DOI:
10.1039/D2RA05201F
(Paper)
RSC Adv., 2022,
12, 32619-32629
Sustainable synthesis of biomass-derived carbon quantum dots and their catalytic application for the assessment of α,β-unsaturated compounds†
Received
19th August 2022
, Accepted 2nd November 2022
First published on 14th November 2022
Abstract
Herein, we demonstrate a simple, reproducible, and environment-friendly strategy for the synthesis of carbon quantum dots (CQDs) utilizing the mango (Mangifera indica) kernel as a renewable green carbon source. Various analytical tools characterized the as-prepared CQDs. These fluorescent CQDs showed significant water solubility with a uniform size of about 6 nm. The as-synthesized CQDs show significantly enhanced catalytic activity for the production of α,β-unsaturated compounds from the derivatives of aromatic alkynes and aldehydes under microwave irradiation in aqueous media. A potential mechanistic pathway and role of carboxylic functionalities were also revealed via various control experiments. The protocol shows outstanding selectivity towards the assessment of α,β-unsaturated compounds over other possible products. A comparative evaluation suggested the as-synthesized CQDs show higher catalytic activity under microwave radiation as compared to the conventional ways. These recyclable CQDs represent a sustainable alternative to metals in synthetic organic chemistry. A cleaner reaction profile, low catalyst loading, economic viability and recyclability of the catalyst, atom economy, and comprehensive substrate applicability are additional benefits of the current protocol according to green chemistry.
Introduction
Chalcones (1,3-diphenylprop-2-ene-1-one) are α,β-unsaturated carbonyl compounds and an imperative forerunner for accessing flavonoids, isoflavonoids, and many other heterocyclic motifs.1 Due to this, the α,β-unsaturated carbonyl group, chalcones, and their substituted compounds display meaningful biological activities that are significant and auxiliary in drug design. They are also very useful intermediates in organic synthesis, especially for heterocycles.2 Due to their inherent ability to act as a Michael acceptor to nucleophilic amino acid residues, they have been used to synthesize compounds of biological relevance such as terahydromyricoid3 and the antibacterial reutericyclin.4 In addition, they exist in some natural products, such as honeybee queen juice secretion5 and caffeic acid6 (Fig. 1).
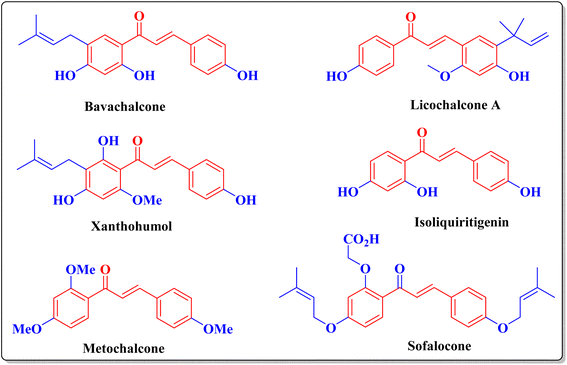 |
| Fig. 1 Examples of α,β-unsaturated compounds in natural products and pharmaceutical applications. | |
These compounds (1,3-diphenylprop-2-ene-1-one) can be simply produced by the “Claisen–Schmidt condensation” reaction.7 In the past decades, numerous methodologies have been developed by the chemists for the construction of chalcone by utilizing diverse basic catalysts like KOH,8 K2CO3,9 MgO/ZnO,10 organolithium,11 NaOH–Al2O3,12 modified phosphates,13 hydrotalcite,14 zeolites,15 alumina,16 barium hydroxides,17 MgO,18 calcined hydrotalcites,19 NaNO3,20 or KF modified natural phosphates,21 and aminopropyl-functionalized SBA-15
22 but many of the testified procedures involve some disadvantages such as using costly reagents, waste disposal problems, low yielded products, and prolonged reaction. Under strongly basic environments, side products often emerge due to self-condensation, polymerization, and rearrangement. Recently, many efforts have been made to modify the reaction, remove undesirable side products due to the self-condensation polymerization, and rearrangement avoiding bases. During the past few years, several researchers have been used various metal salts such as CuSO4,23 SnCl2,24 In(OTf)3,25 and AuCl3 as homogeneous catalysts26 to catalyzed this reaction under various conditions. The major task in the existing literature is the recovery of the catalyst.
Environmental pollution from the widespread use of metallic catalysts and hazardous solvents increases, which is a dangerous matter of concern to live beings.27 It is important to minimize metallic catalysts in chemical transformations because these metals are hazardous, and their appropriate discarding is complex. In addition, the use of small amounts of transition metals in pharmaceutical drugs can have serious toxic effects on living populations.28 In synthetic processes, hazardous and volatile solvents also contribute to chemical waste, causing serious adverse effects on the environment.29 thus, the execution of environmentally green and sustainable chemicals, including catalysts and solvents, has arisen as a major green chemistry goal and safer energy source.
Therefore, the evolution of metal-free catalysts, like carbocatalysts, would substantially replace the synthetic approaches of many conventional organic synthesis and purification processes. Over the past decades, carbon-based catalysts have been increasingly used in general organic reactions such as oxidation, reduction, and diverse coupling reactions. However, carbocatalysts have been employed as an alternative to metal catalysts in organic synthesis due to their great catalytic activity, selectivity, and non-toxicity.30 Some scanty reports are existing on the synthesis of α,β-unsaturated compounds utilizing graphene oxide (GO) as a carbocatalysts.31 The utilization of graphene oxide gave moderate yields of the desired product with the large catalyst loading. The preparation of GO requires harsh reaction conditions involving the use of detrimental agents. Furthermore, trace metals' participation on GO surfaces is possible in the reaction. Therefore, the design and development of more sustainable carbocatalysts are required.
Carbon quantum dots (CQDs) are the most growing topic in synthetic chemistry and materials science because of their marvelous properties. Because of the presence of the highly reactive surface area with different functionalities, it demonstrates enormous catalytic activity for several chemical transformations.32 Additionally, these materials show a high water solubility, huge surface area with rich functionalities, strong and tunable photoluminescence (PL), high stability, good biocompatibility, and non-toxicity.33 Newly, several research papers emphasized the synthesis method of CQDs, such as simple thermal treatment, arc discharge, laser ablation, acidic or electrochemical oxidation, pyrolysis using numerous chemical-based carbon sources.34 Most of these methodologies bear severe shortcomings such as costly starting material, tedious separation method, time-consuming procedure, multistep manufacturing, complex experimental setup, need for acidic conditions, and surface passivation. Thus, the development of the economic, efficient, and green synthesis of CQDs without using chemicals is of immense attention.
