DOI:
10.1039/D2RA05114A
(Paper)
RSC Adv., 2022,
12, 32027-32034
Theoretical study on the ferroelectric and light absorption properties of Li2SbBiO6 for harvesting visible light
Received
16th August 2022
, Accepted 24th October 2022
First published on 9th November 2022
Abstract
Ferroelectric oxides with large bandgaps have restricted applications in photovoltaic and photocatalytic fields. Based on recent experiments with the ferroelectric compound, LiSbO3, the stability and optoelectronic properties of a new ferroelectric compound, namely Li2SbBiO6, are investigated in this study. The calculated results demonstrate that Li2SbBiO6 satisfies the stability conditions of the elastic coefficients and phonon dynamics. Li2SbBiO6 maintains the ferroelectric polarization strength of LiSbO3 and significantly reduces the bandgap, and thus has been explored for applications in photovoltaic and photocatalytic fields. Li2SbBiO6 is a new potential ferroelectric oxide for harvesting visible light owing to its suitable bandgap and a large hole–electron effective mass ratio.
1. Introduction
Polar materials with non-centrosymmetric structures have pyroelectric, piezoelectric, ferroelectric, and nonlinear optical properties and have been found to exhibit unique applications in diverse fields.1–4 The conventional ferroelectric materials, LiNbO3 and LiTaO3, belong to the noncentrosymmetric R3c space group and show excellent ferroelectricity and unique features in the photovoltaic and photocatalytic decomposition of water.5–7 LiNbO3 and LiTaO3 are difficult to be promoted in photovoltaic and photocatalytic applications owing to their wide bandgaps. Some rare-earth ferroelectric oxides such as LuMnO3, YMnO3, and TbMnO3 do not have very strong ferroelectric polarization strengths as LiNbO3 and BiFeO3, but they exhibit excellent band gaps and high absorption coefficients, leading to significant interest in the ferroelectric photovoltaic field.8,9 The ferroelectric photovoltaic material, BiFeO3, can effectively maintain a high ferroelectric polarization strength and reduce the bandgap by doping to achieve high photovoltaic performance.10 The bandgap values of ferroelectric oxides with an LiNbO3 structure are usually larger than 3 eV and poorly absorb visible light.11–15 LiSbO3 with the LiNbO3 structure recently synthesized by Inaguma et al. showed potential ferroelectricity and a high ferroelectric Curie temperature.16 Rich structural phase transitions can appear in LiSbO3 at different pressures with large static dielectric tensor and insulating properties.17 LiSbO3 with a R3c structure has a large bandgap and mainly absorbs ultraviolet light according to our recent study.18 LiBiO3 with the Pccn space group can be prepared via the hydrothermal treatment method.19 Young et al. theoretically predicted that the energy of LiBiO3 with a R3c structure is relatively close to that of the Pccn space group and is a potentially stable ferroelectric photovoltaic material with a strong bulk photovoltaic effect.20 Bi-based materials have attracted attention for splitting water to produce hydrogen under visible light.21,22 In Bi-based compounds such as BiFeO3, BiVO4, Bi2O3, KBiO3, LiBiO3, and NaBiO3 excited by visible light, the bandgap can be reduced to below 3.0 eV due to hybridization between the O 2p and Bi 6s orbitals.23,24 The overlapping of O 2p and Bi 6s orbitals in Bi-based photocatalysts is beneficial for decreasing the bandgap to capture visible light.25 The introduction of Cr ions into BiFeO3 to form the Bi2FeCrO6 double perovskite with a R3 structure can also significantly reduce the bandgap and maintain a high ferroelectric polarization strength, effectively improving photovoltaic efficiency.26 Considering Sb and Bi elements in the VA group and the unique 6s orbital of the Bi element and double perovskite Bi2FeCrO6 with the R3 structure, the introduction of Bi elements to form Li2SbBiO6 in the ferroelectric material LiSbO3 can aid ferroelectric materials with the absorption of visible light. In this study, the stability and optoelectronic properties of Li2SbBiO6 are investigated using first-principles calculations. The calculation results show that Li2SbBiO6 satisfies stability conditions and maintains the ferroelectric strength of LiSbO3, while significantly reducing the bandgap to absorb visible light.
