DOI:
10.1039/D2RA03376C
(Review Article)
RSC Adv., 2022,
12, 20227-20238
Research progress on the effects of nanoparticles on gas hydrate formation
Received
31st May 2022
, Accepted 4th July 2022
First published on 13th July 2022
Abstract
Gas hydrate has great application potential in gas separation, energy storage, seawater desalination, etc. However, the intensity of mass and heat transfer is not enough to meet the needs of efficient hydrate synthesis. Nanoparticles, different from other liquid chemical additives, are considered as effective additives to promote hydrate formation due to their rich specific surface area and excellent thermal conductivity. This work summarizes the effect of the nanoparticles on the thermodynamics and kinetics of hydrate formation. And also, this work probes into the mechanism of the effect of the nanoparticles on the formation of hydrate as well as provides some suggestions for future research. It is found that it's difficult for nanoparticles to effectively promote the formation of the gas hydrate without the use of surfactants, because the adhesion characteristics of the nanoparticles make them easily agglomerate or even agglomerate in solution. In addition, at present, the research on the influence of nanoparticles on the formation and decomposition of natural gas hydrate is still very fragmented, and the micro mechanism of the influence is not clear, which requires more systematic and specific research in the future. At the same time, the development of nanoparticles that can promote the formation of natural gas hydrate should also become the focus of future research.
1. Introduction
Gas hydrate is an ice like crystal compound composed of water molecules and small volume gas molecules such as methane (CH4), carbon dioxide (CO2), nitrogen (N2), hydrogen (H2), ethylene (C2H6), propane (C3H8), etc., and forms under certain temperature and pressure conditions, in which water molecules build cages with different spatial structures through hydrogen bonds and gas molecules stably reside in the cages with van der Waals forces between gas molecules and the hydrate cage. Generally, about 164 cubic meters (standard temperature and pressure (STP)) of gas can be accommodated in a standard volume of hydrates.1,2 As early as the early 19th century, researchers found and proposed the idea of hydrates, but the application research of hydrates to energy or the environment has not been paid attention to. Until the 1990s, researchers found that the formation and decomposition process of hydrate is simple, there was almost no mass loss, environmental protection, and the gas storage capacity was large. The relevant hydrate technologies had certain potential in energy storage, seawater desalination, CO2 gas separation and capture, natural gas hydrate (NGH) exploitation, H2 purification, pipeline safety and other application fields. More importantly, it is found, compared with traditional technologies, hydrate technology has gradually attracted the extensive interest of researchers and become the current research focus because of its natural advantages such as simple process, low energy consumption, high capacity and environmentally friendly.1,3–5
CO2 emission reduction is an important part of mitigating the continuous deterioration of global climate, and hydrate CO2 separation and capture is an important means to achieve CO2 emission reduction. The principle of the hydrate CO2 separation and capture is based on the difference of the phase equilibrium conditions for hydrate formation of different gas components in the mixture. Under certain temperature and pressure conditions, the components with milder phase equilibrium conditions preferentially form gas hydrate and enrich in the hydrate phase, while other components stay in the gas phase. By separating the solid hydrate from the gas phase, the separation of different component gases can be thereby realized.6 The research on hydrate CO2 separation and capture mainly focuses on the thermodynamics, kinetics, micro mechanism, CO2 separation process and separation equipment of gas hydrate formation and decomposition. After nearly two decades of research, although researchers have carried out studies in many aspects and achieved considerable research results, the research on hydrate CO2 separation and capture still remains in the experimental research stage. The slow formation rate of gas hydrate and the low gas consumption in the process of hydrate formation are the two major obstacles restricting the industrial application of hydrate CO2 separation and capture. Essentially, the two obstacles are the problem of heat and mass transfer in the process of hydrate formation. Therefore, enhancing heat and mass transfer in hydrate formation process is the primary research direction, and the effect of hydrate formation promoters on hydrate formation is the main content of the research. Chemical promoters, such as tetrahydrofuran (THF), cyclopentane (CP), tetrabutylammonium bromide ion (TBA+), propane (C3H8), sodium dodecyl sulfate (SDS) and Tween 80, have been intensively studied. Their addition has indeed promoted the formation of gas hydrate, improved the hydrate formation rate and CO2 separation efficiency. However, so far, these hydrate formation promoters have not brought revolutionary optimization to hydrate technology. Moreover, because these promoters are volatile, or chemical toxicity, or difficult to treat, the development of hydrate formation chemical promoters has encountered some bottlenecks. Recently, nanoparticles have been regarded as a new type of solid promoter because of their high specific surface area, quantum size effect and macro quantum tunneling effect, which have the dual effects of thermodynamic and kinetic promoter in the formation of hydrate, and have gradually attracted more and more attention.
Nanoparticles are fine particles with a size of 1–100 nm. Compared with micron particles, nanoparticles have higher specific surface area and better heat transfer enhancement characteristics.7,8 In 2006, Li et al.9 took the lead in adding copper (Cu) nanoparticles into the hydrate formation system and proved that Cu nanoparticles promoted the formation of gas hydrate. Due to the high thermal conductivity of nanoparticles, the thermal conductivity of the solution was thereby significantly improved after adding nanoparticles into the solution. Ma et al.10 used this property to synthesize CH4 hydrate in supersaturated magnesium sulfate (MgSO4) or copper sulfate (CuSO4) solution. It was found that the presence of MgSO4 or CuSO4 nanoparticles significantly promoted the formation of CH4 hydrate relative to ionic solution. Li Dongliang et al.11 further proved the heat transfer enhancement effect of carbon nanotube and Cu nanoparticles in the formation of THF hydrate through experimental research, and proposed that the enhancement effect would be more significant in the presence of dispersant. The greater the concentration of nanoparticles and the smaller the size of nanoparticles, the more outstanding the enhancement effect. However, due to its high surface energy, the nanoparticles are easy to coalesce in the solution and difficult to form a stable, uniform and highly dispersed suspension solution, which reduces the thermal conductivity of the overall solution and is detrimental to the formation of hydrate. Therefore, the research of nanoparticles as hydrate formation promoters has not attracted much attention of researchers.