We reasoned that CQDs were defined as an exceptional catalyst for acid catalyzed reactions35 and might be used to avoid many of the limits and drawbacks inherent in the synthetic methods mentioned above related to the α,β-unsaturated compounds from a broad range of starting materials. As a part of our continuous efforts to explore the novel green protocols and nanomaterials synthesis.36 herein we report the synthesis of CQDs using mango (Mangifera indica) kernel as a renewable green resource. The as-prepared fluorescent CQDs have been characterized by utilizing various analytical tools. The catalytic activity of as-synthesized CQDs has been probed for the synthesis of α,β-unsaturated compounds from the derivatives of aromatic aldehyde and alkyne under microwave irradiation in aqueous media (Scheme 1). A potential mechanistic pathway and role of carboxylic functionalities were also revealed via various control experiments. The protocol shows outstanding selectivity towards the assessment of α,β-unsaturated compounds over other possible products.
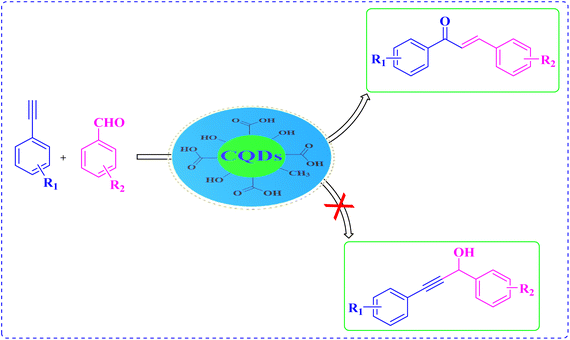 |
| Scheme 1 Assessment of α,β-unsaturated compounds. | |
Experimental
Common part and used instruments is presented in ESI.†
Preparation of carboxy functionalized CQDs
Typically, carboxy functionalized CQDs were manufactured by hydrothermal process. Firstly, we dried the mango (Mangifera indica) kernel part, then put it into a typical ceramic crucible, and carbonized it in a muffle furnace. The temperature was slowly raised to 300 °C with a heating rate of 10 °C min−1. The obtained solid compound cooled naturally and then crushed into a fine powder using pastel mortar. After cooling down naturally, the resultant dark black product was ground into fine powder. Then obtained 500 mg sample was poured into 100 ml ultrapure water and dispersed to get a black solution. The obtained solution was centrifuged (12
000 × g, 15 min), and finally CQDs were stored through filtration with a 0.22 μm membrane. We got a brown-yellow color solution of carbon quantum dots in daylight and the greenish color shown in the UV region. The obtained CQDs were kept at 4 °C for further characterization and use.
Method for α,β-unsaturated compound
To understand the reactivity and activity of the as-prepared catalyst with microwave-irradiation, benzaldehyde (2.0 mmol), phenylacetylene (2.0 mmol) with 10 ml of CQDs aqueous solution (5 mg ml−1) were used as a reactant under microwave-irradiation in aqueous medium using the maximum power of 400 W at 80 °C for 10 minutes. The conversion of the reaction was examined by TLC. The obtained product was separated using ethyl acetate. The solid compound was obtained after evaporation of the solvent using a rotary evaporator. The resulting residue was purified via recrystallization with EtOH.
Results and discussion
Preparation and characterization of CQDs
Nonetheless, a great deal of effort has developed towards synthesis of CQDs using green raw materials.37 However, the majority of them were synthesized through hydrothermal treatment in organic solvent using either harsh acids or bases or expensive equipment with additional requirement of surface passivation. Synthesis of CQDs with excellent physicochemical and fluorescent properties with minimal environmental impact is still a challenge. Green fluorescent CQDs have been synthesized in this study from the readily accessible starting material mango (Mangifera indica) kernel through the simple sustainable method. Mango kernel (Mangifera indica) was used as the carbon source in this study because it has a high concentration of dietary fibre, polyphenols, carotenoids, gallic acid, flavonoids, and other useful compounds.38
Various analytical tools including SEM, XRD, TEM EDAX, FT-IR, XPS, Raman, UV-vis, and PL were employed to characterize the as-prepared catalyst.
XRD analysis is employed to identify the crystallographic properties of CQDs (Fig. 2). The synthesized catalyst showed the characteristic broad diffraction peak of CQDs centered at 2θ = 24.3° lattice spacing of (002), indicating the presence of amorphous nature.39 XRD pattern can also be applied to estimate the size of CQDs utilizing the “Debye–Scherrer” equation. The size of the as-synthesized catalyst was found to be approximately 3 nm by this equation. The surface morphology and porous structure of synthesized carbon quantum dots were analyzed based on SEM images (Fig. 3). As shown in Fig. 4, the TEM image was used to confirm the formation of CQDs. The size distribution of the particles are shown in inset Fig. 4. It could be observed that the CQDs were well distributed and sphere-shaped, with an average size of 6 nm. The present sustainable methodology produces CQDs with a small size distribution that is equivalent to microwave and hydrothermal approaches,40 which is significant given how challenging it was to produce such a narrow size range utilising a natural carbon source.
