DOI:
10.1039/D2RA04158H
(Paper)
RSC Adv., 2022,
12, 21160-21171
Phase behavior of binary and ternary fluoropolymer (PVDF-HFP) solutions for single-ion conductors
Received
5th July 2022
, Accepted 19th July 2022
First published on 2nd August 2022
Abstract
A fluoropolymer poly(vinylidene fluoride-co-hexafluoropropylene) (PVDF-HFP) has a dielectric constant of ∼11, providing charge screening effects. Hence, this highly polar PVDF-HFP material has been employed as a matrix for solid polymer electrolytes (SPEs). In this study, the phase behavior of binary PVDF-HFP solutions was analyzed using the Flory–Huggins theory, in which ethylene carbonate, propylene carbonate, dimethyl carbonate, γ-butyrolactone, and acetone were employed as model solvents. In particular, for the binary PVDF-HFP/acetone system, the solid–liquid and liquid–liquid phase transitions were qualitatively described. Then, the phase diagram for ternary acetone/PVDF-HFP/polyphenolate systems was constructed, in which the binodal, spinodal, tie-line, and critical point were included. Finally, when a polyelectrolyte lithium polyphenolate was mixed with the PVDF-HFP matrix, it formed a single-ion conductor with a Li+ transference number of 0.8 at 23 °C. In the case of ionic conductivity, it was ∼10−5 S cm−1 in solid state and ∼10−4 S cm−1 in gel state, respectively.
Introduction
Poly(vinylidene fluoride-co-hexafluoropropylene) (PVDF-HFP) has been a benchmark matrix for solid-state polymer electrolytes due to its superior dielectric constant (εr ≈ 11), low glass transition temperature, high mechanical strength, and electrochemical stability.1–6 Specifically, the high εr affords a small binding energy with salt ions leading to an effective dissociation of charged particles, whereas the low Tg allows the polymer segmental motion (to be active) in its amorphous regions. These characteristics provide a pathway for an enhanced ionic conductivity depending on both charge concentration and mobility. Basically, all the properties of the fluoropolymer PVDF-HFP are largely governed by its chemical structure in the copolymer backbone. If the HFP content in the VDF/HFP units is less than 15–19 mol%, the copolymer is semicrystalline with thermoplastic properties, desirable for solid-state polymer electrolyte applications.4–7 The polymer electrolytes are composed of a polymer matrix and salt (or ionic liquid or polyelectrolyte), in which both plasticizer and active/passive filler could be added for improving ionic conductivity and mechanical properties, respectively.8–11 Furthermore, instead of a single polymer matrix, two different polymers can be mixed together to form a blend. For example, PVDF-HFP has been blended with other molecules such as poly(ethylene oxide) (PEO), poly(methyl methacrylate) (PMMA), poly(vinyl acetate) (PVAc), poly(vinyl chloride) (PVC), thermoplastic polyurethane (TPU), poly(methyl methacrylate-co-acrylonitrile-co-lithium methacrylate) (PMAML), poly(ionic liquid), polysiloxane, and carboxymethyl cellulose (CMC).8,12–26 Here, it is notable that these polymer electrolytes have been developed for solid-state batteries (SSBs), which are one of the post-lithium-ion batteries (PLIBs) including sodium-ion batteries (SIBs), lithium–sulfur batteries (LSBs), and lithium–air batteries (LABs).27–32 Specifically, the perfluoropolyether-based block copolymer electrolyte was designed for ultra-stable SIBs.33 In addition, versatile new concepts such as flexible cross-linked network electrolytes, metal–organic framework (MOF)-based electrolytes, porous organic cage ionic conductors, an aligned liquid crystalline polymer combined with ionic liquids and salt, polymer-in-salt electrolytes, and dual-/single-ion conductors have been introduced for the next-generation PLIB applications.34–38
In this study, I investigated the single-ion conductor based on the polymer blend composed of PVDF-HFP and lithium polyphenolate (LPF), in which LPF is a polyelectrolyte providing Li+ ions for the PVDF-HFP matrix. Through this work, I tried to improve the existing PEO/LPF-based single conductor39 by replacing PEO with the high-performance PVDF-HFP matrix. This is because PEO has a low dielectric constant (εr ≈ 5) and too high crystallinity (∼70–80%), leading to a relatively low ionic conductivity.40–42 Hence, this work was focused on the analysis of PVDF-HFP solutions and then, the application of PVDF-HFP/LPF blends to the solid polymer electrolytes (SPEs). Firstly, the phase behavior of binary and ternary PVDF-HFP solutions was investigated, for which the Flory–Huggins lattice theory was employed.43–48 To date, most studies on the phase behavior of PVDF or PVDF-HFP solutions have been carried out through experiments.49–54 An exception for this state is that Chen and his coworkers simply calculated the phase diagram of PVDF/dimethylacetamide (DMAc)/H2O and described the PVDF-membrane formation via nonsolvent induced phase inversion.50 In contrast, Wang et al. compared in their experiments the phase behavior of two different fluoropolymer-based ternary systems, i.e., PVDF/DMAc/H2O and poly(vinylidenedifluoride-co-chlorotrifluoroethylene) (PVDF-CTFE)/DMAc/H2O.52 Here, the former was more easily phase-separable than the latter. Then, Shi et al. studied the effect of additive (LiCl and glycerol) on the phase behavior of PVDF-HFP/n-methyl-2-pyrrolidone (NMP)/H2O and found that the additive may alter the morphology and structure of the resulting membrane through a facilitated phase separation.53 Recently, Wei and his coworkers studied the effect of LiCl on phase behavior of the PVDF-CTFE/DMAc/H2O system and found that LiCl addition promoted both solid–liquid and liquid–liquid phase transitions, which is in line with Shi et al.'s results.54 Therefore, considering the deficiency of theoretical calculation for the PVDF-HFP solution thermodynamics, this work seems to bridge the gap in this field by providing theoretical predictions about the phase behavior of PVDF-HFP solutions in terms of binodal, spinodal, tie line, and critical point.43–48 For this purpose, the Flory–Huggins interaction parameter (χ) was essential, which was calculated based on solubility parameter (δ).55,56 However, if δ is unknown, it could be estimated through a group contribution method.57 Finally, with the understanding of phase behavior of PVDF-HFP solutions, PVDF-HFP was blended with a polyelectrolyte lithium polyphenolate (LPF) in acetone for the SPE applications. The resulting SPE showed a Li+-transference number of ∼0.8 as a single-ion conductor minimizing a concentration gradient and cell polarization in rechargeable lithium batteries.10,58,59
Materials and methods
Materials
PVDF-HFP (Mn ≈ 120.0 kg mol−1, Mw ≈ 400.0 kg mol−1, and polydispersity index (PDI) = 3.3) was provided from Elf Atochem, which is composed of VDF
:
HFP with 88
:
12 by mole ratio (Kynar-FLEX® 2801). P-Toluenesulfonyl chloride, HEPES buffer solution, horseradish peroxidase II (HRP II), NaOH, MgSO4, SiO2, hydroquinone, and other solvents were purchased from Sigma-Aldrich and used as received.
