DOI:
10.1039/D2RA05183D
(Paper)
RSC Adv., 2022,
12, 30577-30587
Visible-light photoelectrocatalysis/H2O2 synergistic degradation of organic pollutants by a magnetic Fe3O4@SiO2@mesoporous TiO2 catalyst-loaded photoelectrode†
Received
18th August 2022
, Accepted 21st October 2022
First published on 26th October 2022
Abstract
In this paper, we describe a method for photoelectrocatalysis (PEC)/H2O2 synergistic degradation of organic pollutants with a magnetic Fe3O4@SiO2@mesoporous TiO2 (FST) photocatalyst-loaded electrode. At optimal conditions of pH 3.0, 2.25% H2O2, working electrode (fixed FST 30 mg) potential +0.6 V (vs. SCE), and 10 mg L−1 of all experimental pollutants, the FST PEC/H2O2 synergistic system exhibited high activity and stability for the removal of various organic pollutants under visible light with comparable degradation efficiencies, including MB (98.8%), rhodamine B (Rh B, 96.7%), methyl orange (MO, 97.7%), amoxicillin (AMX, 83.9%). Moreover, this system obtained TOC removal ratios of 83.5% (MB), 77.9% (Rh B), 80.2% (MO), 65.5% (AMX) within 8 min. The kinetic rate constants of the PEC/H2O2 synergistic system were nearly 53 and 1436 times higher than that of the PEC process and H2O2 photolysis under visible light, respectively. Furthermore, the main reactive oxidant species (˙OH, ˙O2−) were studied and enhanced mechanisms of the photocatalytic-electro-H2O2 coupling system were proposed. This work brings new insights to efficiently purify organic pollutants by PEC coupled with peroxide under solar light illumination.
1. Introduction
With advances in industrialization and rapid population growth, organic pollutants are frequently detected in water environments. They continue to be enriched in food chains and food webs, endangering ecological environments and human health.1 These organic pollutants cannot be completely eliminated by wastewater treatment processes, resulting in residues in groundwater and surface water. Therefore, it is necessary to develop suitable technological approaches for the decomposition and mineralization of refractory organic pollutants and removal of refractory organic pollutants from water environments.
Refractory organic pollutants cannot be effectively degraded and mineralized by using the conventional biological, physical and chemical wastewater treatment methods. Compared to these traditional technologies, advanced oxidation processes (AOPs) have many advantages with regards to degrading refractory organic pollutants, which include complete mineralization and oxidation of refractory organic pollutants, low secondary pollution outcomes, and small working area. AOPs such as heterogeneous catalysis,2 photoelectrocatalysis (PEC),3 photocatalysis,4 Fenton5 and Fenton-like,6 electro-Fenton and photoelectro-Fenton,7 electrochemical oxidation,8 etc. can be used for wastewater treatment. The PEC technology combines photocatalysis and electrochemical methods, which is an efficient process to remove recalcitrant organic pollutants from water.9 Biasing the semiconductor photoanode with a voltage under illumination, photogenerated h+–e− pairs can be effectively inhibited by an external electric field, resulting in improved photocatalytic activities.10 A lot of research has been done on the photoanode materials, reaction mechanisms and kinetics of PEC,11 which shows that it is a promising wastewater treatment technology.
TiO2 is considered as a promising photocatalyst for wastewater treatment12 due to its unique photoelectric performance and high photochemical stability, and it has been widely exploited as photoanode material in PEC systems.13 However, due to the large forbidden bandwidth of TiO2 (3.2 eV for anatase type), it can only absorb ultraviolet light (λ ≤ 387 nm), which limits its photocatalytic activity under visible light.14 In addition, photogenerated hole–electron pairs (h+–e−) of TiO2 can rapidly recombine, resulting in low catalytic efficiency.15 So there have been many attempts to modify titanium dioxide (e.g. dye sensitization, metal/non-metal doping, morphology control, and composite photocatalysts) to overcome aforesaid challenges.16 Among the composite photocatalysts, magnetic TiO2 is not only to be separated and recovered from reaction mixtures easily, but also contains some magnetic nanoparticles that have good visible-light absorption and excellent optical/electrical properties, further improving the degradation kinetics of pollutants.17 Due to its low toxicity, biocompatibility, and superparamagnetism, magnetite (Fe3O4) has become one of the most widely used magnetic nanoparticles18,19 in the fabrication of magnetic TiO2 photocatalysts. Previous studies have shown that direct contact of the magnetic core with photocatalysts leads to photodissolution of Fe3O4,20 as well as increases recombination of photogenerated charge carriers, thereby reducing the catalytic activity.21 It has been reported that the SiO2 interlayer between TiO2 and the magnetic core can effectively prevent the chemical- and photo-dissolution of Fe3O4
22,23 and can improve the photocatalytic activity of TiO2.24 And because of silica's well-known special characteristics, such as low cost, thermal and mechanical stability, surface chemical and adsorption capacity, and optical transparency in the wavelength region where TiO2 absorbs,25 previous studies have shown that the core–shell SiO2@TiO2 has excellent photocatalytic efficiency for the pollutant degradation than pure TiO2.26 In recent years, the research on magnetic TiO2 composite photocatalysts based on Fe3O4 has mostly focused on the application of Fe3O4@SiO2@TiO2 in photocatalysis.27–33 The role of Fe3O4 in these studies is primarily in the area of magnetic separation, but less attention is paid to how to improve TiO2 photocatalytic activity. Meanwhile, less research has been conducted on Fe3O4@SiO2@TiO2 in photoelectrocatalysis,34 and few studies have comprehensively discussed the issues of expanding the light absorption range, increasing the photoelectrocatalytic activity, effective charge separation performance, and stable recyclability.35 Therefore, it is necessary to further study the effect of Fe3O4 on the band gap and optical absorption of Fe3O4@SiO2@TiO2 composites, and on the optical and electrical properties of composite photoanodes. We successfully prepared a novel composite photoelectrocatalytic material (Fe3O4@SiO2@mesoporous TiO2, FST), encapsulated by layers of Fe3O4, SiO2 and mesoporous TiO2 particles with a unique core–shell structure. The dense SiO2 layer prevents the magnetic core from interacting with TiO2, reducing the electron–hole recombination rate, and preventing photo-dissolution of Fe3O4 (ref. 36) and dissolution under acidic conditions. Fe3O4 can lower the band gap of FST and enhance the visible-light absorption. Due to the mesoporous structure and anatase TiO2 particles, FST has a high specific surface area and exceptional catalytic performance.
