DOI:
10.1039/D2RA03966D
(Paper)
RSC Adv., 2022,
12, 21417-21421
Ring-opening of non-activated aziridines with [11C]CO2 via novel ionic liquids†
Received
27th June 2022
, Accepted 14th July 2022
First published on 2nd August 2022
Abstract
Novel ionic liquids based on DBU and DBN halide salts were developed as a catalytic system for ring-opening of non-activated aziridines with [11C]CO2. The ability of ionic liquids to activate aziridines represents a simple methodology for the synthesis of 11C-carbamates and can be extended for CO2-fixation in organic and radiochemistry.
Introduction
Positron emission tomography (PET) is a dynamic functional imaging technique used to study biochemical interactions in vivo with extensive applications in clinical trials and drug development. PET utilizes positron emitting radionuclides, frequently, carbon-11 (11C, t1/2 = 20.4 min) and fluorine-18 (18F, t1/2 = 109.7 min), which are incorporated into a biologically active molecule (radiotracers) that are injected in vivo and used to generate a tomographic image.1 Due to the relatively short half-life of 11C, radiochemical reactions with this radionuclide must be fast and efficient. Cyclotron-produced 11C is generated in target by the 14N(p,α)11C nuclear reaction as either [11C]CO2 or [11C]CH4 for conversion to [11C]CH3I or [11C]CH3OTf and applied for 11C-methylation reactions. More recently 11C-carbonylation reactions are being applied for clinical PET research studies, where [11C]CO2 is used directly or converted to [11C]CO, [11C]COCl2 or [11C]HCN.2 While recent developments of [11C]CO reactions have been made,3 its use is still limited by the poor solubility in organic solvents, low reactivity and often need for pressurized reactors. The conversion of [11C]CO2 to [11C]CO is relatively time consuming and inefficient. [11C]COCl2 is more reactive than [11C]CO for 11C-carbonylation reactions, however its production requires specialized equipment and use of chlorine gas.4 11C-carbonylation can also be achieved using [11C]HCN, but is limited primarily to 11C-carboxylic acids and primary 11C-amides.5 Using [11C]CO2 directly for 11C-carbonylation removes the need for converting the cyclotron produced [11C]CO2 into other C1 building blocks and can be used directly for the syntheses of a wide range of 11C-carbonyl functionalities.6
[11C]CO2 fixation reactions have gained widespread use for 11C-carbonylation of PET radiotracers,7 and are often achieved by trapping [11C]CO2 directly from the cyclotron in a bulky organic base, such as 2-tert-butylimino-2-diethylamino-1,3-dimethylperhydro-1,3,2-diazaphosphorine (BEMP), 1,8-diazabicyclo[5.4.0]undec-7-ene (DBU) or 1,5-diazabicyclo[4.3.0]non-5-ene (DBN) under ambient conditions. This method of [11C]CO2 fixation been successfully utilized to generate 11C-carbamates in high radiochemical yields (Fig. 1A),8–11 including [11C]CURB and [11C]SL25.1188 that were developed and translated for clinical PET research by our laboratories (Fig. 1A entries 3 and 4).12,13
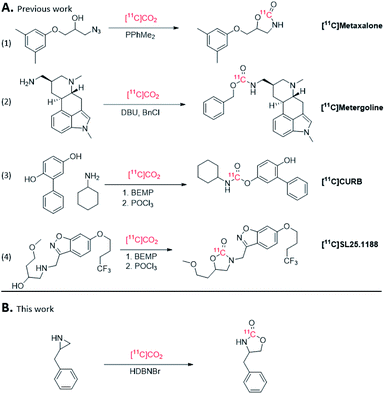 |
| Fig. 1 (A) Previous syntheses of 11C-carbamates using [11C]CO2. (B) This work. | |
The use of ionic liquids (ILs)14–19 as a catalytic system for trapping of CO2 from the atmosphere has been reported in environmental chemistry20 and organic synthesis. For example, IL-catalysed CO2 insertion by ring-opening of aziridines or epoxides21 have been explored to form cyclic carbamates and carbonates, respectively.22–26 Inserting CO2 into epoxides to form cyclic carbonates, such as dioxolan-2-ones, have been shown to be an efficient way to bind atmospheric CO2.26 However, the resulting cyclic carbonates are prone to ring-open in aqueous conditions.27 However, CO2 insertion into aziridines forms oxazolidine-2-ones, which are a common motif in pharmaceuticals as well as [11C]SL2511.88 (Fig. 1A), and has been achieved by ring-opening aziridines with CO2 using metal or organic halides.22 Relevant to [11C]CO2 fixation, ILs based on halide salts derived from DBU have been reported for ring-opening of epoxides with CO2.28 While ILs have been employed for 18F-radiochemistry,29,30 to our knowledge, ILs have not been explored in 11C- radiochemistry.