Methods
1H nuclear magnetic resonance (NMR) spectra were obtained using an NMR spectrometer (Bruker). Infrared (IR) absorption data were obtained from IR spectrometer (Bomem, MB 100-C15) at 4000–400 cm−1. Here, the KBr disc method was used for sample preparation. Thermal analysis was carried out by differential scanning calorimetery (DSC) (DuPont model 910 thermal analyzer) at a scanning rate of 10 °C min−1 under N2 with a flow rate of 50 mL min−1. Note that, in this study, the DSC data were reported based on the first heating curve. Thermogravimetric analysis (TGA) was performed using a TA instruments over a temperature range of 25–700 °C at a scanning rate of 10 °C min−1 under N2 with a flow rate of 50 mL min−1. Impedance data were obtained for estimating both the ionic conductivity (σ) and the transference number of Li+ ions (tLi+) by using a frequency response analyzer (FRA, Solartron SI 1260), for which a stainless steel (SUS) or Li symmetrical cell was used, respectively. Note that the performance of polymer electrolyte (e.g., the reproducibility of ionic conductivity data) was evaluated through the 1st order linear fitting as a function of composition, indicating that both y-intercept and slope have the standard errors of ∼10−6 S cm−1. Through this regression analysis, the data distribution could be estimated although there should be experimental uncertainties.
SPEs were prepared by dissolving LPF
:
PVDF-HFP (=1
:
1 to 1
:
5 by wt ratio) in acetone and cast it in a Teflon plate (see Scheme 1 for chemical structure of LPF). Then the cell with stainless steel (SUS)/SPE/SUS or Li/SPE/Li configuration was assembled and vacuum-sealed using a blue bag from Shield Pack, Inc. in a glove box under argon environment. Here, SUS/SPE/SUS is for estimating the bulk resistance and ionic conductivity of SPE, whereas Li/SPE/Li is for measuring Li+ ion's transference number (tLi+).
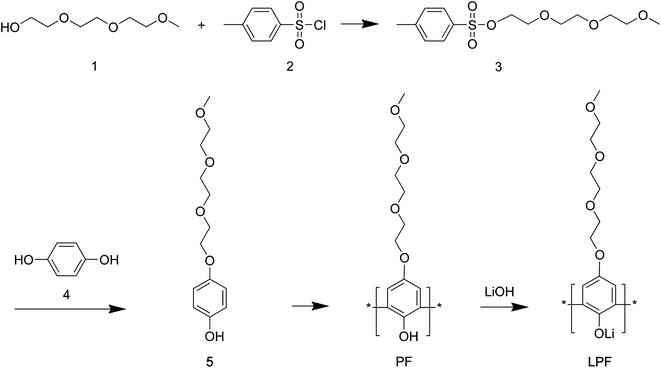 |
| Scheme 1 Synthesis of polyphenols: polyphenolate (PF) and lithium polyphenolate (LPF). | |
Synthesis
Synthesis of (3). NaOH (11.4 g, 0.285 mol) was dissolved in 60 mL H2O to make 5 M NaOH. Tri(ethylene glycol) monomethyl ether (1) (32.84 g, 0.2 mol) was dissolved in 50 mL THF (Scheme 1). Subsequently, two solutions were mixed together in 1000 mL flask under less than 5 °C. Then, p-toluenesulfonyl chloride (2) (36.2 g, 0.18 mol) in 50 mL of THF was dropwise added into the above solution and mixed. After about 2 hours, the solution's color was change into milky white, indicating the successful reaction. The solution was poured into 400 mL cold water. Using diethyl ether 50 mL, the synthesized chemical was extracted (×three times). It was washed several times and finally dried. The product was a transparent yellowish liquid.
Synthesis of (5). Hydroquinone (4) (30.8 g, 279.71 mmol) and (3) (89 g, 279.75 mmol), KOH (39 g, 837 mmol) were mixed in 200 mL DMSO and then reacted for 12 hours at 23 °C. Then using dimethyl ether, HCl and chloroform, the product was extracted. It was washed using water, dried using MgSO4, and finally dried under vacuum at 23 °C.
Synthesis of PF. HRP II (24 mg) was dissolved in 55 mL HEPES buffer solution in 250 mL three-neck flask. Then (5) (8.83 g, 34.46 mmol) was dissolved in 25 mL 1,4-dioxane, and then this solution (5 in 1,4-dioxane) was added slowly into the above HRP-II/HEPES buffer solution. Then 30% H2O2 solution (1 mL) was added into this buffer solution, which was repeated for 5 times per 10 min under below 5 °C. Then solution's color was gradually changed to yellow. Then the reaction was allowed for additional ∼10–15 min under 23 °C and stirred overnight, resulting in dark brown color.
Synthesis of LPF. PF (4 g, 15.7 mmol) was dissolved in 20 mL 1,4-dioxane. LiOH (38 mg, 15.7 mmol) was dissolved in 10 mL water. Then two solutions were mixed together, resulting in a black colored solution. Then 1,4-dioxane and water was removed from the product using a rotary evaporator. Then the product was stored in vacuum overnight.
Results and discussion
Dielectric property of PVDF-HFP
The electric potential energy (UE) between two charges (q1 and q2) in a dielectric is a function of the permittivity (ε = ε0εr) of a material as well as charge-separation distance (r12). |
 | (1) |
where ε0 and εr are the vacuum permittivity and a relative permittivity (or dielectric constant), respectively. Fig. 1a shows the coulombic potential well describing the binding energy between cation and anion when the dielectric constant of polymer is εr ≈ 11 for PVDF-HFP (its chemical structure in Fig. 1b) and εr ≈ 5 for PEO, respectively.6,39,60 According to eqn (1), when is high, the binding energy between cation and anion should be small because of the screening effect of a polarized medium on charges. Thus, PVDF-HFP displays a narrow coulombic potential well, whereas PEO exhibits a wide one. For example, when ion separation distance is 10 Å, the binding energy is 0.29 eV in PEO, whereas 0.13 eV in PVDF-HFP. This characteristic implies that the charge concentration for ionic conductivity will be higher in PVDF-HFP than in PEO. Thus, for developing SPEs, it is reasonable to replace PEO with PVDF-HFP or other high dielectric fluoropolymers, e.g., PVDF-CTFE (εr ≈ 13), and poly(vinylidenefluoride-co-trifluoroethylene) (PVDF-TrFE) (εr ≈ 18).3–6
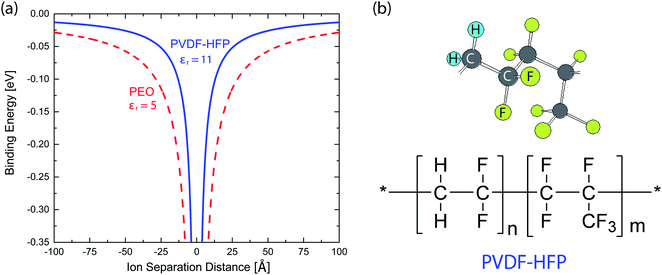 |
| Fig. 1 (a) Binding energy as a function of ion separation distance. Here, a monovalent cation is assumed to be at the origin, whereas a monovalent anion at the indicated distance. Potential wells were calculated for PVDF-HFP with εr ≈ 11 and PEO with εr ≈ 5. (b) Chemical structure of PVDF-HFP copolymer. | |