Because of mass transfer limitations and the low number of active radicals generated in the heterogeneous PEC systems, the efficiency of photoelectrocatalytic oxidation for pollutant removal should be increased. UV/H2O2 systems have been applied to the degradation of various pollutants.37 The addition of H2O2 to other AOPs can improve the degradation efficiency of organic pollutants by strengthening the generation of radicals in a wider spectral range.38 It has been reported that H2O2 can enhance the generation of radicals and improve the degradation efficiency in PEC systems.39,40
In this study, a PEC/H2O2 coupling system with magnetic FST-loaded photoanode was constructed to discuss the synergistic degradation of organic pollutants. The FST PEC/H2O2 system was effective for the degradation of methylene blue, rhodamine B, methyl Orange, and amoxicillin under visible light. With the addition of 2.25% H2O2, the reaction kinetic rate constant of the PEC/H2O2 synergistic system was nearly 53 times that of individual PEC. In the PEC/H2O2 process, ˙O2− and ˙OH were generated to degrade pollutants, and at the same time, the photogenerated e− could be captured by a sufficient amount of H2O2 to generate ˙OH, which increased the amounts of strong oxidizing substances and promoted the separation of photogenerated h+–e−. The formed photocatalytic-electro-H2O2 coupling system improved the degradation efficiency of diverse organic pollutants, and maintained the high degradation efficiency within 8 degradation cycles.
2. Experimental section
2.1. Materials and reagents
Indium tin oxide (ITO) coated glass slides (25 mm × 25 mm × 1.1 mm, 8–12 Ω sq−1) were purchased from Sigma-Aldrich (Shanghai Warehouse). Methylene blue (MB, C16H18ClN3S, ≥98%), rhodamine B (Rh B, C28H31ClN2O3, ≥99%), methyl orange (MO, C14H14N3NaO3S, 98%), amoxicillin (AMX, C16H19N3O5S·3H2O, 98%), titanium butoxide (C16H36O4Ti, AR), poly(propylene glycol) (PPG 1000, H[OCH(CH3)CH2]nOH, average Mn ∼ 1000), tetraethyl orthosilicate (TEOS, C8H20O4Si, >99%), ethanol (CH3CH2OH, ≥99.5%) were purchased from Macklin Inc. (Shanghai, China). FeCl3·6H2O (AR), FeSO4·7H2O (AR), NH3 H2O (AR, 28%), HCl (AR, 36%), glacial acetic acid (CH3COOH, AR), and other inorganic metal salts were purchased from Sinopharm Chemical Reagent Co., Ltd (Shanghai, China). All solutions were prepared using ultrapure water from a Milli-Q water purification system (resistivity > 16 MΩ cm at 25 °C).
2.2. Fabrication of photoelectrode
The detailed synthesis of FST and TiO2 can be found from ESI.†
FST-loaded photoelectrode: 30 mg FST was sonicated in 1 mL ethanol to obtain a 30 mg mL−1 FST suspension. The ITO coated glass slides, which have been cleaned via ultrasonic waves in acetone, ethanol and distilled water, were connected to a section of electrode wires with conductive silver glue. The epoxy resin glue was used to seal the connection between ITO and the electrode wire. Then, 30 mg mL−1 FST suspension was applied on the ITO work surface and dried at 25 °C. During the PEC/H2O2 synergistic degradation experiment, the Nd–Fe–B (25 mm × 25 mm × 5 mm) magnet was coated with a layer of polyvinyl chloride film to avoid the contact of the Nd–Fe–B magnet with the reaction solution, and glued to the back of the ITO work surface.31
TiO2-loaded photoelectrode: 30 mg TiO2 was sonicated in 1 mL ethanol to obtain a 30 mg mL−1 TiO2 suspension. Add the TiO2 suspension dropwise to the cleaned ITO conductive surface and dry at 25 °C.
2.3. Characterization of FST
Sample microscopic morphologies were investigated by field emission transmission electron microscopy (JEM-2100F, JEOL, Japan). The X-ray diffraction (XRD) pattern was measured by a BrukerX-ray diffractometer (D8 advance, Germany). X-ray photoelectron spectroscopy (XPS, ThermoEscalab 250Xi, Thermo fisher, USA) was performed to assess surface properties and composition. The UV-vis diffuse reflectance spectrum was determined by UV-visible-near infrared light spectrophotometry (Cary 5000, Agilent, USA). Mesoporous structures of catalysts were measured using the surface area and pore size analyzer (Autosorb-iQ, Quantachrome, USA). Magnetic data was obtained by an MPMS magnetic measurement system (MPMS-VSM, Quantum Design, USA).
2.4. The PEC/H2O2 synergistic degradation experiment
Experiments were conducted in a quartz photoelectric reactor with the volume of 60 mL, and the light source was a xenon light source (H8X-F300, Beijing Newbit Technology Co., Ltd.). Potentiostat experiments were performed using a three electrode system consisting of a working electrode (W.E., FST + ITO photoelectrode, anode), a counter electrode (C.E., Pt electrode, cathode), and a reference electrode (R.E., saturated calomel electrode, recorded as SCE), which were controlled using a CHI electrochemical analyzer (CHI 760E, CH Instruments, Inc., USA). The distance between the working electrode and the light source was 10 cm. To enhance conductivity, 0.05 mol per L Na2SO4 was added to the pollutants solution (the concentration was 10 mg L−1) as the supporting electrolyte.
The UV-vis spectrophotometer (UV-5800, Shanghai) was used to determine pollutant concentrations (λmax,MB = 664 nm, λmax,Rh B = 554 nm, λmax,MO = 463 nm, λmax,AMX = 198 nm). The total organic carbon (TOC) was determined using a TOC analyzer (Elementer, vario TOC).
3. Results and discussion
3.1. Morphology and structural analysis of catalysts
The FST microspheres were characterized by scanning transmission electron microscopy (STEM). The high-angle annular dark-field (HAADF) image in Fig. 1a exhibited an overlay of Fe3O4 core and TiO2 shell with a clear separation. A clear contrast difference was also observed in EDS mappings (Fig. 1b), confirming the core–shell structure of FST. The diameter of Fe3O4 core was about 580 nm, while thickness of the SiO2 intermediate layer and TiO2 shell layer was about 45 nm and 50 nm, respectively.