2. The calculation method and structure
First-principles calculations are based on the plane-wave basis set of the VASP software.27,28 The generalized gradient approximation and the plane pseudopotential wave method are used to deal with the interactions between electrons and ions.29 The Perdew–Burke–Ernzerhof (PBE) functional is used as the exchange–correlation function.30 The cutoff energy is chosen to be 520 eV, and the K-point sample including the Γ point is selected to be 6 × 6 × 6. The lattice parameters and atomic positions are completely relaxed in the calculations. The electron self-consistency accuracy is 10−6 eV, and the atomic force is less than 0.01 eV Å−1. The Heyd–Scuseria–Ernzerhof (HSE) hybrid functional is employed to calculate the bandgap and complex frequency-dependent dielectric matrix in random phase approximation (RPA) schemes with an 8 × 8 × 8 K-point sample.31 The elastic constants (Cij) are calculated using the strain–stress method in the VASP package with the 8 × 8 × 8 K-point sample.32 The standard berry phase method was applied to calculate ferroelectric polarization.33,34 A 2 × 2 × 2 supercell containing 80 atoms was used to calculate the phonon frequency for Li2SbBiO6, and a 4 × 4 × 4 K-point sample was used for this supercell. LiSbO3 exhibits the R3c symmetry based on experimental reports,16 while Li2SbBiO6 shows the R3 symmetry by Bi substitution for Sb similar to the Bi2FeCrO6 double perovskite, as shown in Fig. 1. The calculated lattice parameters are shown in Table 1, where the lattice parameters of R3c-LiSbO3 and R3c-LiBiO3 are in good agreement with the experimental16 and theoretical values,20 respectively.
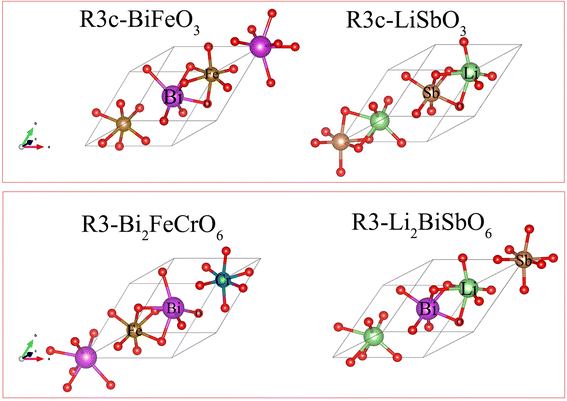 |
| Fig. 1 The structures of LiSbO3 and Li2SbBiO6. | |
Table 1 The calculated lattice parameters (lattice constant and angle α)
|
LiSbO3 |
LiSbO3 |
LiBiO3 |
LiBiO3 |
Li2SbBiO6 |
a (Å) |
5.39 (ref. 16) |
5.46 |
5.67 (ref. 20) |
5.71 |
5.60 |
α (°) |
56.4 (ref. 16) |
56.6 |
56 (ref. 20) |
56.1 |
56.3 |
3. The stability of Li2SbBiO6
R3c-LiSbO3 has six independent elasticity coefficients, while R3-Li2SbBiO6 has seven independent elasticity coefficients. The calculated elastic coefficients are shown in Table 2. The elastic coefficients of LiSbO3 satisfy the inequalities of the stability conditions of the R3c structure:35
C132 < 0.5 × C33 × (C11 + C12), |
C142 < 0.5 × C44 × (C11 − C12), |
Table 2 The calculated elastic coefficients (GPa)
|
C11 |
C12 |
C13 |
C14 |
C33 |
C44 |
C66 |
LiSbO3(present) |
245.3 |
52.0 |
86.4 |
−23.4 |
258.6 |
112.9 |
— |
LiSbO3 (ref. 18) |
283.6 |
56.2 |
104.0 |
−28.8 |
308.7 |
135.0 |
— |
LiSbO3(10 GPa) (ref. 18) |
322.3 |
77.8 |
130.3 |
−32.2 |
336.4 |
150.8 |
— |
LiSbO3(10 GPa) (ref. 17) |
289.6 |
75.0 |
119.8 |
−28.5 |
304.1 |
135.9 |
— |
LiSbO3(12 GPa) (ref. 17) |
295.8 |
79.3 |
124.6 |
−28.9 |
309.8 |
138.3 |
— |
Li2BiSbO6 |
196.7 |
50.2 |
68.7 |
−16.2 |
200.1 |
84.3 |
73.2 |
The seven independent elastic coefficients of Li2SbBiO6 also satisfy the stability conditions of the R3 space group:35
C132 < 0.5 × C33 × (C11 + C12), |