Phase behavior of binary PVDF-HFP solutions
The Flory–Huggins theory can describe the phase behavior of polymer solutions, for which the two parameters such as interaction parameter (χ) and the relative molar volume of a polymer (r2) should be provided. In the case of solvent, r1 = 1. The molar Gibbs energy of mixing (ΔGmix) for a binary polymer solution is expressed as follows,45,61 |
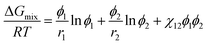 | (2) |
where R, T, and ϕi are the gas constant, temperature (K), and the volume fraction of component i (=1 for solvent and 2 for polymer). Here, χij could be estimated from the solubility parameter information as follows, |
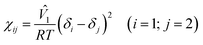 | (3) |
where
1 and δi are a molar volume of solvent and a solubility parameter of component i. Here, the χ parameter is inversely proportional to temperature. Then, the equilibrium condition of chemical potentials, Δμi (= ∂ΔGmix/∂ni), is expressed as follows, |
Δμαi = Δμβi (i = 1, 2)
| (4) |
where α and β indicate two different phases at equilibrium. Using eqn (4), the upper critical solution temperature (UCST) phase behavior could be predicted. Furthermore, the melting point of a binary polymer solution could be described as follows, |
 | (5) |
where Tm and T0m are the temperatures of a binary solution and a pure polymer, respectively. ΔHu is the enthalpy of polymer's structural unit (when crystallinity is 100%), and Vu is the volume of polymer's structural unit. In general, the chain length of polymer is much larger than that of solvent (i.e., r2 ≫ r1 = 1). Thus, the eqn (5) could be reduced as follows, |
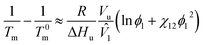 | (6) |
which is called Nish–Wang equation.62
Specifically, propylene carbonate (PC), ethylene carbonate (EC), dimethyl carbonate (DMC), and γ-butyrolactone (GBL) have been used as solvent (or plasticizer) for polymer electrolytes.62–64 Thus, using these solvents, the UCST phase behavior of PVDF-HFP solutions was calculated. As a first step, the model polymer (PVDF-HFP) was assumed to have a moderate molecular weight Mn = 40.0 kg mol−1 with δ2 = 11.3 (cal cm−3)1/2.63 Then, the properties of solvents were summarized in Table 1.64 According to the Flory–Huggins model, the PVDF-HFP solutions exhibited a better miscibility with the sequence of GBL > DMC > EC > PC, which was largely governed by χ12 parameters (Table 1). Note that smaller χ12, more miscible each other. In Fig. 2, the critical point (ϕc2,Tc) is (0.06, 302.96) for PVDF-HFP/PC, (0.05, 249.56) for PVDF-HFP/EC, (0.06, 147.56) for PVDF-HFP/DMC, and (0.06, 115.91) for PVDF-HFP/GBL, respectively. Here, it is notable that the Flory–Huggins model can capture ‘qualitatively’ the trend of UCST phase behaviors of binary polymer solutions.
Table 1 Solubility parameter (δ1), molecular weight (MW), density (d), molar volume (
1), relative molar volume (r2), and interaction parameter (χ12) for PVDF-HFP/solvent systems. Here, PVDF-HFP as a model system has δ2 = 11.3 (cal cm−3)1/2, d = 1.77 g cm−3, Mn = 40.0 kg mol−1, and molar volume = 22
600 cm3 mol−1
Solvent |
δ1 (MPa1/2) |
δ1 (cal cm−3)1/2 |
MW (g mol−1) |
d (g cm−3) |
1 (cm3 mol−1) |
r2 (−) |
χ12 (−) |
EC |
30.1 |
14.7 |
88.06 |
1.3214 |
66.64 |
339 |
138.70 K/T |
PC |
27.2 |
13.3 |
102.09 |
1.2047 |
84.74 |
267 |
170.59 K/T |
GBL |
25.8 |
12.6 |
86.06 |
1.1284 |
76.29 |
296 |
64.89 K/T |
DMC |
20.3 |
9.9 |
90.08 |
1.0697 |
84.21 |
268 |
83.07 K/T |
Ace |
20.3 |
9.9 |
58.08 |
0.7845 |
74.03 |
305 |
73.02 K/T |
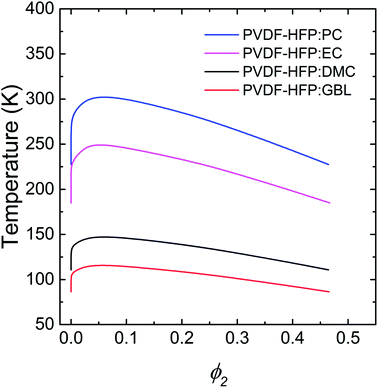 |
| Fig. 2 The UCST phase behavior of binary PVDF-HFP solutions, which was calculated based on the Flory–Huggins model. Here, PC, EC, DMC, and GBL were used as a model solvent or plasticizer. PVDF-HFP has Mn = 40.0 kg mol−1 and δ = 11.3 (cal cm−3)1/2. | |
For the binary PVDF-HFP/acetone (Ace) system, not only liquid–liquid phase equilibria (LLE), but also solid–liquid phase equilibria (SLE) were calculated because the acetone was used as a processing solvent for fabricating a SPE in this study. Note that both Ace and DME have the same δ1 = 9.9 (cal cm−3)1/2, but they have different molecular weights and densities, distinguishing the phase behavior. Hence, the LLE curve for PVDF-HFP/Ace was slightly different from that of PVDF-HFP/DMC. Furthermore, using eqn (6), i.e., Nish–Wang model, the SLE curve was calculated as shown in Fig. 3a. Here, the parameters used are ΔHu = 104.7 J g−1 × 202 g mol−1 = 21
148.4 J mol−1, Vu = 114 cm3 mol−1,
1 = 74.03 cm3 mol−1, T0m = 143 + 273 = 416 K, and R = 8.314 J mol−1 K−1. Note that PVDF-HFP copolymer has the unit molecular weight of 202 g mol−1 and an enthalpy of fusion, ca. 104.7 J g−1.65,66
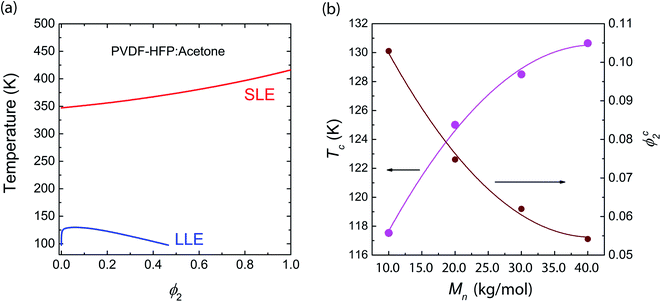 |
| Fig. 3 Phase behavior of PVDF-HFP/Acetone systems: (a) LLE from Flory–Huggins model, and SLE from Nish–Wang model. (b) Critical points (ϕc2,Tc) as a function ofMn. When the number average molecular weight (Mn) is 10.0, 20.0, 30.0, and 40.0 kg mol−1, the relative molar volume (r2) is 76, 153, 209 and 305, respectively. | |
The phase behavior in Fig. 3a suggests that PVDF-HFP may be crystallized out before liquid–liquid (L–L) phase separation if kinetics is sufficiently slow. Furthermore, the critical points (ϕc2,Tc) were calculated for various molecular weights (or chain lengths) of PVDF-HFP (Fig. 3b). The critical point (ϕc2,Tc) is (0.10, 117.53) for 10.0 kg mol−1, (0.08, 125.01) for 20.0 kg mol−1, (0.06, 128.50) for 30.0 kg mol−1, and (0.05, 130.65) for 40.0 kg mol−1, respectively. As shown in Fig. 3b, the slope of curves decreases with increasing Mn, indicating a saturation behavior.