Ring-opening of activated aziridines and epoxides have been reported in PET radiochemistry using both 11C and 18F nucleophiles, namely, [11C]HCN and [18F]fluoride.31–35 In the present study, we describe the application of ILs, including novel DBU and DBN halide salts, to assess their ability to trap [11C]CO2 and ring-open aziridines to form cyclic [11C]carbamates (Fig. 1B).
Methods
No-carrier added [11C]CO2 was produced on a MC-17 cyclotron (Scanditronix, Sweden) using the 14N(p,α)11C nuclear reaction in a pressurized gas target contain a nitrogen
:
oxygen mixture (99.5
:
0.5). The radiosynthesis was performed using a TracerMaker™ carbon-11 synthesis platform (Scansys Laboratorieteknik, Denmark). Analytical HPLC was performed on a 1260 Infinity II HPLC system (Agilent, CA) with a M177 γ-radiation detector (Ludlum Instruments, TX) connected in series after the UV detector. Radiochemistry was performed in lead-shielded hot cells in a laboratory designed to handle radioactive material. The work was performed in accordance with the radiation protection guidelines and regulations from the Canadian Nuclear Safety Commission and internal safety policies and procedures.
Chemistry
Synthesis of HDBU and HDBN halide salts were performed according to a previously reported method.28 DBU/DBN (20 mmol) and ammonium halide (20 mmol) was taken up in methanol (50 mL) and refluxed overnight. Volatiles were removed in vacuo to give the desired IL as a solid (see ESI† for details).
Radiochemistry
[11C]CO2 was first trapped on a HayeSep D column (700 mg) cooled to −180 °C using liquid nitrogen on a TracerMaker™ synthesis platform. Upon heating the HayeSep D column, [11C]CO2 was released and carried by helium gas to a reaction vessel pre-charged with IL (10–20 mg), aziridine (5 mg) and NMP (200 μL), heated at 85 °C for 15 min under argon atmosphere prior to addition of [11C]CO2. After maximum radioactivity in the reaction vessel was reached, the transfer lines were removed and the reaction was heated for 5 min. An ascarite trap was connected to the outlet line from the vial during transfer from the HayeSep D column to trap unreacted [11C]CO2. After completion of the reaction, the gas in the headspace of the reaction vessel was vented through the same ascarite trap. [11C]CO2 trapping efficiency (TE) was calculated as the amount of activity in the reaction mixture after venting compared to the amount on the ascarite trap. Radiochemical conversion (RCC) was subsequently measured by radio-HPLC from an aliquot of the crude reaction mixture and calculated as the amount of desired product compared to untrapped [11C]CO2 and any byproducts in the radio-HPLC chromatogram.