C142 + C152 < 0.5 × C44 × (C11 − C12), |
The elasticity coefficient of R3c-LiSbO3 calculated using the PBE function is smaller than that of the SCAN function (Table 2),18 and the elasticity coefficient becomes larger under high pressure conditions according to reports in the literature.17,18 All elasticity coefficients of R3c-LiSbO3 and R3-Li2SbBiO6 in Table 2 meet the stability conditions. The eigenvalue matrices of the elastic coefficients of LiSbO3 and Li2SbBiO6 in Table 3 are greater than 0 and satisfy the mechanical stability condition. The phonon frequencies and phonon density of states were calculated to verify the stability of LiSbO3 and Li2SbBiO6 (Fig. 2). The results show that they are potentially stable structures with no imaginary frequencies throughout the Brillouin zone. Li2SbBiO6 has a smaller elasticity coefficient than LiSbO3, which implies that mechanical properties, such as Young's modulus, bulk modulus, and hardness, may be modulated by the mutual doping of Sb and Bi. The calculated phonon density of state shows that the phonon frequency of Li2SbBiO6 shifts toward lower frequencies as compared to that of LiSbO3, which is in line with the trend of the reduced elasticity coefficient.
Table 3 The calculated eigenvalue matrices of elastic coefficients (GPa)
|
λ1 |
λ2 |
λ3 |
λ4 |
λ5 |
λ6 |
LiSbO3 |
79.7 |
100.7 |
131.1 |
156.2 |
207.6 |
396.7 |
Li2BiSbO6 |
61.6 |
78.3 |
101.0 |
124.2 |
159.1 |
318.7 |
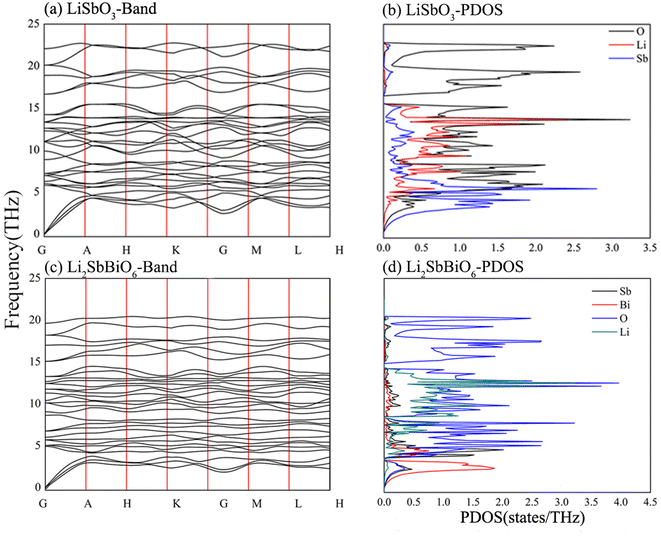 |
| Fig. 2 The calculated phonon frequency and phonon density of states. | |
4. Ferroelectricity analysis
The ferroelectric polarization strengths of LiSbO3 and Li2SbBiO6 are calculated using the berry phase method. As shown in Fig. 3, the energy curves and ferroelectric polarization strengths are calculated by the linear interpolation of the atomic positions between the centrosymmetric and polarized structures. The centrosymmetric and polarized structures for LiSbO3 are R
c and R3c, respectively, while R
and R3 structures are chosen for Li2SbBiO6. Displacement-type ferroelectric materials can form energy double potential well curves between the ferroelectric (polar) and paraelectric (centrosymmetric) states. The calculated energies of LiSbO3 and Li2SbBiO6 show significant ferroelectric double potential wells. Interestingly, the calculated ferroelectric polarization values of LiSbO3 and Li2SbBiO6 are 34 μc cm−2 and 39 μc cm−2, respectively. Thus, the ferroelectric polarization strength of Li2SbBiO6 is slightly greater than that of LiSbO3. According to our previous study, Li–O atomic interactions play a significant role in the ferroelectricity of LiSbO3.18 We further calculated the effect of the ionic movement on the ferroelectricity of Li2SbBiO6 (Fig. 4). The movement of Li–O atoms induces the double potential well shift and promotes the ferroelectric stability in Li2SbBiO6. This is consistent with the results of the phonon density of states calculations (Fig. 2), where evidently the Li–O phonon coupling effect is stronger in the high phonon frequency area.