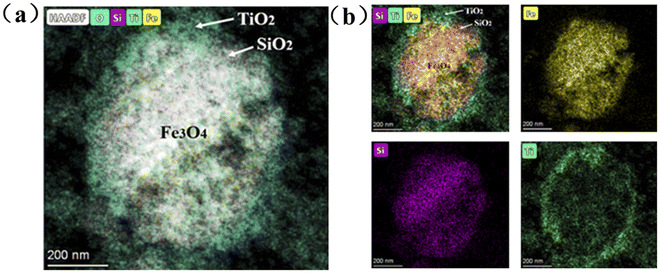 |
| Fig. 1 Scanning transmission electron microscopy (STEM) of FST: (a) HAADF image, (b) EDS mappings of Fe3O4, SiO2, and TiO2. | |
FST, Fe3O4@SiO2 and Fe3O4 nanoparticles were analyzed by X-ray diffraction (XRD) (Fig. 2). Fe3O4 nanoparticles exhibited characteristic peaks at 2θ = 30.1°, 35.44°, 43.2°, 53.56°, 57.1° and 62.6°, in agreement with previously reported XRD patterns of Fe3O4.41 The obtained material was Fe3O4 crystal with anregular octahedral inverse spinel structure. Fe3O4@SiO2 particles exhibited comparable XRD patterns to Fe3O4, indicating that the Fe3O4 crystal structure was well maintained. Characteristic peaks at 25.4°, 37.8°, 48.1°, 54.1°, 55.2°, 62.8° and 68.8° of FST can be attributed to anatase TiO2 (101), (004), (200), (105), (211), (204) and (116), respectively. The high crystallinity of nano-TiO2 can reduce the migration distance of photogenerated charges, and accordingly reduce the recombination rate.42
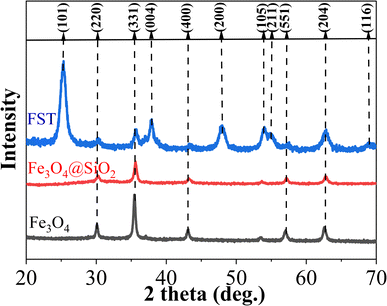 |
| Fig. 2 X-ray diffraction (XRD) pattern. | |
The characterization data and analysis details of mesoporous structure, magnetic response and XPS spectrum were described in the ESI.†
3.2. Photo-electric performance
The UV-vis diffuse reflectance spectra (DRS) of Fe3O4, Fe3O4@SiO2, TiO2 nanoparticles and FST were shown in Fig. 3a. TiO2 exhibited a lower optical absorption response from 400–1000 nm. Comparing the optical absorption responses of Fe3O4, Fe3O4@SiO2 and FST at 400–1000 nm, Fe3O4 has the strongest light absorption, while FST has a weaker light absorption due to the encapsulation of SiO2 and TiO2. However, the visible-NIR absorption of FST is still significantly enhanced compared with that of TiO2. Since FST is very sensitive to visible light, it enhances the performance of visible-light driven photoelectrocatalytic systems. Using the Tauc equation (eqn (1)) and the Kubelka–Munk function (eqn (2)), band gaps of Fe3O4, Fe3O4@SiO2, TiO2 and FST were calculated as previously reported.43 |
[F(R)hʋ]0.5 = A(hʋ − Eg)
| (1) |
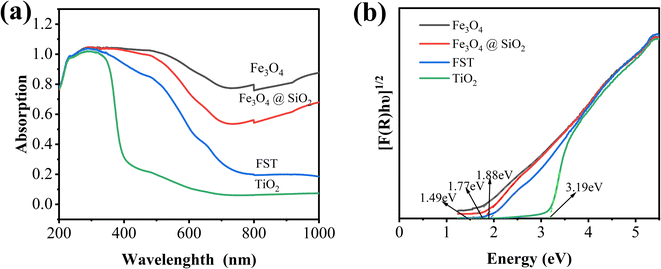 |
| Fig. 3 (a) UV-vis diffuse reflectance spectrum (DRS), (b) Tauc plot of DRS. | |
Fig. 3b shows that the effective Eg values of samples were calculated by plotting [F(R)hʋ]0.5 versus hʋ. The indirect Eg values of catalysts can be determined by extrapolating the linear part at [F(R)hʋ]0.5 = 0. The Eg values for Fe3O4, Fe3O4@SiO2, FST and TiO2 were 1.49, 1.77, 1.88 and 3.19 eV, respectively. The addition of Fe3O4 and SiO2 reduced the forbidden bandwidth of TiO2, so that the light absorption of FST extended to the visible light region. The higher photocatalytic performance at higher wavelength has shown the capability to potentially utilize natural solar light as activation source.29 Compared with other materials (WO3, ZnO, CdS, etc.) with low Eg and activity in visible light, FST has lower Eg, and has the characteristics of nontoxicity, biochemical inertness, low cost of TiO2.
To investigate the charge transfer of interface between photocatalysts and reaction solutions, Electrochemical Impedance Spectroscopy (EIS) was conducted. The EIS Nyquist plots were shown in Fig. 4a. The circular radius of ITO + FST was smaller than the pristine ITO and ITO + TiO2. A smaller impedance arc radius at low frequencies signifies a lower charge transfer resistance (Rct),44 thus, h+–e− pairs are efficiently separated. In this regard, ITO + FST possessed faster charge transfer rate and more efficient separation of h+–e− pairs than ITO and ITO + TiO2 at the solid–liquid interface.
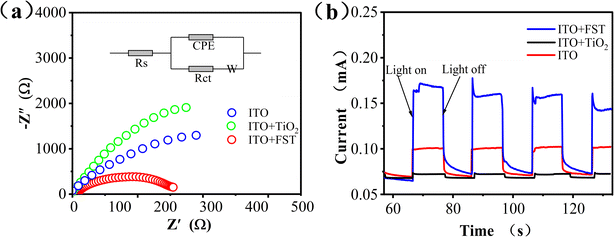 |
| Fig. 4 (a) EIS responses of ITO, ITO + TiO2 and ITO + FST, (b) surface photocurrent curves. | |
The photocurrent of W.E. at +0.6 V (vs. SCE) was measured to test the separation efficiency of photogenerated charge by several on/off cycles of visible-light. Photocurrent response curves of pristine ITO, ITO + TiO2 and ITO + FST were shown in Fig. 4b. The photocurrents generated by ITO + FST, ITO and ITO + TiO2 photoelectrodes were 0.17, 0.10, and 0.07 mA, respectively. The photocurrent of the ITO + FST electrode was about 1.7-fold that of ITO electrode, and 2.4-fold that of ITO + TiO2 electrode, respectively. The photocurrent enhancement of ITO + FST induced by visible light indicates that the photogenerated h+–e− pairs at the interface have higher separation efficiency and less recombination. The high separation rate of h+–e− pairs can promote the movement and subsequent reaction of electrons. However, TiO2 had lower conductivity than ITO, and absorbed less visible-light, so the photocurrent of the ITO + TiO2 was lower than that of the pristine ITO.
3.3. Control experiments for different degradation methods
Taking MB as the model pollutant, the effects of direct photolysis (PO), photocatalysis (PC), electrocatalysis (EC), photoelectrocatalysis (PEC), and PEC/H2O2 synergistic system on MB degradation were compared (Fig. 5). In the PO, PC, PEC, and PEC/H2O2 synergistic system, illumination was the visible light provided by the xenon lamp light source. In the EC, PEC, and PEC/H2O2 synergistic system, anode potential was +0.6 V (vs. SCE). As shown in curve (a), the MB solution showed less than 1.9% degradation when irradiated with visible light in the H2O2 PO for 40 min. Curve (b) showed that the degradation efficiency was slightly increased up to about 25% by visible-light PC after adding FST to the MB solution. Curve (c) represented the PC in the presence of H2O2, the degradation efficiency was significantly improved, and 31.1% of the MB was removed after 40 min. Curve (d–f) showed the experimental results under EC conditions. MB exhibited stability, whether H2O2 (curve (d)) or FST (curve (e)) are added alone. After adding H2O2 and FST at the same time (curve (f)), the removal rate slightly increased, while the degradation efficiency was less than 10% after 40 min. Curves (g) and (h) represented experimental results in PEC. Under these conditions, the removal rate of MB reached 61.5% after adding only FST for 40 min, the removal rate of MB reached 96.1% after adding only H2O2 for 10 min, and was close to 100% after 20 min. Curve (i) showed the experimental results under PEC/H2O2 synergistic degradation conditions. FST and H2O2 significantly improved MB degradation when they coexisted, the degradation efficiency was close to 100% after 5 min. Therefore, FST PEC has good synergistic effects with H2O2 PO and can quickly remove pollutants.
