, W. Shuai, Y. Liu, L. Yang, Y. Tan, T. Zheng, H. Yao, J. Xu, Z. Zhu, D. H. Yang, Z. S. Chencand and S. Xu, Eur. J. Med. Chem., 2021, 216, 113316 CrossRef CAS PubMed.
- H. J. Shin, C. T. Oh, T. R. Kwon, H. S. Beak, Y. H. Joo, J. H. Kim, C. S. Lee, J. H. Lee, B. J. Kim, S. S. Shin and E. S. Park, Int. J. Mol. Med., 2015, 36, 1353–1360 CrossRef CAS PubMed.
- K. I. Fujita, A. Komatsubara and R. Yamaguchi, Tetrahedron, 2009, 65, 3624–3828 CrossRef CAS.
- G. Jenner, J. Mol. Catal., 1989, 55, 241–246 CrossRef CAS.
- Y. Watanabe, T. Ohta and Y. Tsuji, Bull. Chem. Soc. Jpn., 1983, 56, 2647–2651 CrossRef CAS.
- G. C. Y. Choo, H. Miyamura and S. Kobayashi, Chem. Sci., 2015, 6, 1719–1727 RSC.
- W. Yao, Y. Zhang, H. Zhu, C. Ge and D. Wang, Chin. Chem. Lett., 2020, 31, 701–705 CrossRef CAS.
- X. Cui, Y. Zhang, F. Shi and Y. Deng, Chem.–Eur. J., 2011, 17, 1021–1028 CrossRef CAS PubMed.
- A. M. Asencio, D. J. Ramón and M. Yus, Tetrahedron, 2011, 67, 3140–3149 CrossRef.
- A. J. A. Watson, A. C. Maxwell and J. M. J. Williams, J. Org. Chem., 2011, 76, 2328–2331 CrossRef CAS PubMed.
- C. Liu, S. Liao, Q. Li, S. Feng, Q. Sun, X. Yu and Q. Xu, J. Org. Chem., 2011, 76, 5759–5773 CrossRef CAS PubMed.
- F. Li, P. Qu, J. Ma, X. Zou and C. Sun, ChemCatChem, 2013, 5, 2178–2182 CrossRef CAS.
- S. D. Tambe, M. S. Jadhav, R. S. Rohokale and U. A. Kshirsagar, Eur. J. Org. Chem., 2018, 2117–2121 CrossRef CAS.
- Y. Zhou, J. Wang, Z. Gu, S. Wang, W. Zhu, J. L. Acenã, V. A. Soloshonok, K. Izawa and H. Liu, Chem. Rev., 2016, 116, 422–518 CrossRef CAS PubMed.
- T. Xu, C. W. Cheung and X. Hu, Angew. Chem., Int. Ed., 2014, 53, 4910–4914 CrossRef CAS PubMed.
- L. Buzzetti, G. E. M. Crisenza and P. Melchiorre, Angew. Chem., Int. Ed., 2019, 58, 3730–3747 CrossRef CAS PubMed.
- A. A. Soto, K. Kaastrup, S. Kim, K. Ugo-Beke and H. D. Sikes, ACS Catal., 2018, 8, 6394–6400 CrossRef.
- L. Lin, X. Bai, X. Ye, X. Zhao, C. Tan and Z. Jiang, Angew. Chem., Int. Ed., 2017, 56, 13842–13846 CrossRef CAS PubMed.
- M. Majek and A. J. V. Wangelin, Angew. Chem., Int. Ed., 2014, 53, 1–6 CrossRef.
- M. A. Ansari, D. Yadav, S. Soni, A. Srivastava and M. S. Singh, J. Org. Chem., 2019, 84, 5404–5412 CrossRef CAS PubMed.
- K. Wang, L. Meng and L. Wang, J. Org. Chem., 2016, 81, 7080–7087 CrossRef CAS PubMed.
-
(a) K. Muralirajan, R. Kancherla and M. Rueping, Angew. Chem., Int. Ed., 2018, 57, 14787–14791 CrossRef CAS PubMed;
(b) A. G. Condie, J. C. Gonzalez-Gomez and C. R. J. Stephenson, J. Am. Chem. Soc., 2010, 132, 1464–1465 CrossRef CAS PubMed;
(c) S. W. Wang, J. Yu, Q. Y. Zhou, S. Y. Chen, Z. H. Xu and S. Tang, ACS Sustainable Chem. Eng., 2019, 7, 10154–10162 CrossRef CAS;
(d) X. Y. Yu, Q. Q. Zhao, J. Chen, W. J. Xiao and J. R. Chen, Acc. Chem. Res., 2020, 53, 1066–1083 CrossRef CAS PubMed.
- X. Lang, J. Zhao and X. Chen, Angew. Chem., Int. Ed., 2016, 55, 4697–4700 CrossRef CAS PubMed.