Phase behavior of ternary PVDF-HFP solutions
The Flory–Huggins model (eqn (2)) could be extended for describing the phase behavior of ternary polymer solutions with components, i = 1, 2, 3.43,47 |
 | (7) |
where χij =
1/RT(δi − δj)2 with i or j = 1, 2, 3 from eqn (2). In this work, the components, 1, 2, and 3 correspond to acetone, PVDF-HFP, and PF, respectively. Importantly, ΔGmix in the eqn (7) is expressed ‘per lattice site’.61 However, if one converts ΔGmix into ΔGsysmix = ΔGmix/(n1r1 + n2r2 + n2r2) ‘per the system’ by multiplying (n1r1 + n2r2 + n2r2) in both right- and left-hand sides, the Flory–Huggins model could be expressed as follows,47,61 |
 | (8) |
where niis the number of moles of component i. Now for calculating the phase boundary for ternary systems, the chemical potential (Δμi) of component i should be expressed by differentiating the free energy of mixing in eqn (8) per the system.46,48 |
 | (9) |
|
 | (10) |
|
 | (11) |
where s = v1/v2,r = v1/v3, and s/r = v3/v2. Here, vi is molar volume of component i. The binodal curve could be estimated for ternary systems by extending eqn (2) as below. |
Δμαi = Δμβi (i = 1, 2, 3)
| (12) |
Furthermore, the spinodal curve and the critical point could be calculated according to my previous work.67
For constructing the phase diagram of ternary Ace/PVDF-HFP/PF system, the eqn (12) was employed, enabling the calculation of the phase boundaries. Here, it is noteworthy that the Flory–Huggins theory can consider only van-der-Waals forces in terms of the χ interaction parameter. Thus, it was assumed that PF is a non-ionizable molecule as a model molecule. However, the density and solubility parameter for PF are unknown. Hence, they were estimated through the group contribution method as summarized in Tables 2 and 3.57 Accordingly, the density of PF is 1.74 g cm−3 (=∑Mi/∑Vwi = 257.92/148.35), where Mi (g mol−1) and Vwi (cm3 mol−1) are the mass and van der Waals volume of structural group i, respectively. The molar volume is 987 cm3 mol−1, which was estimated from the relation of Mn/d = 1717/1.74. The unit-molar volume (Vu) is 193 cm3 mol−1 from unit molecular weight divided by density like MWu/d = 336/1.74. Lastly, the solubility parameter of PF was estimated to be 16.6 (cal cm−3)1/2 = 34.1 MPa1/2 by using the Hoftyzer–Van Krevelen method as below.
|
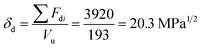 | (13) |
|
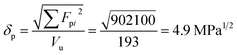 | (14) |
|
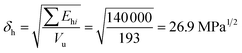 | (15) |
where
Fdi and
Fpi are force contributed from dispersion and polar components, respectively.
Ehi is an energy contributed from hydrogen bonding component. Then, the solubility parameter was finally calculated from the relation,
δ

= 34.1 MPa
1/2was converted to 16.6 (cal cm
−3)
1/2 for estimating the
χ interaction parameter.
Table 2 Group increments of mass and van der Waals volume for polyphenolate (PF)
Structural group |
Number of group |
Mi (g mol−1) |
Mi(cm3 mol−1) |
 |
1 |
76.09 |
43.3 |
–O– |
4 |
16 |
5.5 |
–OH– |
1 |
17 |
8.0 |
–CH2– |
6 |
14.3 |
10.23 |
–CH3 |
1 |
15.03 |
13.67 |
Table 3 Solubility parameter component group contributions (Hoftyzer–Van Krevelen method) for polyphenolate (PF)
Structural group |
Number of group |
Fdi (MJ m−3)1/2 mol−1 |
Fpi (MJ m−3)1/2 mol−1 |
Ehi J mol−1 |
 |
1 |
1270 |
110 |
0 |
–O– |
4 |
100 |
400 |
30 000 |
–OH– |
1 |
210 |
500 |
20 000 |
–CH2– |
6 |
270 |
0 |
0 |
–CH3 |
1 |
420 |
0 |
0 |
Fig. 4 shows the phase diagrams for a ternary Ace/PVDF-HFP/PF system, in which the components 1, 2, and 3 correspond to acetone, PVDF-HFP, and PF, respectively. Here, the physical parameters are χ12 = 0.25,χ13 = 5.61,χ23 = 3.51, s = v1/v2 = 0.001091, and r = v1/v3 = 0.075022 at T = 298 K (Table 4). Resultantly, the phase diagram exhibited the critical point at (ϕc1, ϕc2, ϕc3) = (0.83152, 0.01541, 0.15308), indicating that the phase-separation regions are very large. Specifically, when ϕ1 ≈ 0, the metastable region is from ϕ3 ≈ 0.01223 to 0.14265, whereas the unstable region is from ϕ3 ≈ 0.14265 to 1. Here, it is noteworthy that the nucleation-growth is undergone in a metastable region, whereas the spinodal decomposition proceeds in an unstable region. Hence, the dominant phase-separation process is through the spinodal decomposition in most compositions. Furthermore, two polymers are usually immiscible because of no entropic gain, i.e., ΔGmix = ΔHmix − TΔSmix ≈ ΔHmix ≥ 0. In particular, for the PVDF-HFP/PF blend, χ23 = 3.51 at T = 298 K, whereas the critical interaction parameter (χc23) is
.62 Note that if onlyχ23 < χc23, two polymers are miscible. However, currently, χ23 = 3.51 > χc23 = 0.05, indicating PVDF-HFP and PF are immiscible.