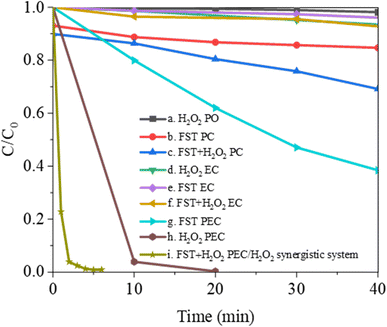 |
| Fig. 5 MB degradation by photolysis (PO), photocatalysis (PC), electrocatalysis (EC), photoelectrocatalysis (PEC), and PEC/H2O2 synergistic system. | |
The synergy factor (S) obtained from eqn (3)
45 elucidates on the synergy between PEC and H2O2 photolysis in the PEC/H2O2 degradation system, and demonstrates the priority of the combined process.
where,
K1,
K2, and
K12 are kinetic constants for H
2O
2 PO, PEC process, and PEC/H
2O
2 synergistic system, respectively. Experimental data was fitted with the Langmuir–Hinshelwood model, and kinetic constants (
Table 1) of the different degradation methods presented by the different curves (a, g, i) in
Fig. 5 were calculated. Since the concentration of MB was low, it was assumed that the reaction would take place with a pseudo-first-order equation. The correlation coefficient (
R2) for all processes was above 0.95, indicating that the assumption was reasonable. Based on
Table 1, the kinetic constant
K of the FST PEC/H
2O
2 synergic system (1.2924 min
−1) was found to be about 1436 and 53 times higher than that of the H
2O
2 PO (0.0009 min
−1) and FST PEC (0.0244 min
−1), respectively. Substituting
K1,
K2 and
K12 into
eqn (3), the value of
S was 51.08 (much greater than 1), indicating that the composite process was not a simple addition of PEC and H
2O
2 photolysis.
Table 1 Kinetic constants and correlation coefficients (R2) of MB degradation
Curve in Fig. 5 |
Degradation system |
Kinetic constant (min−1) |
R2 |
a |
H2O2 PO |
0.0009 (K1) |
0.9620 |
g |
FST PEC |
0.0244 (K2) |
0.9979 |
i |
FST + H2O2 PEC/H2O2 synergistic system |
1.2924 (K12) |
0.9562 |
3.4. Optimization of FST PEC/H2O2 synergistic system
Using methylene blue (MB) as the model pollutant, the experimental conditions and degradation performance of the PEC/H2O2 synergistic system was studied.
Effects of pH. To study the effects of the pH on MB degradation efficiency, the initial pH of the reaction solution, including 10 mg L−1 MB, 0.05 mol per L Na2SO4, and 2.25% H2O2, was adjusted to 1.0, 2.0, 3.0, 4.0, and 5.0, respectively, and the ITO + FST working electrode (fixed FST 30 mg) was applied at +0.6 V (vs. SCE) potential, after which degradation was performed by visible-light irradiation. MB degradation rates under different degradation times in each group were compared and analyzed (Fig. 6a). When pH was in the range of 1.0–5.0, the degradation efficiency of MB reached 99% after 6 min. At the pH range of 1.0–3.0, the degradation efficiency was more than 80% after 1 min, and reduced to less than 80% at the pH range of 4.0–5.0, indicating that lower pH value is more conducive for improving photocatalytic activities.46 The degradation efficiency was largest at pH 1.0, but its advantages relative to pH 2.0–3.0 were not marked. Therefore, pH 3.0 was selected as the optimal reaction pH value.
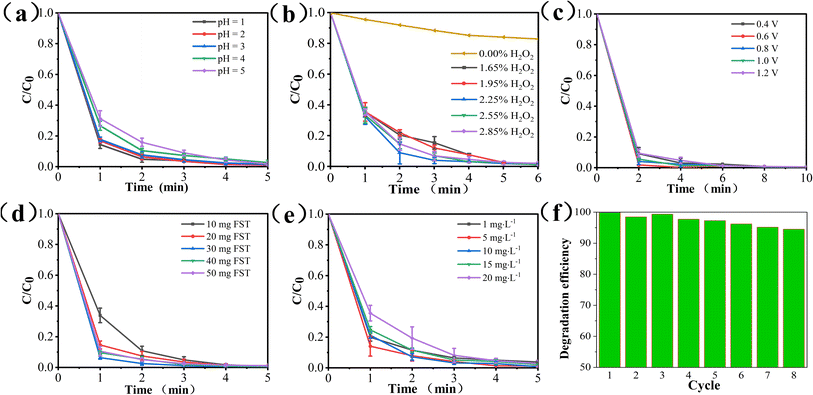 |
| Fig. 6 PEC/H2O2 synergistic degradation of MB. (a) pH values, (b) H2O2 concentration, (c) anodic potential, (d) FST fixation amount, (e) initial mass concentration of MB, (f) recycling test of FST. | |
Effects of H2O2 concentrations. To determine the impact of H2O2 concentrations on PEC/H2O2 synergistic degradation, the ITO + FST working electrode (fixed FST 30 mg) was applied at a bias voltage of +0.6 V (vs. SCE). The solution in the photocell contained 10 mg L−1 MB, 0.05 mol per L Na2SO4, and H2O2 with concentrations of 0.00%, 1.65%, 1.95%, 2.25%, 2.55% and 2.85%, respectively. Finally, the solution was adjusted to pH value of 3.0. Degradation efficiency of MB at different degradation times under each group were compared and analyzed (Fig. 6b). The amount of H2O2 was established to be an important factor in MB degradation. When H2O2 concentration was 2.25%, degradation efficiency was highest within 1 to 3 min. H2O2 is beneficial for photocatalytic reaction by trapping the conduction band e− in order to generate more ˙OH radicals.47 However, when the concentration of H2O2 was higher than 2.25%, the degradation efficiency of MB didn't continue to increase (2.55% H2O2), but decreased (2.85% H2O2). Excess H2O2 consumes ˙OH, the main active species for degrading MB, and H2O2 reacts with ˙OH to generate ˙HO2 or ˙O2− with much less reactivity,48 which reduces the catalytic reaction rate and is unfavorable for MB degradation. Therefore, the optimal concentration of H2O2 in this system is 2.25%.