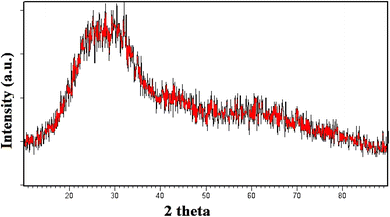 |
| Fig. 2 XRD pattern of CQDs. | |
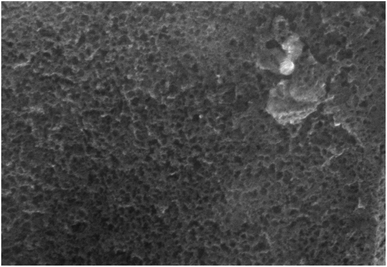 |
| Fig. 3 SEM image of CQDs. | |
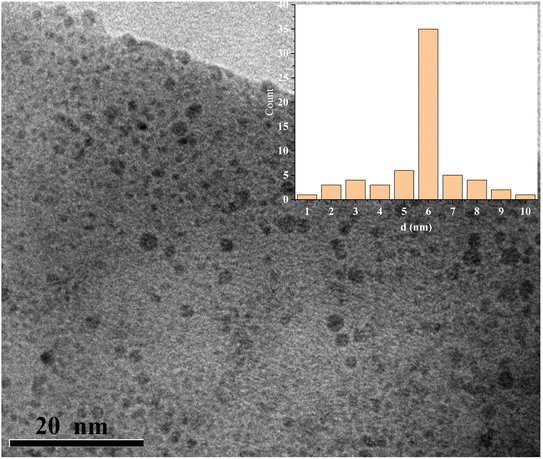 |
| Fig. 4 TEM image of CQDs. | |
In FT-IR spectra, the spectral broad band near 3437 cm−1 is correspond to the stretching and bending vibrations modes of the O–H and N–H bonds (Fig. 5). The spectral band at 1692 cm−1 corresponds to the C
O bond (carboxylic).41 The results proved that the as-synthesized catalyst has hydrophilic functionalities on its surface such as –OH, –NH2, and –COOH. In addition, the XPS spectrum was employed to detect the exact quantity of the element's composition on the surface of CQDs. Fig. 6a is the XPS spectrum of CQDs. The three peaks in the XPS spectrum at 399.74, 284.34, and 531.43 eV were allotted to N 1s, C 1s, and O 1s, individually.42
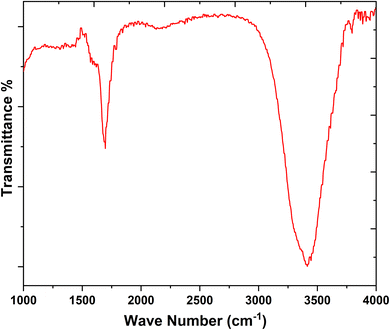 |
| Fig. 5 FT-IR spectra of CQDs. | |
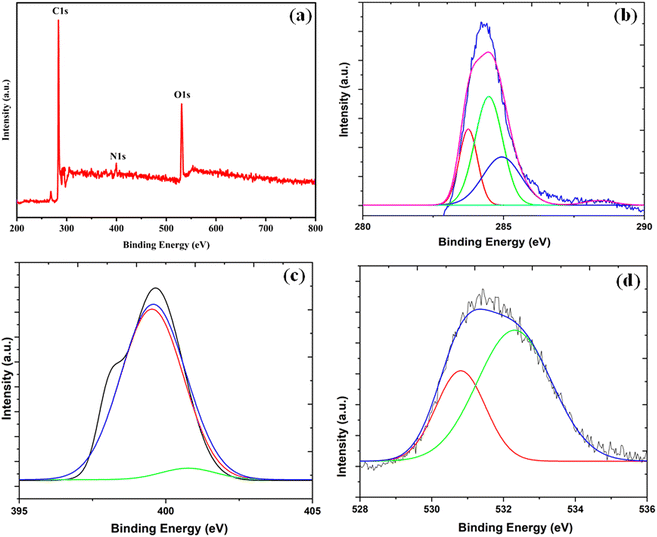 |
| Fig. 6 XPS spectrum of CQDs (a) complete survey, (b) C 1s spectra (c) N 1s spectra, (d) O 1s spectra. | |
The C 1s XPS spectrum reveals four peaks centered at 283.8, 284.6, 284.8, and 285.1 eV corresponding to C species in the forms of C
C, C–N, C–O and C
O (carboxylic) correspondingly,43 (Fig. 6b). In the deconvoluted N 1s spectrum, the two peaks at 399.79 and 399.86 eV related to C–N and N–H bonds were present (Fig. 6c). The deconvoluted O 1s spectrum reveals two peaks at 532.41 eV (C–OH bond) and 530.63 eV (C–O bond) (Fig. 6d). These results also confirm the formation of hydrophilic functionalities (–OH, –NH2, and –COOH) on the surface of the catalyst. This observation was also supported by the FT-IR spectrum.
Fig. 7 presents the optical properties of synthesized CQDs. It is shown strong green luminescence under UV light, indicating excellent optical properties. A band at 280 nm appeared in the UV-visible absorption spectrum of the CQDs. This was due to the transition of the C
C bond (π–π*) and the C
O bond (n–π*).44
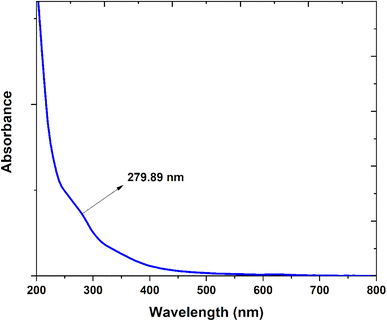 |
| Fig. 7 UV spectrum of CQDs. | |
Fig. 8 presents the fluorescent excitation and emission spectrum of CQDs. The fluorescent excitation spectra exhibited a peak at around 420 nm and the emission spectrum was exhibited under UV irradiation at 534 nm.45
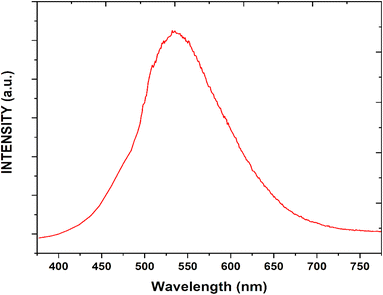 |
| Fig. 8 PL spectrum of CQDs. | |
Fig. 9 represents the chemical composition of carbon quantum dots, which was examined by EDAX analysis. The results represents that CQDs are composed of C (67.51%), N (1.03%) and O (31.46%).