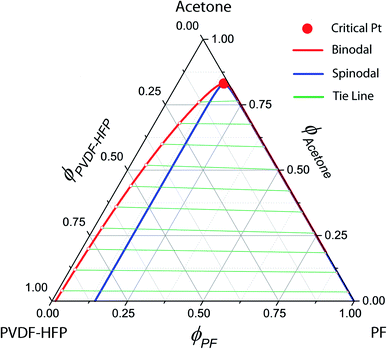 |
| Fig. 4 Phase diagram for the ternary Ace/PVDF-HFP/PF system. T = 298 K; χ12 = 0.25;χ13 = 5.61;χ23 = 3.51; s = 0.001091; r = 0.075022. PVDF-HFP's properties: Mn = 120.0 kg mol; d = 1.77 g cm3; molar volume = 67796.61 cm3 mol; δ = 11.3 (cal cm−3)1/2. PF's properties: Mn = 1.7 kg mol; d = 1.74 g cm3; molar volume = 986.78 cm3 mol; δ = 16.6 (cal cm−3)1/2. Acetone's properties: Molar volume = 74.03 cm3 mol; δ = 9.9 (cal cm−3)1/2. | |
Table 4 Physical parameters for calculating the phase diagrams of ternary Ace/PVDF-HFP/PF systems when T = 298 K,χ12 = 0.25,χ13 = 5.61,χ23 = 3.51, s = 0.001091, and r = 0.075022. Here, MW = molecular weight; d = density; vi = molar volume; and δi = solubility parameter
Materials |
Component i |
MW (g mol−1) |
d (g cm−3) |
vi (cm3 mol−1) |
δi (MPa1/2) |
δi (cal cm−3)1/2 |
Number average molecular weight (Mn). |
Ace |
1 |
58.08 |
0.7845 |
74.03 |
20.3 |
9.9 |
PVDF-HFP |
2 |
120 000a |
1.77 |
67 796.61 |
23.2 |
11.3 |
PF |
3 |
1,717a |
1.74 |
986.78 |
34.1 |
16.6 |
Interestingly, the phase behavior of the Ace/PVDF-HFP/PF system in Fig. 4 is very similar to that of the solvent/polymer/nonsolvent system (e.g., NMP/PVDF-HFP/H2O).53,54 Hence, just like nonsolvent induced phase separation (NIPS), it is expected that PF-induced phase separation takes place in the Ace/PVDF-HFP/PF system because PVDF-HFP and PF are immiscible. Importantly, according to Shi et al., the addition of salts (e.g., LiCl) into this solvent/polymer/nonsolvent system induced the binodal to shift further towards the polymer–solvent axis (i.e., an enlarged phase-separation probability).53 At this moment, it is important to remind that the Flory–Huggins theory cannot deal with coulombic interactions. However, if the ternary Ace/PVDF-HFP/LPF system (here, LPF is ionizable) is considered, the two phenomena are basically expected. One is that the ionization of LPF may increase the entropy of electrolyte system, and the other is that Li+ ions may have a coordination bonding (a weak transient crosslinking) with Lewis base (Florine) in PVDF-HFP, indicating the modified intra-/inter-molecular interactions (i.e., enthalpy) among component molecules. Hence, based on the two thermodynamic (entropic and enthalpic) effects, the phase-separation boundaries should be shifted for adjusting a new equilibrium point.53,54
Single Li-ion conducting solid polymer electrolyte: synthesis, properties and performance
In the backdrop of aforementioned PVDF-HFP thermodynamics, LPF was synthesized and blended with PVDF-HFP using acetone (recall Scheme 1). Fig. 5a shows 1H NMR spectra for PF, i.e., a precursor for LPF, in which ‘–H from benzene, –OH, –CH2–, and –CH3’ were observed at ∼7 ppm, ∼5.5 ppm, ∼4–3.5 ppm, and ∼3.7 ppm, respectively. The molecular weights of PF are about Mn ≈ 1.7 kg mol−1 (equivalent to ∼7 structural units) and Mw ≈ 3.2 kg mol−1, indicating that PDI ≈ 1.9. Here, it is noticeable that the spatial size of PF is close to oligomer, suggesting a partial increase of (ϕ3/r3)
ln
ϕ3 in eqn (8) by reducing the relative molar volume (r3) of PF. In addition, Fig. 6 shows the TGA data for the synthesized PF, displaying the major thermal decomposition at ∼300–400 °C.
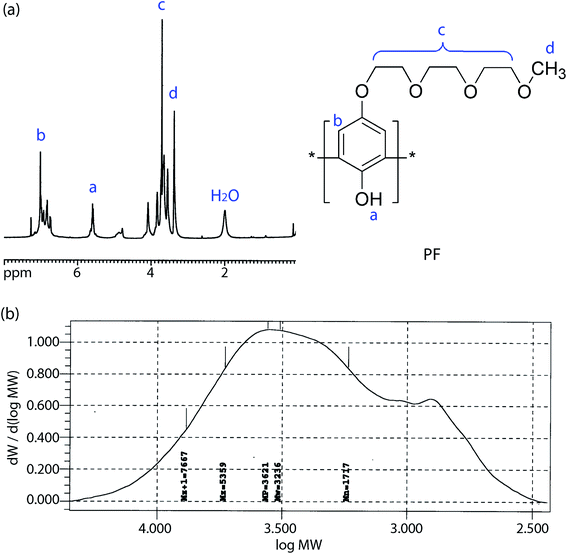 |
| Fig. 5 (a) 1H NMR spectra and chemical structure of PF. (b) GPC data for PF: Mn = 1.7 kg mol−1, Mw = 3.2 kg mol−1, and PDI ≈ 1.9. | |
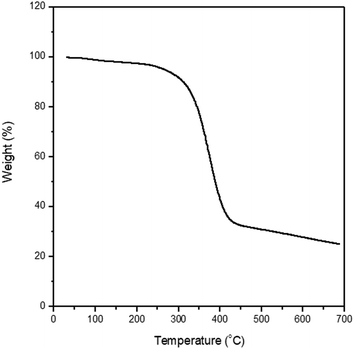 |
| Fig. 6 TGA data for polyphenolate (PF). | |
As a next step, I examined the infrared (IR) spectra for PF and LPF, respectively. As shown in Fig. 7a, –O–H stretching at 3438 cm−1, –C–H stretching at 2881 cm−1, –C
C stretching at 1610 cm−1 and 1500 cm−1, –C–O (phenol) stretching at 1192 cm−1, –C–O–C (ethylene oxide) stretching at 1106 cm−1, and (Bz)–C–O–C stretching at 1004 cm−1 were observed. Interestingly, based on the IR spectra, LPF and PF show a partial difference in the relative intensity (not position) of peaks. Notably, the –O–H stretching was observed at 3438 cm−1 for a hygroscopic LPF sample also, suggesting the H2O absorption during FT-IR measurement in air. However, it is notable that in the case of electrical measurement, the device was fabricated in a glove box under argon environment, not in air.
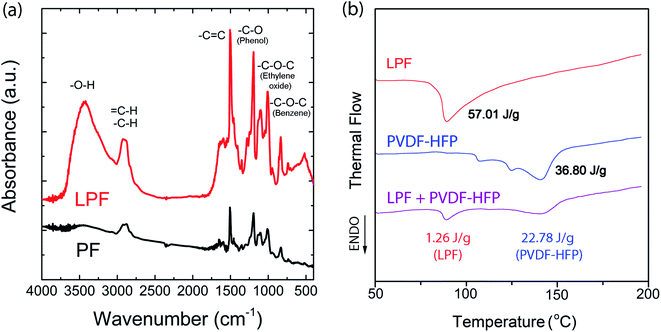 |
| Fig. 7 (a) IR spectra for both PF and LPF. (b) DSC thermograms for LPF, PVDF-HFP, and LPF : PVDF-HFP = 1 : 1.7 (wt ratio) mixture. | |
Fig. 7b shows the DSC thermogram for LPF, PVDF-HFP, and the polymer blend LPF
:
PVDF-HFP = 1
:
1.7, which was recorded during the first heating scans at 10 °C min−1. The melting points (Tm) of the pure components (LPF and PVDF-HFP) were observed at 87 °C and 142 °C, respectively. In the case of LPF
:
PVDF-HFP = 1
:
1.7, the blend exhibited Tm at the same temperature of each components, indicating that PVDF-HFP and LPF are basically immiscible as expected from most two polymers. As shown in Fig. 7b, when blended, the enthalpy was reduced from 57.01 J g−1 to 1.26 J g−1 for LPF, whereas from 38.08 J g−1 to 22.78 J g−1 for PVDF-HFP, indicating the diminished crystallinity (98% reduction for LPF and 38% for PVDF-HFP). Here, if one compares PVDF-HFP and LPF, the crystalline region of PVDF-HFP could survive more than that of LPF when blended each other. Finally, in the case of PVDF-HFP, its ideal enthalpy of melting is 104 J g−1.68 Hence, the crystallinity of pure PVDF-HFP is ca. 35.39% = (36.80/104) × 100, whereas that of PVDF-HFP in the LPF/PVDF-HFP mixture is ca. 21.90% = (22.78/104) × 100.