Effects of applied anodic potential. To examine the effects of various W.E. anodic potentials on degradation of MB, 10 mg L−1 MB, 0.05 mol per L Na2SO4, and 2.25% H2O2 were added to the photoelectric reaction cell, the solution pH was adjusted to 3.0, and ITO + FST W.E. (fixed FST 30 mg) was applied at potentials of +0.4 V, +0.6 V, +0.8 V, +1.0 V, and +1.2 V (vs. SCE). As shown in Fig. 6c, MB rapidly degraded with increasing potential at the potential range of +0.4–+0.6 V (vs. SCE). At the potential of +0.6 V (vs. SCE), the degradation efficiency of MB was 98.25% within 2 min. The bias voltage applied on W.E. makes the visible-light photogenerated electrons to flow to the C.E. along the external circuit, thereby reacting with H2O2 to generate ˙OH.49 However, as the potential continued to rise, degradation efficiency of MB decreased, because too high potential caused the electropolymerization of MB50 and the oxidation of H2O2. H2O2 had an oxidation peak at +0.6 V (vs. SCE) (Fig. S4†). If the potential exceeded + 0.6 V, the H2O2 added in the system was consumed by oxidation, which reduced the MB's degradation efficiency. Thus, anode potential +0.6 V (vs. SCE) is the optimal potential of the system.
Effects of FST loading amounts. The fixed amount of FST catalyst on the working electrode directly affects the degradation efficiency of MB. As displayed in Fig. 6d, the effects of FST loading amounts on MB degradation, where the conditions included 10 mg L−1 MB, 0.05 mol per L Na2SO4, 2.25% H2O2, pH 3.0, +0.6 V (vs. SCE) to the W.E. and different FST fixed quantities (10, 20, 30, 40, and 50 mg). When the fixed amounts of FST were 10–30 mg, the degradation efficiency of MB increased with increasing fixed amounts of catalysts. When the fixed amount of catalysts was 30 mg, the MB degradation efficiency was maximum, while the degradation efficiency of MB gradually decreased at >30 mg. Too much catalysts negatively affect the degradation efficiency, while agglomeration between catalyst particles reduces the number of active sites.51 Besides, too much FST increases the thickness of the catalyst layer on the surface of ITO, which affects photoanode conductivity and visible light transmittance. Thus, the optimal fixed amount of FST is determined to be 30 mg.
Effects of MB initial mass concentrations. The effects of MB initial mass concentrations (1, 5, 10, 15, 20 mg L−1) on degradation rates were evaluated. The system contained 0.05 mol per L Na2SO4, 2.25% H2O2, pH 3.0, the W.E. (fixed FST 30 mg) applied at the potential of +0.6 V (vs. SCE). The effects of initial mass concentration of MB solution on degradation were shown in Fig. 6e. The degradation efficiencies of five different concentrations of MB by FST PEC/H2O2 system were above 90%, with 10 mg L−1 exhibiting the best degradation effect.
Recyclability performance of FST. The PEC/H2O2 degradation experiment was performed at optimal conditions of 10 mg L−1 MB, 0.05 mol per L Na2SO4, 2.25% H2O2, pH 3.0, and +0.6 V (vs. SCE) potential to the W.E. (fixed FST 30 mg). After W.E. was vacuum-dried at 80 °C, the above degradation experiment was repeated 8 times, and then the degradation efficiency of MB measured each time. As can be seen from Fig. 6f, the MB degradation efficiency were above 98% within 5 recycled and reused experiments, and the degradation efficiency only showed a slight decrease in 6–8 degradation cycles, demonstrating that FST catalysts were recyclable and stable. Comparable findings have been reported, where such magnetically attached FST working electrode had the desired stability.33 The magnetic FST was loaded on the surface of ITO working electrode by attractive forces of the magnet, the FST was firmly fixed and had a good stability.
3.5. PEC/H2O2 synergistic degradation of pollutants
To further discussion the versatility of PEC/H2O2 synergistic system for the treatment of different organic pollutants, the degradation experiments of four kinds of pollutants (MB, Rh B, MO, AMX) were carried out. As displayed in Fig. 7, the maximum degradation efficiencies of MB, Rh B, MO, and AMX in the FST PEC/H2O2 synergistic system could reach up to 98.8%, 96.7%, 97.7% and 83.9% after 8 min illumination, respectively. Moreover, this system obtained TOC removal rate of 83.5% (MB), 77.9% (Rh B), 80.2% (MO), and 65.5% (AMX), indicating the incomplete mineralization of MB, Rh B, MO, and AMX in the PEC/H2O2 system within 8 min and the presence of intermediate products. In general, the FST PEC/H2O2 synergistic degradation system showed excellent oxidative degradation and mineralization abilities for MB, Rh B, MO, and AMX, indicating the wide applicability of PEC/H2O2 coupling system for organic pollutants wastewater treatment.
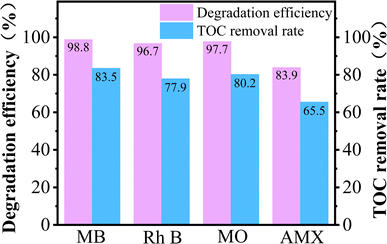 |
| Fig. 7 Degradation efficiency and TOC removal rate of MB, Rh B, MO, and AMX. | |
3.6. Degradation mechanism
The possible reaction mechanism of the FST PEC/H2O2 synergistic degradation system is shown in Fig. 8. When visible light irradiates FST, the energy (hν) of the incident photon is higher than the semiconductor band gap (Eg), and the electrons in the valence band (VB) are excited to transition into the conduction band (CB) to become highly active photogenerated electrons (e−); photogenerated positively charged holes (h+) stay in the VB, generating photogenerated h+–e− pairs. Upon separation, the e− and h+ move to the surface of the semiconductor. The h+ in the VB reacts with H2O to generate hydroxyl radicals (eqn (4)); the e− in the CB moves to the platinum counter electrode through an external circuit under the action of a bias voltage. Photogenerated e− reacts with adsorbed or dissolved O2 on the electrode surface to form ˙O2− (eqn (5)). In an acidic environment of the system, e− reacts with O2 to form H2O2 (eqn (6)),52,53 after which H2O2 reacts with ˙O2− to produce more ˙OH (eqn (7)).54 By adding H2O2 to the system, e− can directly activate H2O2 to generate a large number of ˙OH (eqn (8)).49 The ˙OH and ˙O2− in the system are involved in oxidative degradation of pollutants. |
H2O2 + ˙O2− → ˙OH + OH− + O2
| (7) |
|
H2O2 + e− → ˙OH + OH−
| (8) |
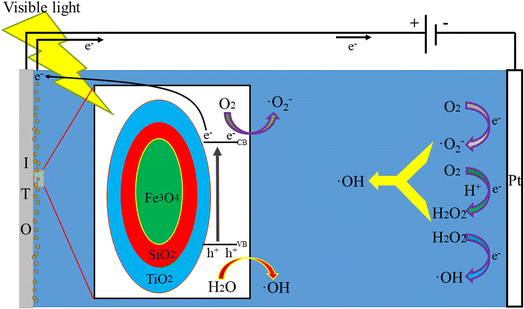 |
| Fig. 8 Reaction mechanism of FST PEC/H2O2 synergistic degradation system. | |
The free radical quenching experiment was performed to verify the generation and degradation mechanisms of active free radicals in the PEC/H2O2 synergistic degradation reaction. Tert-butanol (TBA), benzoquinone (BQ), ethylenediaminetetraacetic acid disodium salt (EDTA–2Na), and silver nitrate (AgNO3) were respectively added to the FST PEC/H2O2 degradation system for MB as quenchers, to scavenge for related reactive species, including hydroxyl radicals (˙OH), superoxide radicals (˙O2−), photogenerated holes (h+) and photogenerated electrons (e−).55,56 Fig. 9 displayed that addition of TBA, as a scavenger for ˙OH, significantly inhibited the MB degradation reaction, indicating that ˙OH was the main species in this system. From the eqn (4), (5), (6), (7) and (8), ˙OH is the most active substance produced by the system. The degradation efficiency of MB slightly decreased after adding BQ, suggesting that variations of ˙O2− had little effects on MB degradation. ˙O2− was only generated by eqn (5), and accounted for a small amount of active species. The addition of EDTA–2Na had no significant effects on MB degradation. Although EDTA–2Na captures h+ and reduces the ˙OH generated in eqn (4), the capture of h+ is more conducive to separating e− and h+, and the eqn (5), (6), (7) and (8) caused by photogenerated e− can still generate large amounts of hydroxyl radicals, which continue to degrade pollutants. When AgNO3 was added as an e− scavenger, MB undergoed a certain degradation efficiency change, indicating that e− played one significant role in this system. Eqn (5), (6), (7) and (8) show that e− contributes to generation ˙O2− and ˙OH. Based on the above findings, the ˙OH generated by e− and external H2O2 in eqn (8) are the most important active species in the pollutant degradation process. In the FST PEC/H2O2 synergistic degradation system, electrons are generated by FST photocatalysis, and bias voltage promotes separation of h+ and e−. Due to reactions involving photogenerated h+ and e−, ˙OH and ˙O2− are formed, while electrons promote the formation of ˙OH from a large amount of H2O2 in the solution, forming the photocatalytic-electro-H2O2 synergistic system to promote the rapid degradation of pollutants.