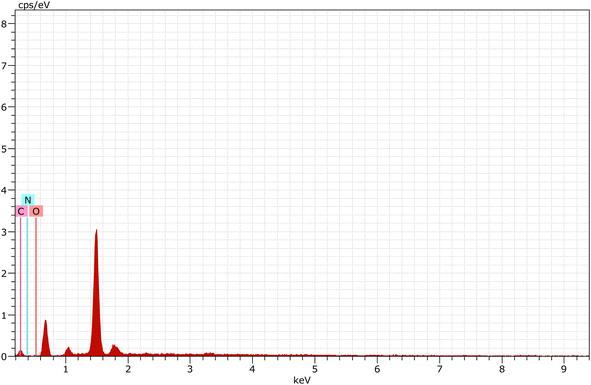 |
| Fig. 9 EDAX spectrum of CQDs. | |
Raman investigation revealed two unique Raman peaks at 1455 cm−1 and 1571 cm−1, referred to as the D (disorder) band and the G (graphite) band, respectively (Fig. 10). The intensity ratio of the D and G bands (ID/IG = 0.70) indicates that the carbon atoms in CQDs were sp2 graphitic carbon atoms in amorphous carbon.46
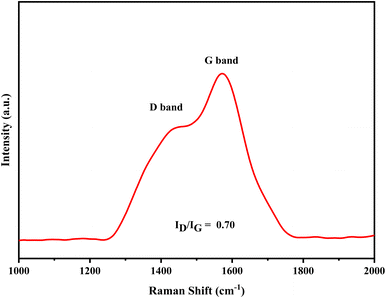 |
| Fig. 10 Raman spectrum of CQDs. | |
Carboxylic functionalities are clearly present on the surface of CQDs according to FTIR and XPS studies. Moreover, a few tests were performed to further confirmation of the –COOH sites on the surface of the CQDs. CQDs aqueous solutions have a pH of 4.2; this confirms their acidic nature. The addition of HCl completely protonated water soluble CQDs, which were then titrated with NaOH (see ESI, Fig. S1†). The pH range between 6.5 and 7 revealed the minimum slope of the curve, which corresponds to the highest buffering ability of CQDs, indicating the presence of several acidic functional groups (0.0015 N).47 Additionally, conductometric titration was used to examine the relative proportion of carboxylic groups. The volume of the NaOH solution was plotted against each conductivity value (see ESI, Fig. S2†). Two breaking points on the titration curves of CQDs were discovered by the convergence of three straight lines. The concentration of carboxylic in the CQDs sample was determined to be 0.0016 N using the consumed NaOH between two breaking points.48
Catalytic activity for the assessment of α,β-unsaturated compound
We first investigated the catalytic efficiency of the synthesized CQDs to produce α,β-unsaturated compounds. In a typical experiment, 2.0 mmol of the substrates with 5.0 mg ml−1 of catalyst was taken in a round bottle flask. We used 400 W power of microwave irradiation at 80 °C temperature under an aqueous solution of the reaction mixture. The conversion was not progressed without CQDs. Consequently, various metal-free materials were employed as a catalyst for a sustainable point of view and to enhance the efficiency of the protocol. The desired product was attained in a lower yield when the GO (10 wt%) or rGO (10 wt%) were used as a catalyst. The most appealing outcome was obtained with CQDs as the catalyst. The yield of the obtained compound depended on the concentration of the catalytic amount. It was concluded that a 5 mg ml−1 concentration was suitable for this conversion; the excess amount of CQDs did not alter the yield of the product. 1 mg ml−1 CQDs have a poor impact on the reaction (Table 1).
Table 1 Examination of reaction conditionsa
S. no. |
Catalyst |
Solvent |
Time |
Yieldb (%) |
Reaction conditions: 4-chlorobenzaldehyde (2.0 mmol) and phenylacetylene (2.0 mmol) as the model substrates; water as the solvent, 5 mg ml−1 CQDs as catalyst illuminated under microwave environment. Isolated yield. In conventional condition. |
1 |
— |
H2O |
30 min |
NR |
2 |
GO |
H2O |
30 min |
32 |
3 |
rGO |
H2O |
30 min |
26 |
5 |
g-C3N4 |
H2O |
30 min |
21 |
6 |
MWCNTs |
H2O |
30 min |
29 |
7 |
Graphite |
H2O |
30 min |
15 |
8 |
CQDs (5 mg ml−1) |
H2O |
10 min |
88 |
9 |
CQDs (3 mg ml−1) |
H2O |
10 min |
70 |
10 |
CQDs (8 mg ml−1) |
H2O |
10 min |
88 |
11 |
CQDs (1 mg ml−1) |
H2O |
10 min |
54 |
12 |
CQDs (5 mg ml−1) |
Tween-18 |
10 min |
24 |
13 |
CQDs (5 mg ml−1) |
EtOH |
10 min |
59 |
14 |
CQDs (5 mg ml−1) |
Ethylene glycol |
10 min |
42 |
15 |
CQDs (5 mg ml−1) |
Glycerol |
10 min |
37 |
16 |
CQDs (5 mg ml−1) |
DMSO |
10 min |
31 |
17 |
CQDs (5 mg ml−1) |
CH2Cl2 |
10 min |
22 |
18 |
CQDs (5 mg ml−1)c |
H2O |
24 h |
56 |
19 |
CQDs 5 (mg ml−1) |
EtOH/H2O (2 : 1) |
10 min |
63 |
20 |
CQDs 5 (mg ml−1) |
EtOH/H2O (1 : 1) |
10 min |
69 |
21 |
CQDs 5 (mg ml−1) |
EtOH/H2O (1 : 2) |
10 min |
74 |
To validate which functionality is responsible for this approach, CQDs were treated with 0.01 M NaOH. Due to the deprotonation, the carboxylic intensity of CQDs of the carboxylic group in the FT-IR spectra was decreasing (bCQDs) (see ESI, Fig. S3†). The carboxylic intensity of CQDs was restored after the treatment of bCQDs with 0.01 M HCl (baCQDs) (see ESI, Fig. S3†). Base treated CQDs were employed as a catalyst in the reaction system. bCQDs in the reaction system resulted in a low yield of the isolated compound even after a long reaction time. Catalytic activity was restored using baCQDs as a catalyst. These results confirm the key role of the carboxylic functionalities in this approach. To satisfy the appropriate conditions, a control experiment was conducted using some general acid catalysts such as HCl, conc. H2SO4, and glacial acetic acid. While excellent yield was obtained in the presence of HCl and conc. H2SO4. Although some drawbacks are associated with this catalyst which is already discussed. Therefore CQDs are viable alternatives for acid-catalyzed reactions.
Various solvents like Tween-18, CH2Cl2, and DMSO were tested in the model reaction. Less amount of desired product was obtained in these solvents but a moderate yield was attained in alcoholic solvents. The best results were observed when the transformation was done in H2O (Table 1). Interestingly, the catalytic activity and reactivity of the synthesized catalyst are higher in the presence of microwave irradiations and it shows lower catalytic activity in traditional thermal conditions (Table 1). The control experiments were also carried out by changing microwave irradiations (300, 400, and 500 W) and temperature during the optimization of reaction conditions. The results indicate that the conversion with 400 W power output at 80 °C shows maximum yield under optimized reaction conditions. In addition, this approach was scaled up to 10 mmol to take advantage of the highly effective sustainable protocol.