- R. S. Rohokale, B. Koenig and D. D. Dhavale, J. Org. Chem., 2016, 81, 7121–7126 CrossRef CAS PubMed.
- V. Srivastava, P. K. Singh and P. P. Singh, Croat. Chem. Acta, 2014, 87, 91–95 CrossRef.
- V. Srivastava, P. K. Singh and P. P. Singh, Croat. Chem. Acta, 2015, 88, 59–65 CrossRef CAS.
- V. Srivastava, P. K. Singh and P. P. Singh, Croat. Chem. Acta, 2015, 88, 227–233 CrossRef CAS.
- V. Srivastava, P. K. Singh and P. P. Singh, Asian J. Chem., 2016, 28, 2159–2638 CrossRef CAS.
- V. Srivastava, P. K. Singh and P. P. Singh, Rev. Roum. Chim., 2016, 61, 755–761 Search PubMed.
- V. Srivastava, P. K. Singh and P. P. Singh, Croat. Chem. Acta, 2017, 90, 435–441 CrossRef CAS.
- V. Srivastava, P. K. Singh, S. Kanaujia and P. P. Singh, New J. Chem., 2018, 42, 688–691 RSC.
- P. K. Singh, P. P. Singh and V. Srivastava, Croat. Chem. Acta, 2018, 91, 383–387 CrossRef.
- V. Srivastava, P. K. Singh and P. P. Singh, Tetrahedron Lett., 2019, 60, 40–43 CrossRef CAS.
- V. Srivastava, P. K. Singh and P. P. Singh, Tetrahedron Lett., 2019, 60, 1333–1336 CrossRef CAS.
- V. Srivastava, P. K. Singh and P. P. Singh, Tetrahedron Lett., 2019, 60, 151041–151638 CrossRef CAS.
- A. Srivastava, P. K. Singh, A. Ali, P. P. Singh and V. Srivastava, RSC Adv., 2020, 10, 39495–39508 RSC.
- V. Srivastava, P. K. Singh and P. P. Singh, Rev. Roum. Chim., 2020, 65, 221–226 CrossRef.
- V. Srivastava, P. K. Singh, A. Srivastava and P. P. Singh, RSC Adv., 2021, 11, 14251–14259 RSC.
- P. P. Singh and V. Srivastava, Org. Biomol. Chem., 2021, 19, 313–321 RSC.
- P. P. Singh, P. K. Singh, M. Z. Beg, A. Kashyap and V. Srivastava, Synth. Commun., 2021, 51(20), 3033–3058 CrossRef CAS.
- V. Srivastava, P. K. Singh, S. Tivari and P. P. Singh, Org. Chem. Front., 2022, 9, 1485–1507 RSC.
- V. Srivastava, P. K. Singh and P. P. Singh, J. Photochem. Photobiol., C, 2022, 50, 100488 CrossRef CAS.
- P. P. Singh and V. Srivastava, RSC Adv., 2022, 12, 18245–18265 RSC.
- A. Rahman, A. Basha, N. Waheed and S. Ahmed, Tetrahedron Lett., 1976, 219–222 CrossRef.
- W. Yao, Y. Zhang, H. Zhu, C. Ge and D. Wang, Chin. Chem. Lett., 2020, 53, 701–705 CrossRef.
- S. Kerdphon, X. Quan, V. S. Parihar and P. G. Andersson, J. Org. Chem., 2015, 80, 11529–11537 CrossRef CAS PubMed.
- D. R. Roy, R. Parthasarathi, B. Maity, V. Subramanian and P. K. Chattaraj, Bioorg. Med. Chem., 2005, 13, 3405–3412 CrossRef CAS PubMed.
- E. F. Pettersen, T. D. Goddard, C. C. Huang, G. S. Couch, D. M. Greenblatt, E. C. Meng and T. E. Ferrin, J. Comput. Chem., 2005, 25, 1605–1612 CrossRef PubMed.
- B. J. Gibbons and T. D. Hurley, Biochemistry, 2004, 43(39), 12555–12562 CrossRef CAS PubMed.
- H. Li, H. Wang, S. Kang, R. B. Silverman and T. L. Poulos, Biochemistry, 2016, 55, 3702–3707 CrossRef CAS PubMed.
- A. Daina, O. Michielin and V. Zoete, J. Chem. Inf. Model., 2014, 54, 3284–3301 CrossRef CAS PubMed.
- C. A. Lipinski, F. Lombardo, B. W. Dominy and P. J. Feeney, Adv. Drug Delivery Rev., 1997, 23, 3–25 CrossRef CAS.
|
This journal is © The Royal Society of Chemistry 2022 |
Click here to see how this site uses Cookies. View our privacy policy here.