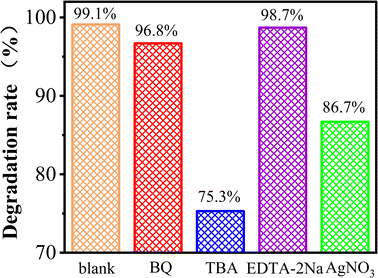 |
| Fig. 9 Degradation efficiency of MB using different radical scavengers. | |
4. Conclusions
In summary, visible-light driven PEC/H2O2 coupling system was developed for degradation of organic pollutants (MB, Rh B, MO, AMX) by magnetic FST-loaded photoelectrode. Through characterization by TEM, XRD, UV-vis DRS, BET, XPS and EIS, the chemical and physical properties of the FST catalyst were systematically analyzed. Taking advantage of the synergistic effect of photoelectrocatalysis and H2O2 photolysis, the system exhibited greatly enhanced performance. The FST PEC/H2O2 synergistic degradation system showed comparable degradation efficiencies for MB (98.8%), Rh B (96.7%), MO (97.7%), and AMX (83.9%), indicating the wide applicability for various organic pollutants wastewater treatment. In the PEC/H2O2 process, through applications of a bias voltage on the working electrode, photogenerated electrons were guided along the external electric field to the auxiliary electrode, thereby reducing h+–e− recombination, realizing the photo-electric coupling effect. At the same time, the photogenerated electrons were captured by a sufficient amount of H2O2 in the system to generate ˙OH, which increased the amounts of strong oxidizing substances and facilitated the separation of photogenerated h+ and e−. The results of quenching experiments also proved that ˙OH and ˙O2− were main reactive species in the photocatalytic-electro-H2O2 oxidation processes. This work brings new insights to efficiently purify organic pollutants by PEC coupled with peroxide under visible light illumination.
Author contributions
Bo Zhang: investigation, methodology, writing – original draft. Xuemei Li: conceptualization, validation, resources, writing – review & editing, supervision, project administration, funding acquisition. Yongshan Ma: software, visualization. Tianyi Jiang: data curation. Yanyan Zhu: validation. Huixue Ren: supervision, funding acquisition. All authors have approved the final version of the manuscript.
Conflicts of interest
There are no conflicts to declare.
Acknowledgements
This project was financially supported by Natural Science Foundation of Shandong Province (No. ZR2020MD115, ZR2020MB086, ZR202102280483).
References
- E. Bailón-García, A. Elmouwahidi, M. A. Álvarez, F. Carrasco-Marín, A. F. Pérez-Cadenas and F. J. Maldonado-Hódar, New carbon xerogel-TiO2 composites with high performance as visible-light photocatalysts for dye mineralization, Appl. Catal., B, 2017, 201, 29–40 CrossRef.
- P. An, Y. Fu, D. L. Wei, Y. L. Guo, W. C. Zhan and J. S. Zhang, Hollow Nitrogen-Rich Carbon Nanoworms with High Activity for Metal-Free Selective Aerobic Oxidation of Benzyl Alcohol, Acta Phys.-Chim. Sin., 2021, 37, 2001025 Search PubMed.
- S. Li, W. Xu, L. Meng, W. Tian and L. Li, Recent Progress on Semiconductor Heterojunction-Based Photoanodes for Photoelectrochemical Water Splitting, Small Sci., 2022, 2, 2100112 CrossRef CAS.
- Y. Zhang, S. M. Zhao, Q. W. Su and J. L. Xu, Visible light response ZnO–C3N4 thin film photocatalyst, Rare Met., 2021, 40, 96–104 CrossRef.
- M. Xiao, Y. Qi, Q. Feng, K. Li, K. Fan, T. Huang, P. Qu, H. Gai and H. Song, P-cresol degradation through Fe(III)-EDDS/H2O2 Fenton-like reaction enhanced by manganese ion: Effect of pH and reaction mechanism, Chemosphere, 2021, 269, 129436 CrossRef CAS PubMed.
- S. Qu, W. Wang, X. Pan and C. Li, Improving the Fenton catalytic performance of FeOCl using an electron mediator, J. Hazard. Mater., 2020, 384, 121494 CrossRef CAS PubMed.
- I. Sirés, C. Arias, P. L. Cabot, F. Centellas, J. A. Garrido, R. M. Rodríguez and E. Brillas, Degradation of clofibric acid in acidic aqueous medium by electro-Fenton and photoelectro-Fenton, Chemosphere, 2007, 66, 1660–1669 CrossRef PubMed.
- S. Periyasamy, X. Lin, S. O. Ganiyu, S. Kamaraj, A. Thiam and D. Liu, Insight into BDD electrochemical oxidation of florfenicol in water: kinetics, reaction mechanism, and toxicity, Chemosphere, 2022, 288, 132433 CrossRef CAS PubMed.
- R. Wang, J. Bai, Y. Li, Q. Zeng, J. Li and B. Zhou, BiVO4/TiO2(N2) Nanotubes Heterojunction Photoanode for Highly Efficient Photoelectrocatalytic Applications, Nano-Micro Lett., 2016, 9, 14 CrossRef PubMed.
- S. Wu and Y. H. Hu, A comprehensive review on catalysts for electrocatalytic and photoelectrocatalytic degradation of antibiotics, Chem. Eng. J., 2021, 409, 127739 CrossRef CAS.