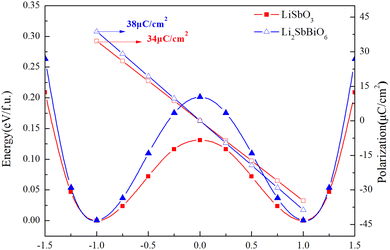 |
| Fig. 3 The calculated energy and polarization as the position of the atom changes. | |
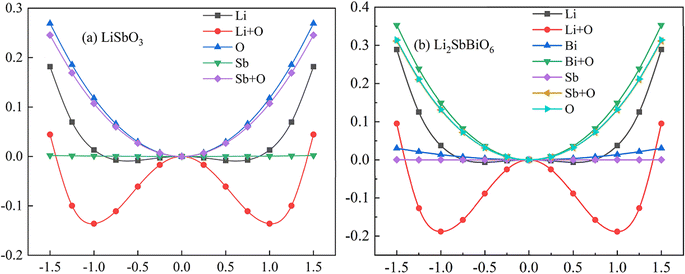 |
| Fig. 4 The calculated energy as the position changes with the movement of different atoms. | |
5. Discussion of photoelectric properties
As shown in Fig. 5, LiSbO3 and Li2SbBiO6 present the characteristics of an indirect bandgap material with 3.2 and 1.9 eV bandgap values, respectively. The calculated bandgap of LiSbO3 using the HSE06 functional is close to the value (3.4 eV) of the MBJ functional as-obtained in our earlier report.18 The energy band of Li2SbBiO6 has been calculated using the HSE06 + SOC (spin orbit coupling) approach due to the heavy Bi element. The calculation results from Fig. 6 show that the energy band structure of Li2SbBiO6 remains basically unchanged by the SOC effect, and the bandgap increases slightly to 2.1 eV. Considering the calculation of HSE06 + SOC is relatively expensive and HSE06 calculations can usually give accurate results in general, the HSE06 approach is mainly used to calculate the optical properties and electronic density of states of Li2SbBiO6. The bandgap of Li2SbBiO6 maintains the nature of the indirect bandgap of LiSbO3, which is unfavorable for photovoltaic performance, but the introduction of Bi ions can significantly reduce the bandgap to absorb visible light. From the density of states calculations (Fig. 5), the valence charges of both LiSbO3 and Li2SbBiO6 mainly comprise O-2p orbitals. It is also found that the antibonding states formed by O-2p and Bi-6s orbitals significantly reduce the bandgap of Li2SbBiO6. The absorption coefficients (α(ω)) of Li2SbBiO6 were acquired using the frequency-dependent dielectric matrix:
where ω is the incident light frequency, and c is the speed of light in a vacuum. The imaginary and real parts of the dielectric function are denoted by εi and εr, respectively. The calculated absorption coefficient of Li2SbBiO6 is shown in Fig. 7. LiSbO3 does not absorb visible light because of its large bandgap. Li2SbBiO6 can significantly absorb visible light and is a potential ferroelectric photovoltaic material. The bandgap of Li2SbBiO6 using the HSE06 calculation is 1.9 eV, which is close to that of the photocatalytic materials BiOI36 and LiBiO3 (ref. 37) with applications in photocatalytic water splitting. The bandgap value of Li2SbBiO6 is not as favorable as that of rare-earth ferroelectric oxides