Literature reveals that in the condensation of phenylacetylene with aromatic aldehyde, the required α,β-unsaturated compounds are accompanied by propargyl alcohol as the side product, and sometimes this derivative has been separated as the main product.49 There are no side products formed in the current protocol that gives α,β-unsaturated carbonyl compounds selectively (Fig. 11).
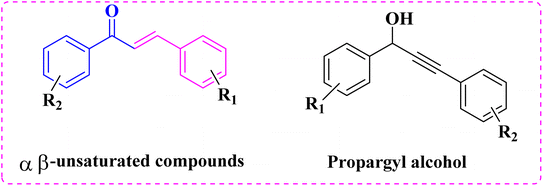 |
| Fig. 11 Possible products. | |
These admirable primary results fortified us to explore further the applicability of the protocol for the assessment of α,β-unsaturated compounds (Table 2). We have employed a variety of aromatic aldehyde to study the scope and confines of this process. As reviewed in Table 2, the aldehydes derivatives bearing both electron-withdrawing and electron-donating groups were evaluated with phenylacetylene derivatives and offered the required compounds with good to outstanding yields. Next, various functionalized alkenes were also tested for the generality and scope of the current methodology. Good functional group tolerance was noted with several alkynes.
Reaction conditions: aromatic aldehydes (2.0 mmol), and various phenylacetylene (2.0 mmol) in 10 ml aqueous solution CQDs (5 mg ml−1) under microwave irradiation. |
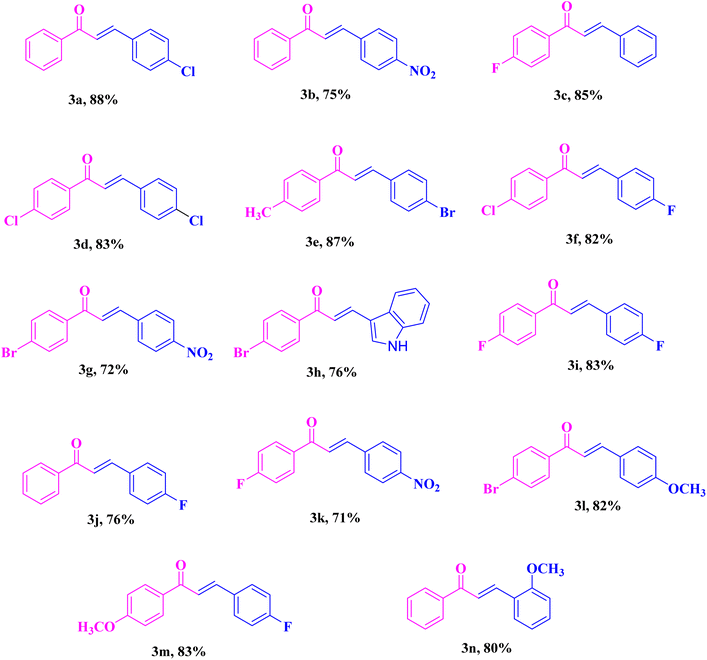 |
On the grounds of optimization outcomes and earlier reports on acid catalyzed routes,31,50 the following plausible mechanistic pathway is suggested (Scheme 2). The carboxylic group (–COOH) of CQDs facilitates the acid catalyzed hydration of phenylacetylene and produces ketone51 (ESI, Scheme SI†). The –COOH group of CQDs activated the aromatic aldehyde nucleophilic attack of the enol form of acetophenone and produced the β-hydroxy ketone intermediate (X) via Claisen–Schmidt condensation.52 Dehydration of intermediate X forced by the acidic sites of CQDs formed the desired result product. In general, the synthesis of α,β-unsaturated compounds accomplished by one pot hydration/condensation sequence of alkynes with aldehydes.
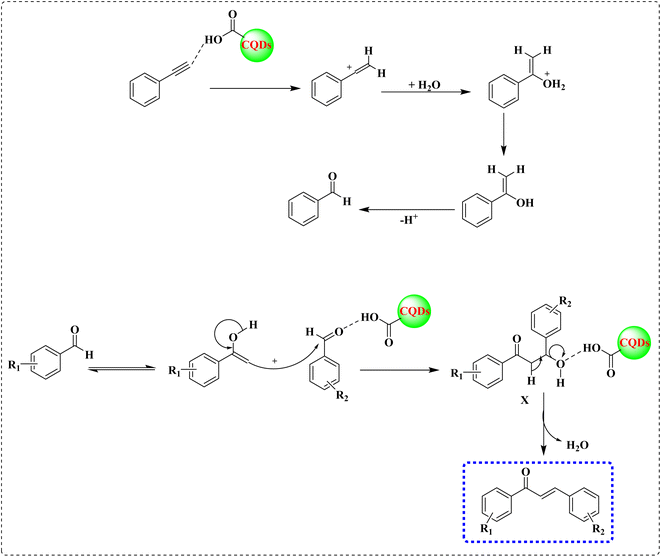 |
| Scheme 2 Plausible mechanism for the access of α,β-unsaturated compound. | |
Recyclability test
From a sustainable development and industrial point of view, the recovery and recycling of carbocatalysts are the primary characteristics. 4-Chlorobenzaldehyde (2.0 mmol) and phenylacetylene (2.0 mmol) under optimized conditions were chosen as a modal reaction to investigate the recyclability of the CQDs.
After the conversion was complete, the target product was removed with ethyl acetate. The aqueous layer containing CQDs was employed for the next reaction cycle. The results estimated that the activity of the catalyst remains approximately unchanged for 5 runs (Fig. 12). The FT-IR spectrum of the recycled catalyst indicated an unchanged structure of the CQDs. Similarly, TEM images of the recovered catalysts show the constant morphology of the catalyst even after five cycles.