Fig. 8a shows a typical Nyquist plot of the impedance data for the PVDF-HFP/LPF system at 23 °C, displaying a bulk resistance (Rb), for which the symmetric cell with a SUS/SPE/SSU structure was fabricated. Here, the ionic conductivity (σ) could be estimated from the relation of l/(RbA), where l and A are thickness and area of a film, respectively. For example, when Rb = 514.25 Ω, l = 172 μm, and A = 4 cm2, σ would be 8.4 × 10−6 S cm−1. In the same way, the resulting ionic conductivity as a function of composition is displayed in Fig. 8b. In this range of 1 ≤ wtPVDF-HFP/wtLPF ≤ 5, the average ionic conductivity is σ = 1.4 × 10−5 S cm−1 with the maximum σ = 3.4 × 10−5 S cm−1 at wtPVDF-HFP/wtLPF = 2 and the minimum σ = 6.5 × 10−6 S cm−1 at wtPVDF-HFP/wtLPF = 5. Although there were partial fluctuations in data, the overall trend indicated that the ionic conductivity was enhanced with increasing the LPF amounts in the SPEs. Furthermore, when the experimental data were fitted linearly, the result was y = (2.45 × 10−5) + (−3.56 × 10−6)x with the standard errors (y-intercept: 8.97 × 10−6 S cm−1 and slope: 2.83 × 10−6 S cm−1), in which y and x denote ionic conductivity and weight fraction (wtPVDF-HFP/wtLPF), respectively. On the other hand, it is notable that the ionic conductivity for PEO/LPF was reported to be ∼106 S cm−1 at 23 °C and ∼105 S cm−1 at 100 °C,39 indicating that PVDF-HFP/LPF is superior to PEO/LPF as a polymer electrolyte system. Furthermore, the Li+ ion's transference number (tLi+) for the PVDF-HFP/LPF system was estimated based on the below relation,10,69
|
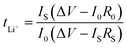 | (16) |
where
I0 and
IS are the initial and steady-state currents under the DC polarization voltage (Δ
V = 10 mV),
R0 and
RS are the initial and steady-state interfacial resistance by the AC impedance method before and after DC polarization. For the PVDF-HFP/LPF system, the AC impedance spectra for the cell were measured before and after DC polarization as shown in
Fig. 7c. Here, during the DC polarization under a constant potential of 10 mV, the current response was measured for the Li/SPE/Li cell as a function of time as shown in
Fig. 7d. Resultantly,
tLi+ ≈ 0.8 was estimated using
eqn (16) with the values of Δ
V = 0.01 V,
I0 = 3.2269 × 10
−5 A,
IS = 1.2063 × 10
−5 A,
R0 = 1
![[thin space (1/6-em)]](https://www.rsc.org/images/entities/char_2009.gif)
015
![[thin space (1/6-em)]](https://www.rsc.org/images/entities/char_2009.gif)
915–49 = 1
![[thin space (1/6-em)]](https://www.rsc.org/images/entities/char_2009.gif)
015
![[thin space (1/6-em)]](https://www.rsc.org/images/entities/char_2009.gif)
866 Ω, and
RS = 1
![[thin space (1/6-em)]](https://www.rsc.org/images/entities/char_2009.gif)
275
![[thin space (1/6-em)]](https://www.rsc.org/images/entities/char_2009.gif)
458–49 = 1
![[thin space (1/6-em)]](https://www.rsc.org/images/entities/char_2009.gif)
275
![[thin space (1/6-em)]](https://www.rsc.org/images/entities/char_2009.gif)
409 Ω, which is a promising result as a single-ion conducting SPE. However, it is notable that
tLi+ is only 0.8 below the ideal ∼1.0, indicating that 20% of electricity was transferred through anions. Hence, although minus charges were embedded in the oligomeric polyphenolate with PDI ∼ 1.9, some anions (relatively smaller molecules among polydisperse LPFs) may migrate under the electric field. This phenomenon suggests that for increasing
tLi+, the minus charges should be well fixed on the macromolecular polyelectrolyte. More importantly, although many research groups have reported
tLi+ at high temperature (
e.g., ∼60–90 °C),
70–74 here I reported
tLi+ at 23 °C. This observation suggests that the polar polymer PVDF-HFP is an effective matrix for ionic conductor applications.
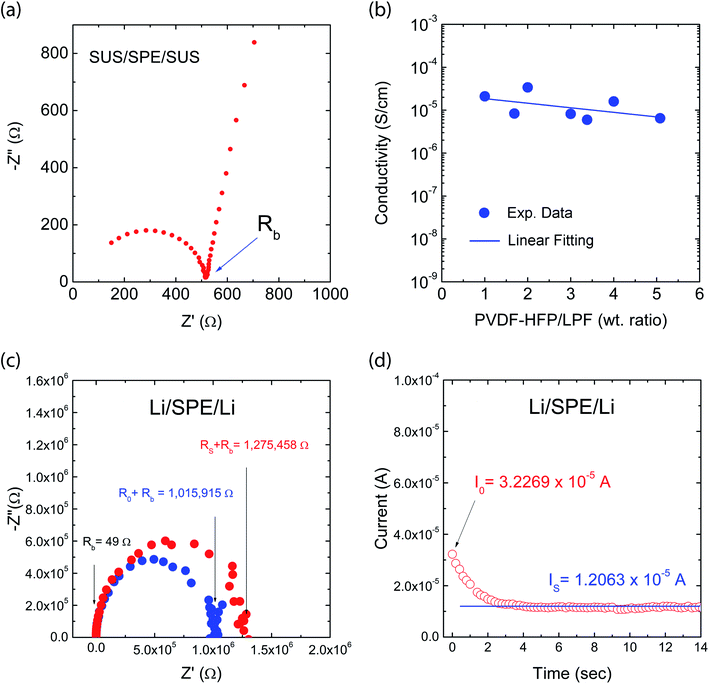 |
| Fig. 8 Ionic conductivity: (a) Example of Nyquist plot. (b) Ionic conductivity as a function of composition for a single Li-ion conducting solid polymer electrolyte based on PVDF-HFP and LPF mixtures. Li transference number: (c) The AC impedance spectra for the cell before and after DC polarization at 23 °C. (d) The current response for Li/SPE/Li cell as a function of time during DC polarization under a constant potential of 10 mV at 23 °C. | |
Finally, although the research theme of mine was in the development of solid-state polymer electrolyte, I carried out the preliminary study about the filler and plasticizer effect on the ionic conductivity. Resultantly, when the inorganic filler SiO2 was added ∼25 wt% of LPF, the ionic conductivity (LPF
:
PVDF-HFP
:
SiO2 = 1
:
1.7
:
0.25) was improved up to ∼1.03 × 10−4 S cm−1 (compared to average 1.4 × 10−5 S cm−1 without filler). Furthermore, when a plasticizer (EC
:
PC = 1
:
1) was added into the above system like LPF
:
PVDF-HFP
:
SiO2:EC
:
PC = 1
:
1.7
:
0.25
:
0.5
:
0.5, the ionic conductivity was similarly ∼1.0–1.1 × 10−4 S cm−1 (see Table 5). This indicates that there is a trade-off relationship between the flexibility (increase of chain motion) and dilution (decrease of carrier concentrations per volume) of electrolyte system. Hence, the ionic conductivity was ∼10−4 S cm−1 in the case of the aforementioned gel polymer electrolyte (GPE).