for absorbing visible light,8,9 but the ferroelectric polarization strength of Li2SbBiO6 is relatively large, which could be favorable for driving carrier separation by the depolarization field. A ferroelectric material with an internal electric field can reduce the probability of carrier recombination and improve the efficiency of photogenerated carriers. We calculated the effective mass to investigate the photoelectric properties. The expression for effective mass is m*(k) = ±ℏ2[∂2E(k)∕∂2k]−1. The calculation results of the effective mass and ferroelectric polarization strength are shown in Table 4. The calculated electron effective mass of LiSbO3 and Li2SbBiO6 is close to 0.5m0, and the electron carrier mobility could be larger due to the small effective mass. The ratio of mh/me was calculated to further explore the response to photoelectric performance. A lower recombination rate and higher transfer rate of photogenerated carriers could be induced for a larger ratio of mh/me. The mh/me ratios of Li2SbBiO6 are 7.414 and 5.934 without and with the SOC effect, respectively, which are greater than those of LiSbO3 polarized structures. The mh/me ratio of the ferroelectric material BiFeO3 is 4.589,38 and the ferroelectric polarization strength is higher than that of Li2SbBiO6, but the bandgap of BiFeO3 is relatively large and absorbs only a small amount of visible light for photocatalytic properties.39,40 The high degradation efficiency could arise from the strong visible light absorption, depolarization field of ferroelectrics, intrinsic small carrier effective mass, and large mh/me ratio in Li2SbBiO6, which contribute to the photoinduced carrier separation in the photocatalytic process.
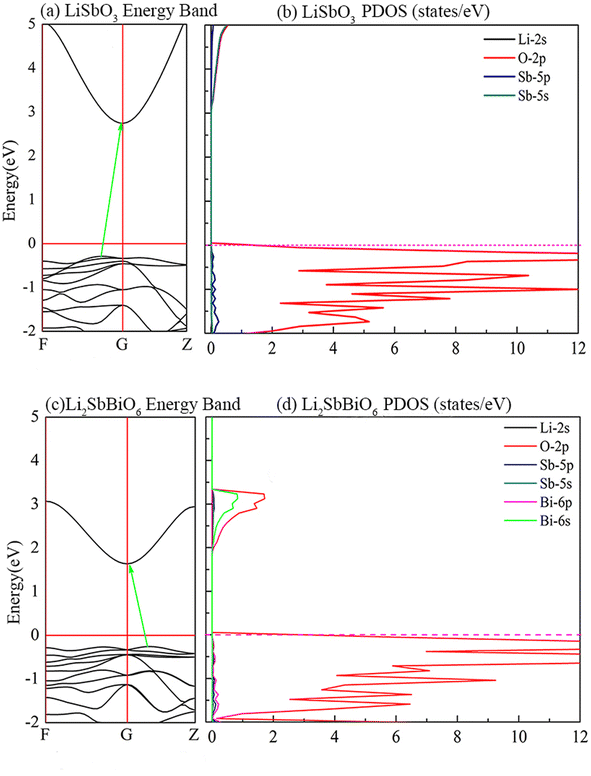 |
| Fig. 5 The calculated energy band structure and density of states. | |
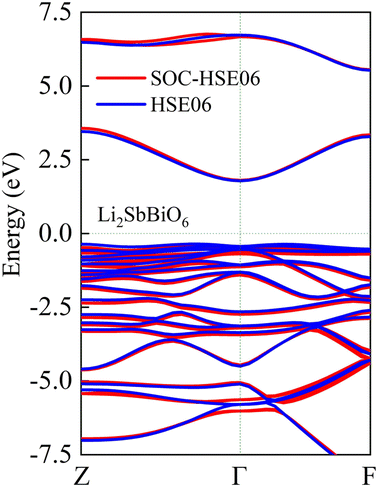 |
| Fig. 6 The calculated energy band of Li2SbBiO6 with and without the SOC effect. | |
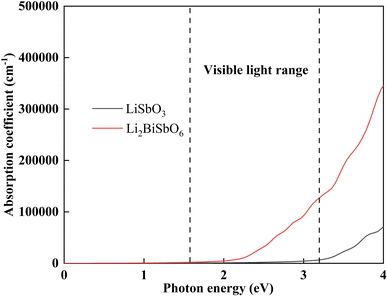 |
| Fig. 7 The calculated light absorption coefficient. | |
Table 4 The calculated effective mass of holes (mh) and electrons (me), the ratio of mh/me, and ferroelectric polarization strength (Ps)
Compounds |
mh (m0) |
me (m0) |
mh/me |
Ps (μc cm−2) |
LiSbO3 |
1.484 |
0.433 |
3.424 |
34 |
LiBiO3 |
1.651 |
0.504 |
3.272 |
47 (50 (ref. 20)) |
Li2SbBiO6 |
3.618(3.258-soc) |
0.488(0.549-soc) |
7.414(5.934-soc) |
39 |
BiFeO3 |
3.171 (ref. 38) |
0.691 (ref. 38) |
4.589 |
90 |
6. Conclusions