Results and discussion
Ring-opening of benzylaziridine with [11C]CO2 to produce [11C]benzyloxazolidin-2-one ([11C]1) using tetrabutylammonium bromide (TBABr) and NMP was used as a starting point for reaction optimization (Table 1). Reactions in the absence of an activation step were carried out at 130 °C for 5 min, and only reached a maximum TE of 24% to produce [11C]1 with a RCC of 24%. Introduction of an activation step prior to [11C]CO2 addition proved to be effective at increasing the TE. By heating benzylaziridine and the TBABr mixture to 85 °C for 15 min prior to adding [11C]CO2, the TE increased to 45% with a higher RCC of 41% (Table 1, entry 1). Reducing the reaction time to 1 min lowered the overall RCC to 37% and decreasing the reaction temperature to 70 °C or ambient temperature lowered the RCC to 29% and 11%, respectively (entries 2–4). 1-Butyl-3-methylimidazolium bromide (BMIMBr), a commercially available quaternary ammonium IL that has been applied for ring-opening of epoxides with CO2,26 resulted in a lower TE and RCC (28% and 27%, respectively; entry 5). Lithium bromide has also been used in ring-opening reactions with CO2 on epoxides and aziridines, however, the poor solubility of LiBr in NMP is attributed to the low overall RCC (3%) in this model reaction (entry 6), despite having similarly effective TE as achieved using TBABr.
Table 1 TEs and RCCs for the radiosynthesis of [11C]benzyloxazolidin-2-one from benzylaziridine and [11C]CO2 using ILsa
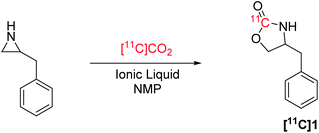
|
Entry |
Ionic liquid/salt |
Amount |
TE |
RCC |
General conditions, with IL (10–20 mg), aziridine (5 mg) and NMP (200 μL), activated at 85 °C for 15 min under argon. Reaction time 5 min at 130 °C. * 1 min reaction time, ** 70 °C reaction temperature, *** ambient reaction temperature. |
1 |
TBABr |
10 mg |
45% |
41% |
2 |
TBABr* |
10 mg |
38% |
37% |
3 |
TBABr** |
10 mg |
30% |
29% |
4 |
TBABr*** |
10 mg |
13% |
11% |
5 |
BMIMBr |
10 mg |
28% |
27% |
6 |
LiBr |
10 mg |
45% |
3% |
7 |
TBABr (BEMP) |
10 mg |
99% |
— |
8 |
LiBr (DBU) |
10 mg |
99% |
— |
9 |
TBABr (DBU) |
10 mg |
98% |
8% |
10 |
HDBUI |
10 mg |
53% |
50% |
11 |
HDBUBr |
10 mg |
69% |
67% |
12 |
HDBUCl |
10 mg |
59% |
57% |
13 |
HDBNI |
10 mg |
55% |
54% |
14 |
HDBNI |
20 mg |
64% |
63% |
15 |
HDBNCl |
20 mg |
68% |
64% |
16 |
HDBNBr |
20 mg |
78% |
77% |
Traditional bases used in [11C]CO2 fixation, namely BEMP or DBU, increased the TE to 99% but were not suitable for forming the ring-opened product, [11C]1 (entries 7–9). It is postulated that the fixating agents bind [11C]CO2 without coordinating to the aziridine and therefore restricts the [11C]CO2 insertion reaction, whilst allowing for high TE. HDBUI, which has been reported as an effective IL for opening epoxides with CO2,28 proved to also be effective for ring-opening benzylaziridine with [11C]CO2 and showed TE of 50% with RCC of 46% (entry 10). Analogues of HDBUI were subsequently investigated. Changing the anion from iodide to bromide gave TE of 69% and RCC of 67% (entry 11). However, using chloride as the anion gave TE of 59% and RCC of 57% (entry 12). Use of DBN instead of DBU as the cation and iodide as the anion gave TE of 55% and RCC of 54% (entry 13). Increasing the amount of IL used from 10 mg to 20 mg resulted in 64% TE and 63% overall RCY using HDBNI (entry 14). The chloride and bromide salts of DBN were synthesized and resulted in improved TE of 68% and RCC of 64% using HDBNCl (entry 15). The optimized synthesis of [11C]1 was achieved using HDNBr and resulted in a TE of 78% with RCC of 77% (entry 16).