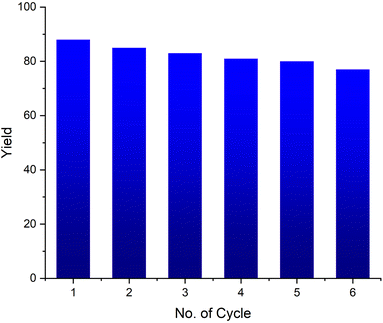 |
| Fig. 12 Recyclability of CQDs. | |
Conclusion
In this work, a simple environmentally friendly method for the synthesis of fluorescent CQDs using the mango kernel as a renewable green resource is described. The CQDs were prepared without using any acidic or alkaline materials. These fluorescent CQDs were characterized via several techniques, including XRD, TEM, EDAX, XPS, Raman, UV-vis, FT-IR, and PL analysis. The as-prepared CQDs have good water-solubility and strong greenish luminescence. The occurrence of free carboxylic groups on the surface of CQDs is recognized. Owing to the occurrence of free acidic groups, the as synthesized CQDs shows admirable catalytic activity for the synthesis α,β-unsaturated compounds via one pot hydration/condensation reaction of alkynes with aldehydes. Under microwave radiation the catalytic activity of CQDs was superior as compared to the conventional method. The selectivity of the protocol was very high; no other side product was obtained in this method. Mild reaction environment, easy workup procedure, good yield, reusable catalyst, shorter reaction time, and use of green solvent are the same advantage of the protocol.
Author contributions
Surendra Saini: methodology, investigation, writing – first draft, conducting a research and investigation process, Krishan Kumar: methodology, investigation, Pratibha Saini: methodology, investigation, data/evidence collection, conducting a research and investigation process, Dinesh Kumar Mahawar: methodology, Kuldeep S. Rathore: formal analysis, data curation, Sanjay Kumar: data curation, Anshu Dandia: development or design of methodology; creation of models, Vijay Parewa: conceptualization, methodology, writing – original draft, development or design of methodology; creation of models, preparation, creation, and/or presentation of the published work.
Conflicts of interest
The authors declare that they have no competing interests.
Acknowledgements
We are thankful to UGC and CSIR, New Delhi for the economic support and the MNIT, Jaipur, for spectral investigations.
References
-
(a) M. Rueping, T. Bootwicha, H. Baars and E. Sugiono, Beilstein J. Org. Chem., 2011, 7, 1680–1687 CrossRef CAS PubMed;
(b) N. Cancio, A. R. Costantino, G. F. Silbestri and M. T. Pereyra, Multidisciplinary Digital Publishing Institute Proceedings, 2019, 41, p. 13 Search PubMed.
- B. A. Bhat, K. L. Dhar, S. C. Puri, A. K. Saxena, M. Shanmugavel and G. N. Qazi, Bioorg. Med. Chem. Lett., 2005, 15, 3177–3180 CrossRef CAS PubMed.
- J. Song and M. Hesse, Tetrahedron, 1993, 49, 6797–6804 CrossRef CAS.
- U. Marquardt, D. Schmid and G. Jung, Synlett, 2000, 1131–1132 CAS.
- R. K. Callow and N. C. Johnston, Bee World, 1960, 41, 152–153 CrossRef CAS.
- H. Cho, M. Ueda, M. Tamaoka, M. Hamaguchi, K. Aisaka, Y. Kiso and T. Tatsuoka, J. Med. Chem., 1991, 34, 1503–1505 CrossRef CAS PubMed.
- C. T. Hsieh, T. J. Hsieh, M. El-Shazly, D. W. Chuang, Y. H. Tsai, C. T. Yen and F. R. Chang, Bioorg. Med. Chem. Lett., 2012, 22, 3912–3915 CrossRef CAS PubMed.
- D. Septianingtyas, N. Zafira, Zulhipri, F. Kurniadewi and H. Dianhar, AIP Conf. Proc., 2021, 2331, 040020 CrossRef CAS.
- W. Wei, W. Qunrong, D. Liqin, Z. Aiqing and W. Duoyuan, Ultrason. Sonochem., 2005, 12, 411414 CrossRef PubMed.
- S. Saravanamurugan, M. Palanichamy, B. Arabindoo and V. Murugesan, Catal. Commun., 2005, 6, 399–403 CrossRef CAS.
- J. B. Daskiewicz, G. Comte, D. Barron, A. Di Pietro and F. Thomasson, Tetrahedron Lett., 1999, 40, 7095–7098 CrossRef CAS.
- S. R. Sarda, W. N. Jadhav, S. R. Bhusare, S. K. Wasmatkar, S. A. Dake and R. P. Pawar, Int. J. ChemTech Res., 2009, 1, 265–269 CAS.
- S. Sebti, A. Solhy, R. Tahir, S. Boulaajaj, J. A. Mayoral, J. M. Fraile and H. Oumimoun, Tetrahedron Lett., 2001, 42, 7953–7955 CrossRef CAS.
- M. J. Climent, A. Corma, S. Iborra and J. Primo, J. Catal., 1995, 151, 60–66 CrossRef CAS.
-
(a) M. J. Climent, H. Garcia, J. Primo and A. Corma, Catal. Lett., 1990, 4, 85–91 CrossRef CAS;
(b) S. Saravanamurugan, M. Palanichamy, B. Arabindoo and V. Murugesan, J. Mol. Catal. A: Chem., 2004, 218, 101–106 CrossRef CAS.
- R. S. Varma, G. W. Kabalka, L. T. Evans and R. M. Pagni, Synth. Commun., 1985, 15, 279–284 CrossRef CAS.
-
(a) A. Fuentes, J. M. Marinas and J. V. Sinisterra, Tetrahedron Lett., 1987, 28, 4541–4544 CrossRef CAS;
(b) A. Aguilera, A. R. Alcantara, J. M. Marinas and J. V. Sinisterra, Can. J. Chem., 1987, 65, 1165–1171 CrossRef CAS.
- M. T. Drexler and M. D. Amiridis, J. Catal., 2003, 214, 136–145 CrossRef CAS.
-
(a) M. J. Climent, A. Corma, S. Iborra and A. Velty, J. Catal., 2004, 221, 474–482 CrossRef CAS;
(b) A. Guida, M. H. Lhouty, D. Tichit, F. Figueras and P. Geneste, Appl. Catal., A, 1997, 164, 251–264 CrossRef CAS.
-
(a) S. Sebti, A. Saber, A. Rhihil, R. Nazih and R. Tahir, Appl. Catal., A, 2001, 206, 217–220 CrossRef CAS;
(b) S. Sebti, A. Solhy, R. Tahir, S. Abdelatif, S. Boulaajaj, J. A. Mayoral and H. Oumimoun, J. Catal., 2003, 213, 1–6 CrossRef CAS;
(c) D. J. Macquarrie, R. Nazih and S. Sebti, Green Chem., 2002, 4, 56–59 RSC.