- P. Alulema-Pullupaxi, P. J. Espinoza-Montero, C. Sigcha-Pallo, R. Vargas and J. L. Paz, Fundamentals and Applications of Photoelectrocatalysis as an Efficient Process to Remove Pollutants from Water: A Review, Chemosphere, 2021, 281, 130821 CrossRef CAS PubMed.
- J. Schneider, M. Matsuoka, M. Takeuchi, J. Zhang, Y. Horiuchi, M. Anpo and D. W. Bahnemann, Understanding TiO2 photocatalysis: mechanisms and materials, Chem. Rev., 2014, 114, 9919–9986 CrossRef CAS PubMed.
- Y. Z. Wang, M. Zu, X. S. Zhou, H. Lin, F. Peng and S. Q. Zhang, Designing efficient TiO2-based photoelectrocatalysis systems for chemical engineering and sensing, Chem. Eng. J., 2020, 381, 122605 CrossRef CAS.
- Y. Li, D. D. Cheng, Y. Luo and L. X. Yang, Coaxial Fe2O3/TiO2 nanotubes for enhanced photo-Fenton degradation of electron-deficient organic contaminant, Rare Met., 2021, 40, 3543–3553 CrossRef CAS.
- C. Zhai, M. Zhu, Y. Lu, F. Ren, C. Wang, Y. Du and P. Yang, Reduced graphene oxide modified highly ordered TiO2 nanotube arrays photoelectrode with enhanced photoelectrocatalytic performance under visible-light irradiation, Phys. Chem. Chem. Phys., 2014, 16, 14800–14807 RSC.
- Z. Q. Long, Q. G. Li, T. Wei, G. M. Zhang and Z. J. Ren, Historical development and prospects of photocatalysts for pollutant removal in water, J. Hazard. Mater., 2020, 395, 122599 CrossRef CAS PubMed.
- G. Mamba and A. Mishra, Advances in Magnetically Separable Photocatalysts: Smart, Recyclable Materials for Water Pollution Mitigation, Catalysts, 2016, 6, 79 CrossRef.
- W. J. Zhou, X. R. Xiao, Y. H. Liu and X. Zhang, Magnetic Se/Fe/PCN-Catalyzed Oxidative Cracking Alkenes in O2, Chin. J. Org. Chem., 2022, 42, 1849–1855 CrossRef.
- X. Y. Chen, J. F. Mao, C. Liu, C. Chen, H. E. Cao and L. Yu, An unexpected generation of magnetically separable Se/Fe3O4 for catalytic degradation of polyene contaminants with molecular oxygen, Chin. Chem. Lett., 2020, 31, 3205–3208 CrossRef CAS.
- D. Beydoun, R. Amal, G. Low and S. Mcevoy, Occurrence and prevention of photodissolution at the phase junction of magnetite and titanium dioxide, J. Mol. Catal. A: Chem., 2002, 180, 193–200 CrossRef CAS.
- S. Xu, W. Shangguan, J. Yuan, M. Chen and J. Shi, Preparations and photocatalytic properties of magnetically separable nitrogen-doped TiO2 supported on nickel ferrite, Appl. Catal., B, 2007, 71, 177–184 CrossRef CAS.
- P. M. Álvarez, J. Jaramillo, F. L. Ero and P. K. Plucinski, Preparation and characterization of magnetic TiO2 nanoparticles and their utilization for the degradation of emerging pollutants in water, Appl. Catal., B, 2010, 100, 338–345 CrossRef.
- Y. Chi, Q. Yuan, Y. Li, L. Zhao and W. Yan, Magnetically separable Fe3O4@SiO2@TiO2-Ag microspheres with well-designed nanostructure and enhanced photocatalytic activity, J. Hazard. Mater., 2013, 262, 404–411 CrossRef CAS PubMed.
- C. Feng, Y. Xie, J. Zhao and G. Lu, Photocatalytic degradation of dyes on a magnetically separated photocatalyst under visible and UV irradiation, Chemosphere, 2001, 44, 1159–1168 CrossRef.
- S. Kamaruddin and D. Stephan, The preparation of silica-titania core-shell particles and their impact as an alternative material to pure nano-titania photocatalysts, Catal. Today, 2011, 161, 53–58 CrossRef CAS.
- I. Kitsou, P. Panagopoulos, T. Maggos, M. Arkas and A. Tsetsekou, Development of SiO2@TiO2 core-shell nanospheres for catalytic applications, Appl. Surf. Sci., 2018, 441, 223–231 CrossRef CAS.
- M. Khan, C. S. L. Fung, A. Kumar, J. He and I. M. C. Lo, Unravelling mechanistic reasons for differences in performance of different Ti- and Bi-based magnetic photocatalysts in photocatalytic degradation of PPCPs, Sci. Total Environ., 2019, 686, 878–887 CrossRef CAS PubMed.
- A. Kumar, M. Khan, L. Fang and I. M. C. Lo, Visible-light-driven N-TiO2@SiO2@Fe3O4 magnetic nanophotocatalysts: synthesis, characterization, and photocatalytic degradation of PPCPs, J. Hazard. Mater., 2017, 370, 108–116 CrossRef PubMed.
- J. He, X. Zeng, S. Lan and I. M. C. Lo, Reusable magnetic Ag/Fe,N-TiO2@Fe3O4@SiO2 composite for simultaneous photocatalytic disinfection of E.coli and degradation of bisphenol A in sewage under visible light, Chemosphere, 2018, 217, 869–878 CrossRef PubMed.
- Q. Y. Li, K. R. Ma, Z. J. Ma, Q. Wei, J. G. Liu, S. P. Cui and Z. R. Nie, Preparation and enhanced photocatalytic performance of a novel photocatalyst: hollow network Fe3O4/mesoporous SiO2/TiO2 (FST) composite microspheres, Microporous Mesoporous Mater., 2018, 265, 18–25 CrossRef CAS.
- J. B. Cai, X. F. Lin and H. F. Zhou, Lysine surface modified Fe3O4@SiO2@TiO2 microspheres-based preconcentration and photocatalysis for in situ selective determination of nanomolar dissolved organic and inorganic phosphorus in seawater, Sens. Actuators, B, 2016, 224, 48–54 CrossRef.
- J. Rashid, M. A. Barakat, Y. Ruzmanova and A. Chianese, Fe3O4@SiO2@TiO2 nanoparticles for photocatalytic degradation of 2-chlorophenol in simulated wastewater, Environ. Sci. Pollut. Res., 2015, 22, 3149–3157 CrossRef CAS PubMed.
- J. Ma, S. Guo, X. Guo and H. G. Ge, Liquid-phase deposition of TiO2 nanoparticles on core-shell Fe3O4@SiO2 spheres: preparation, characterization, and photocatalytic activity, J. Nanopart. Res., 2015, 17, 1–11 CrossRef CAS.
- X. Hu, J. Yang and J. Zhang, Magnetic loading of TiO2/SiO2/Fe3O4 nanoparticles on electrode surface for photoelectrocatalytic degradation of diclofenac, J. Hazard. Mater., 2011, 196, 220–227 CrossRef CAS PubMed.