Only one product resulted in all experiments despite the potential for benzyl aziridine to ring-open via two different pathways to form either [11C]4-benzyloxazolidin-2-one or [11C]5-benzyloxazolidin-2-one. The absolute enantiomeric configuration of the product was confirmed by performing the reaction with CO2 instead of [11C]CO2. The reaction yielded only one product which co-eluted with the radiochemical product by HPLC analysis (see ESI† for details). 1H- and 13C-NMR spectroscopic analysis comparing the synthesized product with a sample of authentic 5-benzyloxazolidin-2-one confirmed that both the radiochemical and chemical product was 4-benzyloxazolidin-2-one.
With the optimized conditions (Table 1, entry 16) using HDBNBr and 15 min activation at 85 °C prior to addition of [11C]CO2 followed by 5 min reaction at 130 °C, the substrate scope of this reaction was evaluated with commercially available aziridines (Table 2). N-Tosylated aziridines (activated aziridines) did not yield a 11C-carbamate product. However, the same reaction conditions applied to non-activated N-protonated and N-alkylated azirdines resulted in 11C-carbamates as the products. Four aziridines were successfully labelled with [11C]CO2 using HDBNBr as IL. Only one radiochemical product was detected in all reactions. Performing the reaction with the spirocyclic precursor 1-azaspiro[2.5]octane (Table 2, entry 1) resulted in 50% TE and 5% RCC of [11C]oxa-azaspirodecan-2-one ([11C]2). 1-Methyl-2-phenylaziridine was converted to [11C]phenyloxazolidin-2-one ([11C]3) with 55% TE and 46% RCC. 2,2-Dimethylaziridine produced [11C]dimethyloxazolidin-2-one ([11C]4) with 74% TE and 63% RCC. 1-Azabicyclo[3.1.0]hexane gave [11C]tetrahydro-1H,3H-pyrrolo[1,1-c]oxazol-3-one ([11C]5) with 95% TE and RCC. The relatively low RCC for [11C]2 are attributed to the steric hindrance by the conformation of the intermediate forming a stable “chair” configuration thus making the formation of a five-membered oxazolidinone unfavourable (Table 2, entry 1). A methylated aziridine was tolerated in this method (Table 2, entry 2). Dimethylaziridne showed TE and RCC comparable to benzylaziridine (Table 2, entry 3). The TE and RCC for formation of the bicyclic [11C]5 were the highest seen in this investigation and could be explained by the reaction mechanism which goes through an intermediate that releases the structural tension of the precursor and favours the formation of the bicyclic product [11C]5 (Table 2, entry 4).
Table 2 TEs and RCCs for radiosynthesis of [11C]2–[11C]6 using HDBNBr. Absolute configuration of the ring-opened product was not determined. All values are averages of 2 experiments
Entry |
Aziridine |
TE |
RCC |
Product |
1 |
 |
50% |
5% |
[11C]2 |
2 |
 |
55% |
46% |
[11C]3 |
3 |
 |
74% |
63% |
[11C]4 |
4 |
 |
95% |
95% |
[11C]5 |
5 |
 |
76% |
1% |
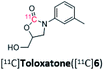 |
Toloxatone is a monoamine oxidase A inhibitor that has been synthesized from (1-(m-tolyl)aziridin-2-yl)methanol in an analogous aziridine ring-opening reaction, albeit using CO2 and metal catalysis.36 The radiosynthesis of [11C]toloxotone in our study ([11C]6; Table 2, entry 5) resulted with a TE of 76% and three radiochemical products were detected in the reaction mixture by radio-HPLC (Fig. S6†). The desired product ([11C]6) corresponded to ca. 1% RCC. Further optimization to improve the RCC were attempted but unsuccessful, and may be due to the alternative enantiomeric ring-opening reaction occurring. Due to the low RCC, isolation of [11C]6 was not possible and therefore the molar activity was not determined.