Table 5 Ionic conductivity of each polymer electrolyte at 23 °C
|
Polymer electrolyte (weight ratio) |
LPF : PEO = 1 : 3.57a |
LPF : PVDF-HFP = 1 : 1.7 |
LPF : PVDF-HFP : SiO2 = 1 : 1.7 : 0.25 |
LPF : PVDF-HFP : SiO2 : EC : PC = 1 : 1.7 : 0.25 : 0.5 : 0.5 |
LPF : PEO = 1 : 3.57 (wt ratio) indicates that the 20 repeat units of PEO per repeat unit of LPF.21 |
σ (S cm−1) |
∼10−6 |
∼3.4 × 10−5 |
∼1.0 × 10−4 |
∼1.1 × 10−4 |
Conclusion
When the highly polar PVDF-HFP fluoropolymer was blended with a polyelectrolyte lithium polyphenolate (LPF) for single-ion conductor applications, the ionic conductivity was ∼10−5 S cm−1 in solid state and ∼10−4 S cm−1 in gel state. More importantly, this LPF/PVDF-HFP single-ion conductor displayed a lithium transference number of ca. 0.8 at 23 °C, indicating that 20% of charges were transported through the anions fixed in the oligomeric polydisperse polyphenolate. Finally, considering the limited thermodynamic studies on the phase behavior of PVDF-HFP solutions and blends, I believe this work should be a significant progress, providing the insight for the phase behavior of PVDF-HFP solutions and blends based on the classical Flory–Huggins lattice theory.
Conflicts of interest
The authors declare no competing financial interest.
Acknowledgements
Advanced Materials Science and Engineering Center of Excellence at Adama Science and Technology University is acknowledged for supporting research in the thematic area of Polymer Materials.
References
- A. C. Jayasuriya, A. Schirokauer and J. I. Scheinbeim, J. Polym. Sci., Part B: Polym. Phys., 2001, 39, 2793–2799 CrossRef CAS.
- R. E. Sousa, J. Nunes-Pereira, J. C. C. Ferreira, C. M. Costa, A. V. Machado, M. M. Silva and S. Lanceros-Mendez, Polym. Test., 2014, 40, 245–255 CrossRef CAS.
- J. Y. Song, Y. Y. Wang and C. C. Wan, J. Electrochem. Soc., 2000, 147, 3219–3225 CrossRef CAS.
- T. S. Ahmed, J. M. DeSimone and G. W. Roberts, Macromolecules, 2007, 40, 9322–9331 CrossRef CAS.
- B. Ameduri, Chem. Rev., 2009, 109, 6632–6686 CrossRef CAS PubMed.
- J. Barbosa, J. Dias, S. Lanceros-Méndez and C. Costa, Membranes, 2018, 8, 45 CrossRef PubMed.
- J.-M. Tarascon, A. S. Gozdz, C. Schmutz, F. Shokoohi and P. C. Warren, Solid State Ionics, 1996, 86–88, 49–54 CrossRef CAS.
- P. Zhang, R. Li, J. Huang, B. Liu, M. Zhou, B. Wen, Y. Xia and S. Okada, RSC Adv., 2021, 11, 11943 RSC.
- K. Huang, Y. Wang, H. Mi, D. Ma, B. Yong and P. Zhang, J. Mater. Chem. A, 2020, 8, 20593–20603 RSC.
- T. S. Mengistie, J. M. Ko and J. Y. Kim, Mater. Res. Express, 2021, 8, 035308 CrossRef CAS.
- J. Li, L. Zhu, J. Zhang, M. Jing, S. Yao, X. Shen, S. Li and F. Tu, Int. J. Energy Res., 2021, 45, 7663–7674 CrossRef CAS.
- K. Elamina, M. Shojaatalhosseini, O. Danyliv, A. Martinelli and J. Swenson, Electrochim. Acta, 2019, 299, 979–986 CrossRef.
- P. Pradeepa, S. Edwinraj, G. Sowmya, J. Kalaiselvimary, K. Selvakumar and M. R. Prabhu, AIP Conf. Proc., 2016, 1728, 020397 CrossRef.
- S. Das and A. Ghosh, J. Phys. Chem. B, 2017, 121, 5422–5432 CrossRef CAS PubMed.
- X. Wang, Z. Liu, Y. Tang, J. Chen, Z. Mao and D. Wang, Solid State Ionics, 2021, 359, 115532 CrossRef CAS.
- K. Mishra, A. Garg, R. Sharma, R. Gautam and S. S. Pundir, Mater. Today: Proc., 2019, 12, 621–627 CAS.
- M. Ulaganathan and S. Rajendran, J. Appl. Polym. Sci., 2010, 118, 646–651 CAS.
- N. H. Basri, S. Ibrahim and N. S. Mohamed, Adv. Mater. Res., 2011, 287–290, 100–103 CAS.
- Z. He, Q. Cao, B. Jing, X. Wang and Y. Deng, RSC Adv., 2017, 7, 3240–3248 RSC.
- Z.-l. Wang and Z.-y. Tang, Electrochim. Acta, 2004, 49, 1063–1068 CrossRef CAS.
- D. Mouraliraman, N. Shaji, S. Praveen, M. Nanthagopal, C. W. Ho, M. V. Karthik, T. Kim and C. W. Lee, Nanomaterials, 2022, 12, 1056 CrossRef CAS PubMed.
- P. Yadav, S. H. Beheshti, A. R. Kathribail, P. Ivanchenko, J. V. Mierlo and M. Berecibar, Polymers, 2022, 14, 363 CrossRef CAS PubMed.
- Z. Ruan, Y. Du, H. Pan, R. Zhang, F. Zhang, H. Tang and H. Zhang, Polymers, 2022, 14, 1950 CrossRef CAS PubMed.
- H.-P. Liang, M. Zarrabeitia, Z. Chen, S. Jovanovic, S. Merz, J. Granwehr, S. Passerini and D. Bresser, Adv. Energy Mater., 2022, 2200013 CrossRef CAS.
- H. Guo, S. Zhong, L. Chen, G. Peng, F. F. Wang, T. T. Yan and J. Hu, Int. J. Electrochem. Sci., 2022, 17, 220145 CrossRef CAS.
- Y. Wu, Y. Li, Y. Wang, Q. Liu, Q. Chen and M. Chen, J. Energy Chem., 2022, 64, 62–84 CrossRef.
- J. W. Choi and D. Aurbach, Nat. Rev., 2016, 1, 16013 CAS.
- D. Zhou, D. Shanmukaraj, A. Tkacheva, M. Armand and G. Wang, Chem, 2019, 5, 2326–2352 CAS.
- A. Ponrouch and M. R. Palacin, Philos. Trans. R. Soc., A, 2019, 377, 20180297 CrossRef CAS PubMed.
- M. Walter, M. V. Kovalenko and K. V. Kravchyk, New J. Chem., 2020, 44, 1677–1683 RSC.
- F. Duffner, N. Kronemeyer, J. Tübke, J. Leker, M. Winter and R. Schmuch, Nat. Energy, 2021, 6, 123–234 CrossRef CAS.
- J. Popovic, Macromol. Chem. Phys., 2022, 223, 2100344 CrossRef CAS.
- X. Wang, C. Zhang, M. Sawczyk, Q. Yuan, F. Chen, T. C. Mendes, P. C. Howlett, C. Fu, D. J. Searles, P. Král, C. J. Hawker, A. K. Whittaker and M. Forsyth, Nat. Mater., 2022 DOI:10.1038/s41563-022-01296-0.