The stability and photoelectric properties of the Li2SbBiO6 ferroelectric compound have been investigated using density functional theory. The calculation results as well as the elastic coefficient and phonon dynamics analyses show that Li2SbBiO6 is a potentially stable ferroelectric material. The ferroelectric stability of Li2SbBiO6 and LiSbO3 is related to the strong effect of the Li–O bond. Li2SbBiO6 slightly improves the ferroelectric polarization of LiSbO3 and significantly reduces the bandgap to expand its applications in photovoltaic and photocatalysis with visible light. The larger mh/me ratio of Li2SbBiO6 accompanied by a suitable bandgap and ferroelectric properties is more favorable for the separation of carriers and absorption of visible light.
Conflicts of interest
The authors declared that they have no conflicts of interest to this work. We declare that we do not have any commercial or associative interest that represents a conflict of interest in connection with the work submitted.
Acknowledgements
This work was supported by the Natural Science Foundation of Guangdong Province, China (Grant No. 2019A1515011914, 2018A030307028, and 2019A1515010916), Maoming Natural Science Foundation of Guangdong, China (Grant No. 2019018001), Guangdong University Student Climbing Project, China (Grant No. pdjh2021a0331), and Natural Science Foundation of Guangdong University of Petrochemical Technology (no. 2017rc19).
References
- P. S. Halasyamani and K. R. Poeppelmeier, Noncentrosymmetric oxides, Chem. Mater., 1998, 10(10), 2753–2769 CrossRef CAS.
- Y. Zhang, P. T. T. Phuong and E. Roake, et al., Thermal energy harvesting using pyroelectric-electrochemical coupling in ferroelectric materials, Joule, 2020, 4(2), 301–309 CrossRef CAS.
- C. Wu, G. Yang and M. G. Humphrey, et al., Recent advances in ultraviolet and deep-ultraviolet second-order nonlinear optical crystals, J. Coord. Chem., 2018, 375, 459–488 CrossRef CAS.
- Y. Zhang, W. Jie and P. Chen, et al., Ferroelectric and piezoelectric effects on the optical process in advanced materials and devices, Adv. Mater., 2018, 30(34), 1707007 CrossRef PubMed.
- I. Inbar and R. E. Cohen, Origin of ferroelectricity in LiNbO3 and LiTaO3, Ferroelectrics, 1997, 194(1), 83–95 CrossRef CAS.
- A. M. Glass, D. Linde and T. J. Negran, High-voltage bulk photovoltaic effect and the photorefractive process in LiNbO3[M], Landmark Papers On Photorefractive Nonlinear Optics, 1995, pp. 371–373 Search PubMed.