Conclusions
Ionic liquids are demonstrated as an effective catalytic system for the radiosynthesis of 11C-carbamates by ring-opening of non-activated aziridines with [11C]CO2. Novels ILs based on DBN were synthesized herein and used to efficiently synthesize [11C]4-benzyloxazolidinone. This methodology was successfully used to ring-open four other non-activated aziridines to form 11C-carbamates. ILs are under exploration for further use with aziridines, epoxides in organic and radiochemistry.
Author contributions
AL: conceptualization, synthesis, data analysis, wrote and edited the manuscript; NV: data analysis and edited the manuscript.
Conflicts of interest
The authors declare no relevant conflicts of interest.
Acknowledgements
The authors thank members of the Azrieli Centre for Neuro-Radiochemistry and Brain Health Imaging Centre for their support.
References
- X. Y. Deng, J. Rong, L. Wang, N. Vasdev, L. Zhang, L. Josephson and S. H. Liang, Angew. Chem., Int. Ed., 2019, 58, 2580–2605 CrossRef CAS PubMed.
- B. H. Rotstein, S. H. Liang, M. S. Placzek, J. M. Hooker, A. D. Gee, F. Dolle, A. A. Wilson and N. Vasdev, Chem. Soc. Rev., 2016, 45, 4708–4726 RSC.
- C. Taddei and A. D. Gee, J. Labelled Compd. Radiopharm., 2018, 61, 237–251 CrossRef CAS PubMed.
- Y. Bramoulle, D. Roeda and F. Dolle, Tetrahedron Lett., 2010, 51, 313–316 CrossRef CAS.
- Y. P. Zhou, K. J. Makaravage and P. Brugarolas, Nucl. Med. Biol., 2021, 102, 56–86 CrossRef PubMed.
- C. Taddei and V. W. Pike, EJNMMI Radiopharmacy and Chemistry, 2019, 4, 25 CrossRef PubMed.
- B. H. Rotstein, S. H. Liang, J. P. Holland, T. L. Collier, J. M. Hooker, A. A. Wilson and N. Vasdev, Chem. Commun., 2013, 49, 5621–5629 RSC.
- A. Del Vecchio, A. Talbot, F. Caille, A. Chevalier, A. Sallustrau, O. Loreau, G. Destro, F. Taran and D. Audisio, Chem. Commun., 2020, 56, 11677–11680 RSC.
- A. A. Wilson, A. Garcia, J. Parkes, S. Houle, J. C. Tong and N. Vasdev, Nucl. Med. Biol., 2011, 38, 247–253 CrossRef CAS PubMed.
- N. Vasdev, O. Sadovski, A. Garcia, F. Dolle, J. H. Meyer, S. Houle and A. A. Wilson, J. Labelled Compd. Radiopharm., 2011, 54, S104 CrossRef.
- J. M. Hooker, A. T. Reibel, S. M. Hill, M. J. Schueller and J. S. Fowler, Angew. Chem., Int. Ed., 2009, 48, 3482–3485 CrossRef CAS PubMed.
- P. M. Rusjan, A. A. Wilson, R. Mizrahi, I. Boileau, S. E. Chavez, N. J. Lobaugh, S. J. Kish, S. Houle and J. C. Tong, J. Cereb. Blood Flow Metab., 2013, 33, 407–414 CrossRef CAS PubMed.
- P. M. Rusjan, A. A. Wilson, L. Miler, I. Fan, R. Mizrahi, S. Houle, N. Vasdev and J. H. Meyer, J. Cereb. Blood Flow Metab., 2014, 34, 883–889 CrossRef CAS PubMed.
- T. Welton, Chem. Rev., 1999, 99, 2071–2083 CrossRef CAS PubMed.
- P. Wasserscheid and W. Keim, Angew. Chem., Int. Ed., 2000, 39, 3772–3789 CrossRef CAS PubMed.
- N. V. Plechkova and K. R. Seddon, Chem. Soc. Rev., 2008, 37, 123–150 RSC.