-
(a) X. Wang and S. Cheng, Catal. Commun., 2006, 7, 689–695 CrossRef CAS;
(b) X. Wang, K. S. Lin, J. C. Chan and S. Cheng, J. Phys. Chem. B, 2005, 109, 1763–1769 CrossRef CAS PubMed.
- Y. Zhao and Q. Song, Org. Chem. Front., 2006, 3, 294–297 RSC.
- Y. Masuyama, W. Takamura and N. Suzuki, Eur. J. Org. Chem., 2013, 2013, 8033–8038 CrossRef CAS.
- K. Miura, K. Yamamoto, A. Yamanobe, K. Ito, H. Kinoshita, J. Ichikawa and A. Hosomi, Chem. Lett., 2010, 39, 766–767 CrossRef CAS.
- D. A. Engel and G. B. Dudley, Org. Lett., 2006, 8, 4027–4029 CrossRef CAS PubMed.
- A. Singh, N. Kaur, N. Singh and D. O. Jang, Mol. Catal., 2017, 439, 100–107 CrossRef.
- K. S. Egorova and V. P. Ananikov, Angew. Chem., Int. Ed., 2016, 55, 12150–21162 CrossRef CAS PubMed.
- V. Parewa, A. Dandia, S. Kumari, S. Bansal and A. Sharma, Green Chem., 2016, 18, 2488–2499 RSC.
- S. Verma, H. P. Mungse, N. Kumar, S. Choudhary, S. L. Jain, B. Sain and O. P. Khatri, Chem. Commun., 2011, 47, 12673–12675 RSC.
- C. Zhuang, W. Zhang, C. Sheng, W. Zhang, C. Xing and Z. Miao, Chem. Rev., 2017, 117, 7762–7810 CrossRef CAS PubMed.
-
(a) S. M. Islam, A. S. Roy, R. C. Dey and S. Paul, J. Mol. Catal. A: Chem., 2014, 394, 66–73 CrossRef CAS;
(b) H. P. Jia, D. R. Dreyer and C. W. Bielawski, Adv. Synth. Catal., 2011, 353, 528–532 CrossRef CAS.
-
(a) N. Sharma, G. S. Das and K. Yun, Appl. Microbiol. Biotechnol., 2020, 104, 7187–7200 CrossRef CAS PubMed;
(b) J. Wei, X. Zhang, Y. Sheng, J. Shen, P. Huang, S. Guo and B. Feng, New J. Chem., 2014, 38, 906–909 RSC;
(c) M. Gholinejad, M. Seyedhamzeh, M. Razeghi, C. Najera and M. Kompany-Zareh, ChemCatChem, 2016, 8, 441–447 CrossRef CAS;
(d) D. Dey, T. Bhattacharya, B. Majumdar, S. Mandani, B. Sharma and T. K. Sarma, Dalton Trans., 2013, 42, 13821–13825 RSC.
-
(a) V. Parewa, P. Saini, M. Sethi, K. Kumar, S. Saini, S. Meena and A. Dandia, Catal. Rev., 2021, 1–55 Search PubMed;
(b) M. G. Álvarez, D. G. Crivoi, F. Medina and D. Tichit, Chem. Eng., 2019, 3, 29 Search PubMed;
(c) X. Y. Jiao, L. S. Li, S. Qin, Y. Zhang, K. Huang and L. Xu, Colloids Surf., A, 2019, 577, 306–314 CrossRef CAS;
(d) J. Zhou, Z. Sheng, H. Han, M. Zou and C. Li, Mater. Lett., 2012, 66, 222–224 CrossRef CAS;
(e) N. Irmania, K. Dehvari, G. Gedda, P. J. Tseng and J. Y. Chang, J. Biomed. Mater. Res., Part B, 2020, 108, 1616–1625 CrossRef CAS PubMed;
(f) M. Gholinejad, M. Bahrami and C. Nájera, Mol. Catal., 2017, 433, 12–19 CrossRef CAS.
-
(a) C. Kang, Y. Huang, H. Yang, X. F. Yan and Z. P. Chen, Nanomaterials, 2020, 10, 2316 CrossRef CAS PubMed;
(b) X. Lin, M. Xiong, J. Zhang, C. He, X. Ma, H. Zhang and Q. Huang, Microchem. J., 2021, 160, 105604 CrossRef CAS;
(c) P. Namdari, B. Negahdari and A. Eatemadi, Biomed. Pharmacother., 2017, 87, 209 CrossRef CAS PubMed;
(d) H. Chen, J. M. Pina, Y. Hou and E. H. Sargent, Adv. Energy Mater., 2022, 12, 2100774 CrossRef CAS;
(e) D. L. Zhao and T. S. Chung, Water Res., 2018, 147, 43–49 CrossRef CAS PubMed;
(f) M. Gholinejad, F. Zareh and C. Najera, Appl. Organomet. Chem., 2018, 32, e3984 CrossRef;
(g) M. Gholinejad, J. Ahmadi, C. Nájera, M. Seyedhamzeh, F. Zareh and M. Kompany-Zareh, ChemCatChem, 2017, 9, 1442–1449 CrossRef CAS.
-
(a) A. Rezaei, L. Hadian-Dehkordi, H. Samadian, M. Jaymand, H. Targhan, A. Ramazani and B. Tian, Spectrochim. Acta, Part A, 2021, 246, 118964 CrossRef PubMed;
(b) B. Majumdar, S. Mandani, T. Bhattacharya, D. Sarma and T. K. Sarma, J. Org. Chem., 2017, 82, 2097–2106 CrossRef CAS PubMed;
(c) D. P. Narayanan, S. K. Cherikallinmel, S. Sankaran and B. N. Narayanan, J. Colloid Interface Sci., 2018, 520, 70–80 CrossRef CAS PubMed.
-
(a) V. Parewa, S. L. Gupta, P. Saini, R. Sharma, S. Meena and A. Dandia, Curr. Res. Green Sustainable Chem., 2020, 3, 100039 CrossRef;
(b) V. Parewa, D. K. Mahawar, R. Sharma, R. S. Badgoti, K. S. Rathore and A. Dandia, Appl. Organomet. Chem., 2019, 33, e5232 Search PubMed;
(c) V. Parewa, S. Parihar, P. Saini, K. S. Rathore and A. Dandia, Catal. Lett., 2019, 149, 3169–3175 CrossRef;
(d) V. Parewa, S. Parihar, R. Sharma, K. S. Rathore and A. Dandia, in Green Sustainable Process for Chemical and Environmental Engineering and Science, ed. Inamuddin, R. Boddula and A. M. Asiri, Elsevier, Netherlands, 2020, ch. 4, pp. 71–103 Search PubMed;
(e) V. Parewa, P. Saini, R. Sharma and A. Dandia, Curr. Res. Green Sustainable Chem., 2020, 3, 100031 CrossRef;
(f) M. Gholinejad, C. Najera, F. Hamed, M. Seyedhamzeh, M. Bahrami and M. Kompany-Zareh, Tetrahedron, 2017, 73, 5585–5592 CrossRef CAS.