- J. Su, Y. Zhang, S. Xu, S. Wang, H. Ding and S. Pan, Highly efficient and recyclable triple-shelled Ag@Fe3O4@SiO2@TiO2 photocatalysts for degradation of organic pollutants and reduction of hexavalent chromium ions, Nanoscale, 2014, 6, 5181–5192 RSC.
- L. Zhang, W. Wang, S. Sun, Y. Sun, E. Gao and Z. Zhang, Elimination of BPA endocrine disruptor by magnetic BiOBr@SiO2@Fe3O4 photocatalyst, Appl. Catal., B, 2014, 148–149, 164–169 CrossRef CAS.
- K. Bezerra, T. R. Fiaschitello, G. Labuto, H. S. Freeman and S. Costa, Reuse of water from real reactive monochromic and trichromic wastewater for new cotton dyes after efficient treatment using H2O2 catalyzed by UV light, J. Environ. Chem. Eng., 2021, 9, 105731 CrossRef CAS.
- X. Li, S. Liu, D. Cao, R. Mao and X. Zhao, Synergetic activation of H2O2 by photo-generated electrons and cathodic Fenton reaction for enhanced self-driven photoelectrocatalytic degradation of organic pollutants, Appl. Catal., B, 2018, 235, 1–8 CrossRef CAS.
- P. D. R. A. Paula, R. P. Cavalcante, D. A. Da Silva, L. Silva, T. Silva, F. Gozzi, E. Mcglynn, A. Brady-Boyd, G. A. Casagrande and H. Wender, H2O2-assisted photoelectrocatalytic degradation of Mitoxantrone using CuO nanostructured films: Identification of by-products and toxicity, Sci. Total Environ., 2019, 651, 2845–2856 CrossRef PubMed.
- J. Sun, Y. Guo, Y. Wang and D. Cao, H2O2 assisted photoelectrocatalytic degradation of diclofenac sodium at g-C3N4/BiVO4 photoanode under visible light irradiation, Chem. Eng. J., 2018, 332, 312–320 CrossRef CAS.
- Z. Lei, X. Pang, N. Li, L. Lin and Y. Li, A novel two-step modifying process for preparation of chitosan-coated Fe3O4/SiO2 microspheres, J. Mater. Process. Technol., 2009, 209, 3218–3225 CrossRef CAS.
- Q. Zhang, J. B. Joo, Z. Lu and M. Dahl, Self-assembly and photocatalysis of mesoporous TiO2 nanocrystal clusters, Nano Res., 2011, 4, 103–114 CrossRef CAS.
- Y. Ma, J. Cui, M. Yin, X. Li and Y. Liu, Enhancement of visible light driven dye degradation and photocatalytic H2 evolution over MoS2 through combination with perylene diimide aggregates, New J. Chem., 2021, 45, 14432–14443 RSC.
- S. Komtchou, A. Dirany, P. Drogui, D. Robert and P. Lafrance, Removal of atrazine and its by-products from water using electrochemical advanced oxidation processes, Water Res., 2017, 125, 91–103 CrossRef CAS PubMed.
- P. Qiu, T. Zhao, X. Zhu, B. Thokchom, J. Yang, W. Jiang, L. Wang, Y. Fan, X. Li and W. Luo, A confined micro-reactor with a movable Fe3O4 core and a mesoporous TiO2 shell for a photocatalytic Fenton-like degradation of bisphenol A, Chin. Chem. Lett., 2021, 32, 1456–1461 CrossRef CAS.
- A. Li, G. Li, Y. Zheng, L. Feng and Y. Zheng, Photocatalytic Property and Reaction Mechanism of (Ni-Mo)/TiO2 Nano Thin Film Evaluated with Congo Red, Acta Phys.-Chim. Sin., 2012, 28, 457–464 CAS.
- M. R. Sohrabi and M. Ghavami, Comparison of direct yellow 12 dye degradation efficiency using UV/semiconductor and UV/H2O2/semiconductor systems, Desalination, 2010, 252, 157–162 CrossRef CAS.
- J. Ma, W. Song, C. Chen, W. Ma, J. Zhao and Y. Tang, Fenton Degradation of Organic Compounds Promoted by Dyes under Visible Irradiation, Environ. Sci. Technol., 2005, 39, 5810–5815 CrossRef CAS PubMed.
- R. Daghrir, P. Drogui and M. A. El Khakani, Photoelectrocatalytic oxidation of chlortetracycline using Ti/TiO2 photo-anode with simultaneous H2O2 production, Electrochim. Acta, 2013, 87, 18–31 CrossRef CAS.
- J. Xu, H. Olvera-Vargas, F. Y. H. Teo and O. Lefebvre, A comparison of visible-light photocatalysts for solar photoelectrocatalysis coupled to solar photoelectro-Fenton: application to the degradation of the pesticide simazine, Chemosphere, 2021, 276, 130138 CrossRef CAS PubMed.
- A. Sharma and B. Lee, Integrated ternary nanocomposite of TiO2/NiO/reduced graphene oxide as a visible light photocatalyst for efficient degradation of o-chlorophenol, J. Environ. Manage., 2016, 181, 563–573 CrossRef CAS PubMed.
- C. Qu and D. Liang, Novel electrochemical advanced oxidation processes with H2O2 generation cathode for water treatment: a review, J. Environ. Chem. Eng., 2022, 10, 107896 CrossRef CAS.
- P. J. Espinoza-Montero, P. Alulema-Pullupaxi and B. A. Frontana-Uribe, Electrochemical production of hydrogen peroxide on Boron-Doped diamond (BDD) electrode, Curr. Opin. Solid State Mater. Sci., 2022, 26, 100988 CrossRef CAS.
- D. Liu, R. Tian, J. Wang, E. Nie, X. Piao, X. Li and Z. Sun, Photoelectrocatalytic degradation of methylene blue using F doped TiO2 photoelectrode under visible light irradiation, Chemosphere, 2017, 185, 574–581 CrossRef CAS PubMed.
- F. Chen, Q. Yang, Y. Wang, J. Zhao, D. Wang, X. Li, Z. Guo, H. Wang, Y. Deng, C. Niu and G. Zeng, Novel ternary heterojunction photcocatalyst of Ag nanoparticles and g-C3N4 nanosheets co-modified BiVO4 for wider spectrum visible-light photocatalytic degradation of refractory pollutant, Appl. Catal., B, 2017, 205, 133–147 CrossRef CAS.
- C. Zhou, C. Lai, D. Huang, G. Zeng, C. Zhang, M. Cheng, L. Hu, J. Wan, W. Xiong, M. Wen, X. Wen and L. Qin, Highly porous carbon nitride by supramolecular preassembly of monomers for photocatalytic removal of sulfamethazine under visible light driven, Appl. Catal., B, 2018, 220, 202–210 CrossRef CAS.
|
This journal is © The Royal Society of Chemistry 2022 |
Click here to see how this site uses Cookies. View our privacy policy here.