- S. Takasugi, K. Tomita and M. Iwaoka, et al., The hydrothermal and solvothermal synthesis of LiTaO3 photocatalyst: Suppressing the deterioration of the water splitting activity without using a cocatalyst, Int. J. Hydrogen Energy, 2015, 40(16), 5638–5643 CrossRef CAS.
- H. Han, S. Song and J. H. Lee, et al., Switchable photovoltaic effects in hexagonal manganite thin films having narrow band gaps, Chem. Mater., 2015, 27(21), 7425–7432 CrossRef CAS.
- X. Huang, T. R. Paudel and S. Dong, et al., Hexagonal rare-earth manganites as promising photovoltaics and light polarizers, Phys. Rev. B: Condens. Matter Mater. Phys., 2015, 92(12), 125201 CrossRef.
- L. You, F. Zheng and L. Fang, et al., Enhancing ferroelectric photovoltaic effect by polar order engineering, Sci. Adv., 2018, 4(7), 3438 CrossRef PubMed.
- J. He, C. Franchini and J. M. Rondinelli, Lithium niobate-type oxides as visible light photovoltaic materials, Chem. Mater, 2016, 28(1), 25–29 CrossRef CAS.
- K. P. Ong, X. Fan and A. Subedi, et al., Transparent conducting properties of SrSnO3 and ZnSnO3, APL Mater., 2015, 3(6), 062505 CrossRef.
- J. Zhang, B. Xu and Y. S. Wang, et al., First-principles investigation of the ferroelectric, piezoelectric and nonlinear optical properties of LiNbO3-type ZnTiO3, Sci. Rep., 2019, 9(1), 1–14 CrossRef PubMed.
- X. F. Xu, X. L. Cai and K. R. Su, et al., Tuning mechanical properties, ferroelectric properties and electronic structure in R3c-MgSnO3 by compressive strain: A first-principle study, Phys. B, 2021, 413143 CrossRef CAS.
- K. Fujiwara, H. Minato and J. Shiogai, et al., Thin-film stabilization of LiNbO3-type ZnSnO3 and MgSnO3 by molecular-beam epitaxy, APL Mater., 2019, 7(2), 022505 CrossRef.
- Y. Inaguma, A. Aimi and D. Mori, et al., High-pressure synthesis, crystal structure, chemical bonding, and ferroelectricity of LiNbO3-type LiSbO3, Inorg. Chem., 2018, 57(24), 15462–15473 CrossRef CAS PubMed.
- S. M. Yang, A. J. Mao and H. Cheng, et al., Pressure-Induced High-κ Dielectric Properties and Multiple Phase Transitions between Novel Nonperovskite and Perovskite Phases in LiSbO3: A First-Principles Study, J. Phys. Chem. C, 2020, 125(1), 878–885 CrossRef.
- K. R. Su, X. F. Xu and G. X. Lai, et al., First-principles investigation of the elastic, photocatalytic and ferroelectric properties of LiNbO3-type LiSbO3 under high pressure, Mater. Today Commun., 2021, 27, 102406 CrossRef CAS.
- T. Takei, R. Haramoto and Q. Dong, et al., Photocatalytic activities of various pentavalent bismuthates under visible light irradiation, J. Solid State Chem., 2011, 184(8), 2017–2022 CrossRef CAS.
- S. M. Young, F. Zheng and A. M. Rappe, First-Principles Materials Design of High-Performing Bulk Photovoltaics with the LiNbO3 Structure, Phys. Rev. Appl., 2015, 4(5), 054004 CrossRef.
- H. Cheng, B. Huang and Y. Dai, Engineering BiOX (X= Cl, Br, I) nanostructures for highly efficient photocatalytic applications, Nanoscale, 2014, 6(4), 2009–2026 RSC.
- S. Y. Yu, L. Zhang and L. B. Zhu, et al., Bismuth-containing semiconductors for photoelectrochemical sensing and biosensing, Coordin. Chem. Rev, 2019, 393, 9–20 CrossRef CAS.
- S. Cao, P. Zhou and J. Yu, Recent advances in visible light Bi-based photocatalysts, Chin. J. Catal., 2014, 35(7), 989–1007 CrossRef.