- M. S. Grewal, M. Tanaka and H. Kawakami, Macromol. Chem. Phys., 2022, 223, 2100317 CrossRef CAS.
- Y. Liu, Q. Zheng, P. Chen, Z. Li, A. Chen, J. Guan, A. Wang and L. Zhang, Macromol. Chem. Phys., 2022, 223, 2100325 CrossRef CAS.
- J. Li, J. Qi, F. Jin, F. Zhang, L. Zheng, L. Tang, R. Huang, J. Xu, H. Chen, M. Liu, Y. Qiu, A. I. Cooper, Y. Shen and L. Chen, Nat. Commun., 2022, 13, 2031 CrossRef CAS PubMed.
- Y. Wang, C. J. Zanelotti, X. Wang, R. Kerr, L. Jin, W. H. Kan, T. J. Dingemans, M. Forsyth and L. A. Madsen, Nat. Mater., 2021, 20, 1255–1263 CrossRef CAS PubMed.
- M. Sun, Z. Zeng, W. Zhong, Z. Han, L. Peng, C. Yu, S. Cheng and J. Xie, J. Mater. Chem. A, 2022, 10, 11732–11741 RSC.
- B. K. Mandal, C. J. Walsh, T. Sooksimuang and S. J. Behroozi, Chem. Mater., 2000, 12, 6–8 CrossRef CAS.
- K. Se, K. Adachi and T. Kotaka, Polym. J., 1981, 13, 1009–1017 CrossRef CAS.
- D. Zhang, L. Li, X. Wu, J. Wang, Q. Li, K. Pan and J. He, Front. Energy Res., 2021, 9, 726738 CrossRef.
- Z. Xue, D. He and X. Xie, J. Mater. Chem. A, 2015, 3, 19218–19253 RSC.
- H. Tompa, Trans. Faraday Soc., 1949, 45, 1142–1152 RSC.
- R. A. Scott, J. Chem. Phys., 1949, 17, 279–284 CrossRef CAS.
- P. J. Flory, Principles of Polymer Chemistry, Cornell University Press, Ithaca, New York, 1953 Search PubMed.
- F. W. Altena and C. A. Smolders, Macromolecules, 1982, 15, 1491–1497 CrossRef CAS.
- L. Yilmaz and A. J. McHugh, J. Appl. Polym. Sci., 1986, 31, 997–1018 CrossRef CAS.
- C. C. Hsu and J. M. Prausnitz, Macromolecules, 1974, 7, 320–324 CrossRef CAS.
- P. van de Witte, P. J. Dijkstra, J. W. A. van den Berg and J. Feijen, J. Membr. Sci., 1996, 117, 1–31 CrossRef CAS.
- X. Wang, L. Zhang, D. Sun, Q. An and H. Chen, Desalination, 2009, 236, 170–178 CrossRef CAS.
- J. Heo, Y. Choi, K. Y. Chung and J. H. Park, J. Mater. Chem. A, 2016, 4, 9496–9501 RSC.
- J. Wang, L. Zheng, Z. Wu, Y. Zhang and X. Zhang, J. Membr. Sci., 2016, 497, 183–193 CrossRef CAS.
- L. Shi, R. Wang, Y. Cao, D. T. Liang and J. H. Tay, J. Membr. Sci., 2008, 315, 195–204 CrossRef CAS.
- L. Zheng, J. Wang, D. Yu, Y. Zhang and Y. Wei, J. Membr. Sci., 2018, 550, 480–491 CrossRef CAS.
- J. Y. Kim, Macromolecules, 2019, 52, 4317–4328 CrossRef CAS.
- J. Y. Kim, Macromolecules, 2018, 51, 9026–9034 CrossRef CAS.
- D. W. Van Krevelen and K. te Nijenhuis, Properties of Polymers, Elsevier Science Ltd, Amsterdam, Netherlands, fourth edn, 2009 Search PubMed.
- L. Stolz, S. Hochstädt, S. Röser, M. R. Hansen, M. Winter and J. Kasnatscheew, ACS Appl. Mater. Interfaces, 2022, 14, 11559–11566 CrossRef CAS PubMed.
- J. Zhu, Z. Zhang, S. Zhao, A. S. Westover, I. Belharouak and P.-F. Cao, Adv. Energy Mater., 2021, 11, 2003836 CrossRef CAS.
- M. Kumar and S. S. Sekhon, Eur. Polym. J., 2002, 38, 1297–1304 CrossRef CAS.
- T. P. Lodge and P. C. Hiemenz, Polymer Chemistry, CRC Press, Boca Raton, Florida, third edn, 2021 Search PubMed.
- T. Nishi and T. T. Wang, Macromolecules, 1975, 8, 909–915 CrossRef CAS.
- S. Wongchitphimon, R. Wang, R. Jiraratananon, L. Shi and C. H. Loh, J. Membr. Sci., 2011, 369, 329–338 CrossRef CAS.
- M. Belmares, M. Blanco, W. A. Goddard 3rd, R. B. Ross, G. Caldwell, S.-H. Chou, J. Pham, P. M. Olofson and C. Thomas, J. Comput. Chem., 2004, 30, 1814–1826 CrossRef PubMed.
- K. Nakagawa and Y. Ishida, J. Polym. Sci., Part B: Polym. Phys., 1973, 11, 2153–2171 CAS.
- J.-H. Cao, B.-K. Zhu and Y.-Y. Xu, J. Membr. Sci., 2006, 281, 446–453 CrossRef CAS.
- J. Y. Kim, Polymers, 2021, 13, 983 CrossRef CAS PubMed.
- K. Nakagawa and Y. Ishida, J. Polym. Sci., Part B: Polym. Phys., 1973, 11, 2153–2171 CAS.
- J. Evans, C. A. Vincent and P. G. Bruce, Polymer, 1987, 28, 2324–2328 CrossRef CAS.
- S. Feng, D. Shi, F. Liu, L. Zheng, J. Nie, W. Feng, X. Huang, M. Armand and Z. Zhou, Electrochim. Acta, 2013, 93, 254–263 CrossRef CAS.
- R. Bouchet, S. Maria, R. Meziane, A. Aboulaich, L. Lienafa, J.-P. Bonnet, T. N. T. Phan, D. Bertin, D. Gigmes, D. Devaux, R. Denoyel and M. Armand, Nat. Mater., 2013, 12, 452–457 CrossRef CAS PubMed.
- Q. Ma, H. Zhang, C. Zhou, L. Zheng, P. Cheng, J. Nie, W. Feng, Y.-S. Hu, H. Li, X. Huang, L. Chen, M. Armand and Z. Zhou, Angew. Chem., 2016, 55, 2521–2525 CrossRef CAS PubMed.
- Q. Ma, Y. Xia, W. Feng, J. Nie, Y.-S. Hu, H. Li, X. Huang, L. Chen, M. Armand and Z.-B. Zhou, RSC Adv., 2016, 6, 32454–32461 RSC.
- L. Porcarelli, A. S. Shaplov, M. Salsamendi, J. R. Nair, Y. S. Vygodskii, D. Mecerreyes and C. Gerbaldi, ACS Appl. Mater. Interfaces, 2016, 8, 10350–10359 CrossRef CAS PubMed.
|
This journal is © The Royal Society of Chemistry 2022 |
Click here to see how this site uses Cookies. View our privacy policy here.