- C. Phung, R. M. Ulrich, M. Ibrahim, N. T. G. Tighe, D. L. Lieberman and A. R. Pinhas, Green Chem., 2011, 13, 3224–3229 RSC.
- Z. Z. Yang, L. N. He, C. X. Miao and S. Chanfreau, Adv. Synth. Catal., 2010, 352, 2233–2240 CrossRef CAS.
- A. C. Kathalikkattil, J. Tharun, R. Roshan, H. G. Soek and D. W. Park, Appl. Catal., A, 2012, 447, 107–114 CrossRef.
- R. Nasir, D. F. Mohshim, H. A. Mannan, D. Qadir, H. Mukhtar, K. Maqsood, A. Ali, B. Maulianda, A. Abdulrahman and A. Bin Mahfouz, Chem. Pap., 2021, 75, 839–852 CrossRef CAS.
- A. K. Yudin, Aziridines and epoxides in organic synthesis, Wiley, Weinheim, Chichester, 2006 Search PubMed.
- A. Sudo, Y. Morioka, E. Koizumi, F. Sanda and T. Endo, Tetrahedron Lett., 2003, 44, 7889–7891 CrossRef CAS.
- Y. C. Wu and G. S. Liu, Tetrahedron Lett., 2011, 52, 6450–6452 CrossRef CAS.
- X.-Y. Dou, L.-N. He, Z.-Z. Yang and J.-L. Wang, Synlett, 2010, 2159–2163 CAS.
- G. Bresciani, M. Bortoluzzi, G. Pampaloni and F. Marchetti, Org. Biomol. Chem., 2021, 19, 4152–4161 RSC.
- Q. He, J. W. O'Brien, K. A. Kitselman, L. E. Tompkins, G. C. T. Curtis and F. M. Kerton, Catal. Sci. Technol., 2014, 4, 1513–1528 RSC.
- V. Laserna, G. Fiorani, C. J. Whiteoak, E. Martin, E. Escudero-Adan and A. W. Kleij, Angew. Chem., Int. Ed., 2014, 53, 10416–10419 CrossRef CAS PubMed.
- N. Fanjul-Mosteirin, C. Jehanno, F. Ruiperez, H. Sardon and A. P. Dove, ACS Sustainable Chem. Eng., 2019, 7, 10633–10640 CrossRef CAS.
- D. W. Kim, Y. S. Choe and D. Y. Chi, Nucl. Med. Biol., 2003, 30, 345–350 CrossRef CAS PubMed.
- D. W. Kim, C. E. Song and D. Y. Chi, J. Am. Chem. Soc., 2002, 124, 10278–10279 CrossRef CAS PubMed.
- N. Vasdev, E. M. van Oosten, K. A. Stephenson, N. Zadikian, A. K. Yudin, A. J. Lough, S. Houle and A. A. Wilson, Tetrahedron Lett., 2009, 50, 544–547 CrossRef CAS.
- E. M. van Oosten, M. Gerken, P. Hazendonk, R. Shank, S. Houle, A. A. Wilson and N. Vasdev, Tetrahedron Lett., 2011, 52, 4114–4116 CrossRef CAS.
- N. M. Gillings and A. D. Gee, J. Labelled Compd. Radiopharm., 2001, 44, 909–920 CrossRef CAS.
- U. Roehn, J. Becaud, L. J. Mu, A. Srinivasan, T. Stellfeld, A. Fitzner, K. Graham, L. Dinkelborg, A. P. Schubiger and S. M. Ametamey, J. Fluorine Chem., 2009, 130, 902–912 CrossRef CAS.
- S. Lehel, G. Horvath, I. Boros, P. Mikecz, T. Marian, A. J. Szentmiklosi and L. Tron, J. Labelled Compd. Radiopharm., 2000, 43, 807–815 CrossRef CAS.
- M. Sengoden, M. North and A. C. Whitwood, ChemSusChem, 2019, 12, 3296–3303 CrossRef CAS PubMed.
|
This journal is © The Royal Society of Chemistry 2022 |