-
(a) X. Y. Jiao, L. S. Li, S. Qin, Y. Zhang, K. Huang and L. Xu, Colloids Surf., A, 2019, 577, 306–314 CrossRef CAS;
(b) A. Tyagi, K. M. Tripathi, N. Singh, S. Choudhary and R. K. Gupta, RSC Adv., 2016, 6, 72423–72432 RSC;
(c) W. Lu, X. Qin, S. Liu, G. Chang, Y. Zhang, Y. Luo and X. Sun, Anal. Chem., 2012, 84, 5351–5357 CrossRef CAS PubMed;
(d) A. J. Permana, A. Haris, H. Setyawati and M. Z. Fahmi, J. Chem. Technol. Metall., 2017, 52, 1101–1104 CAS;
(e) X. Yue, T. Chun-Jing, H. Huang, S. Chao-Qun, Y. K. Zhang, Y. Qun-Feng and W. Ai-Jun, Chin. J. Anal. Chem., 2014, 42, 1252–1258 CrossRef.
- C. P. Khare, Indian medicinal plants: an illustrated dictionary, Springer Science & Business Media, Germany, 2008, pp. 1–900 Search PubMed.
- R. Purbia and S. Paria, Biosens. Bioelectron., 2016, 79, 467–475 CrossRef CAS PubMed.
-
(a) M. Sabet and K. Mahdavi, Appl. Surf. Sci., 2019, 463, 283–291 CrossRef CAS;
(b) R. Atchudan, T. N. J. I. Edison, M. Shanmugam, S. Perumal, T. Somanathan and Y. R. Lee, Phys. E, 2021, 126, 114417 CrossRef CAS;
(c) P. Zhao, Q. Zhang, J. Cao, C. Qian, J. Ye, S. Xu and Y. Li, Nanomaterials, 2022, 12, 1528 CrossRef CAS PubMed.
-
(a) J. Zhou, Z. Sheng, H. Han, M. Zou and C. Li, Mater. Lett., 2012, 66, 222–224 CrossRef CAS;
(b) K. Dehvari, K. Y. Liu, P. J. Tseng, G. Gedda, W. M. Girma and J. Y. Chang, J. Taiwan Inst. Chem. Eng., 2019, 95, 495–503 CrossRef CAS.
- N. Thongsai, N. Tanawannapong, J. Praneerad, S. Kladsomboon, P. Jaiyong and P. Paoprasert, Colloids Surf., A, 2019, 560, 278–287 CrossRef CAS.
-
(a) S. Sahu, B. Behera, T. K. Maiti and S. Mohapatra, Chem. Commun., 2012, 48, 8835–8837 RSC;
(b) C. Zhao, X. Li, C. Cheng and Y. Yang, Microchem. J., 2019, 147, 183–190 CrossRef CAS.
-
(a) H. Nie, M. Li, Q. Li, S. Liang, Y. Tan, L. Sheng, W. Shi and S. Zhang, Chem. Mater., 2014, 26, 3104–3112 CrossRef CAS;
(b) L. H. Zhu, W. Zhang and S. Yu, Nanoscale, 2013, 5, 1797–1802 RSC;
(c) X. Yang, Y. Zhuo, S. Zhu, Y. Luo, Y. Feng and Y. Dou, Biosens. Bioelectron., 2014, 60, 292–298 CrossRef CAS PubMed.
-
(a) L. Zhang, H. Wang, Q. Hu, X. Guo, L. Li, S. Shuang and C. Dong, Mikrochim. Acta, 2019, 186, 1–13 CrossRef;
(b) R. Liu, D. Wu, S. Liu, K. Koynov, W. Knoll and Q. Li, Angew. Chem., Int. Ed., 2009, 48, 4598–4601 CrossRef CAS PubMed;
(c) J. Zhou, Z. Sheng, H. Han, M. Zou and C. Li, Facile synthesis of fluorescent carbon dots using watermelon peel as a carbon source, Mater. Lett., 2012, 66, 222–224 CrossRef CAS.
- R. Qiang, S. Yang, K. Hou and J. Wang, New J. Chem., 2019, 43, 10826–10833 RSC.
- D. R. Kumar, R. Karthik, G. Dhakal, J. Lee and J. J. Shim, J. Clean. Prod., 2022, 374, 133731 CrossRef.
- L. Pérez-Álvarez, L. Ruiz-Rubio and J. L. Vilas-Vilela, J. Chem. Educ., 2018, 95, 1022–1028 CrossRef.
-
(a) M. K. Tiwari, L. Yadav, B. R. Kumar Shyamlal and S. Chaudhary, Asian J. Org. Chem., 2019, 8, 2257–2268 CrossRef CAS;
(b) J. Yuan, J. Wang, G. Zhang, C. Liu, X. Qi, Y. Lan and A. Lei, Chem. Commun., 2015, 51, 576579 Search PubMed.
- N. Mameda, S. Peraka, S. Kodumuri, D. Chevella, R. Banothu, V. Amrutham and N. Nama, RSC Adv., 2016, 6, 58137–58141 RSC.
- Y. Chiang, A. J. Kresge, M. Capponi and J. Wirz, Helv. Chim. Acta, 1986, 69, 1331–1332 CrossRef CAS.
-
(a) F. Dong, C. Jian, F. Zhenghao, G. Kai and L. Zuliang, Catal. Commun., 2008, 9, 1924–1927 CrossRef;
(b) C. G. Halpani and S. Mishra, Tetrahedron Lett., 2020, 61, 152175 CrossRef CAS;
(c) J. R. Patel, M. H. Malani and B. Z. Dholakiya, Res. Chem. Intermed., 2012, 38, 2371–2381 CrossRef CAS.
|
This journal is © The Royal Society of Chemistry 2022 |
Click here to see how this site uses Cookies. View our privacy policy here.