- R. He, D. Xu and B. Cheng, et al., Review on nanoscale Bi-based photocatalysts, Nanoscale Horiz., 2018, 3(5), 464–504 RSC.
- M. Xu, J. Yang and C. Sun, et al., Performance enhancement strategies of Bi-based photocatalysts: a review on recent progress, Chem. Eng. J., 2020, 389, 124402 CrossRef CAS.
- S. Li, B. AlOtaibi and W. Huang, et al., Epitaxial Bi2FeCrO6 multiferroic thin film as a new visible light absorbing photocathode material, Small, 2015, 11(32), 4018–4026 CrossRef CAS PubMed.
- G. Kresse and J. Hafner, Ab initio molecular dynamics for liquid metals, Phys. Rev. B: Condens. Matter Mater. Phys., 1993, 47(1), 558 CrossRef CAS PubMed.
- G. Kresse and J. Furthmüller, Efficient iterative schemes for ab initio total-energy calculations using a plane-wave basis set, Phys. Rev. B: Condens. Matter Mater. Phys., 1996, 54(16), 11169 CrossRef CAS PubMed.
- G. Kresse and D. Joubert, From ultrasoft pseudopotentials to the projector augmented-wave method, Phys. Rev. B: Condens. Matter Mater. Phys., 1999, 59(3), 1758 CrossRef CAS.
- J. P. Perdew, K. Burke and M. Ernzerhof, Generalized gradient approximation made simple, Phys. Rev. Lett., 1996, 77(18), 3865 CrossRef CAS PubMed.
- J. Heyd, G. E. Scuseria and M. Ernzerhof, Hybrid functionals based on a screened Coulomb potential, J. Chem. Phys., 2003, 118(18), 8207–8215 CrossRef CAS.
- Y. Le Page and P. Saxe, Symmetry-general least-squares extraction of elastic data for strained materials from ab initio calculations of stress, Phys. Rev. B: Condens. Matter Mater. Phys., 2002, 65(10), 104104 CrossRef.
- R. D. King-Smith and D. Vanderbilt, Theory of polarization of crystalline solids, Phys. Rev. B: Condens. Matter Mater. Phys., 1993, 47(3), 1651 CrossRef CAS PubMed.
- N. A. Benedek, A. T. Mulder and C. J. Fennie, Polar octahedral rotations: a path to new multifunctional materials, J. Solid State Chem., 2012, 195, 11–20 CrossRef CAS.
- F. Mouhat and F. X. Coudert, Necessary and sufficient elastic stability conditions in various crystal systems, Phys. Rev. B: Condens. Matter Mater. Phys., 2014, 90(22), 224104 CrossRef.
- X. Zhang, Z. Ai and F. Jia, et al., Generalized one-pot synthesis, characterization, and photocatalytic activity of hierarchical BiOX (X = Cl, Br, I) nanoplate microspheres, J. Phys. Chem. C, 2008, 112(3), 747–753 CrossRef CAS.
- R. Ramachandran, M. Sathiya and K. Ramesha, et al., Photocatalytic properties of KBiO3 and LiBiO3 with tunnel structures, J. Chem. Sci, 2011, 123(4), 517–524 CrossRef CAS.
- J. He, C. Franchini and J. M. Rondinelli, Ferroelectric oxides with strong visible-light absorption from charge ordering, Chem. Mater., 2017, 29(6), 2445–2451 CrossRef CAS.
- F. Gao, X. Y. Chen and K. B. Yin, et al., Visible-light photocatalytic properties of weak magnetic BiFeO3 nanoparticles, Adv. Mater., 2007, 19(19), 2889–2892 CrossRef CAS.
- S. M. Lam, J. C. Sin and A. R. Mohamed, A newly emerging visible light-responsive BiFeO3 perovskite for photocatalytic applications: a mini review, Mater. Res. Bull., 2017, 90, 15–30 CrossRef CAS.
|
This journal is © The Royal Society of Chemistry 2022 |
Click here to see how this site uses Cookies. View our privacy policy here.