In this work, we have fully investigated the influence of the nanoparticles on the thermodynamics, kinetics and formation mechanism of gas hydrate formation, as shown in Table 1. The effects of the nanoparticles on gas hydrate formation in different systems are systematically summarized, and the various studies are analyzed and evaluated. On this basis, some suggestions are put forward to further develop the nanoparticles and improve the effects of the nanoparticles on gas hydrate formation.
Table 1 List of recent studies on the effects of nanoparticles or nanofluids on gas hydrate formation
Ref. |
NM |
Gas |
Size (nm) |
Concentration |
Modification |
T (K) |
P (MPa) |
Dispersant |
Remark |
84 |
Fe3O4 |
CH4 |
20 |
0.2–1.6 g L−1 |
SDS-coated |
275.15 |
6 |
|
Compared with only SDS, the induction time was shorter and the gas consumption was higher |
34 |
Fe3O4 |
CO2 |
50 |
0.01–0.15 wt% |
Magnetic field |
274.15–276.15 |
3.01–4.06 |
CTAB |
Under the optimum conditions, the gas consumption increased by 443.9% and the induction time decreased by 96.6% |
85 |
Fe3O4 |
CH4 |
|
0.1 wt% |
SDS & SO-coated, magnetic field |
276.15 |
6 |
|
Shortening induction time and increasing gas storage capacity |
35 |
Fe3O4 |
CO2 |
50 |
0.05–0.25 wt% |
|
274.15, 276.15 |
2.5 |
SDS or CTAB |
0.15 wt% Fe3O4 nanoparticle and 400 ppm SDS in the based fluid is the best aqueous solution for CO2 hydrate formation, with a 70.6% decrement in induction time and a 160% increment in gas consumption |
35 |
CuO |
CH4 |
|
0.01–1 wt% |
|
275.65 |
5.5 |
SDS |
The induction time decreased by 92.7% and the gas storage capacity increased by 34% |
35 |
CuO |
CH4 |
40 |
0.05–1 wt% |
|
274.65, 276.65 |
5, 6 |
SDS |
There is no significant effect on the final gas storage capacity |
86 |
Cu |
CH4 |
25, 75 |
0.0157 M, 0.157 M |
|
275.15–275.76 |
5.24–5.55 |
CTAB |
Cu nanoparticles and CTAB can shorten the induction time, increase the reaction rate and increase gas consumption |
9 |
Cu |
HFC134a |
20 |
0.1 wt%, 1 wt% |
|
274.15 |
5.7 |
SDBS |
The rate of gas hydrate formation is increased and the dissociation pressure is changed |
87 |
ZnO |
CH4 |
10–30 |
0.05 wt%, 0.1 wt% |
|
274.65 |
4.9–6 |
SDS |
Positive effect on induction time, reaction rate, solubility of methane and gas consumption. But no significant effect on gas capacity |
88 |
ZnO |
CO2 |
11.5 |
0.1 wt% |
|
274 |
2.2, 2.6, 3.2 |
|
Increase the gas consumption by up to 16% |
89 |
Ag |
CH4 |
50–75 |
|
|
275.15 |
4.7, 5.7 |
|
The induction time was shortened by 85% and 73.9%, and the gas consumption was increased by 33.7% and 7.4%, respectively |
90 |
Ag |
CH4 |
6–30 |
4.5, 9, 18, 27, 36 μM |
Triangular silver nanoparticles |
275.15, 276.15 |
4.5,4.8,5,5.3,5.5 |
|
Induction time reduced by 97% |
91 |
Ag |
C2H6 |
6–30 |
4.5, 9, 18, 27, 36 μM |
|
276.15, 277.65 |
1.5,1.9,2.2 |
|
Induction time reduced by 97.5% |
36 |
Ag |
CO2 |
|
45, 90 μM |
|
273.65, 275.65 |
2, 3 |
SDS |
Ag nanoparticles + SDS can increase gas consumption and apparent rate constant |
92 |
Al2O3 |
CO2 |
|
0.1–0.6 wt% |
|
273.15 |
0.1 |
THF, SDS |
Under the optimum ratio of THF, SDS and Al2O3, the formation rate can be increased by 3.74 times |
93 |
Al2O3 |
CO2 |
30 |
0.005–0.5 wt% |
Non-modified, hydrophilic and hydrophobic |
275.15 |
4.5 |
|
Three kinds of particles have little effect on the phase equilibrium. The non-modified particles can shorten the induction time, but reduce the gas consumption. 0.01 wt% hydrophilic modification has better promoting effect, shortening induction time and increasing gas consumption |
21, 22 |
C |
CH4 |
10–15 |
0.003 wt%, 0.004 wt% |
Carbon nanotubes |
274.15 |
|
|
Carbon nanotubes can increase gas consumption by 300% and reaction rate, and oxidized carbon nanotubes are more effective at increasing rate. Both have a slightly positive effect on thermodynamic equilibrium |
37 |
C |
CO2 |
50 |
0.4 wt% |
Graphite nanoparticles |
277.15 |
3.5 |
|
Induction time was shortened by 80.8%, and the maximum air consumption was increased by 12.8% |
23 |
C |
CO2 |
50 |
0.4 wt%, 0.8 wt%, 1.2 wt% |
Graphite nanoparticles |
275.7–280.9 |
1.6–3.3 |
|
Graphite nanoparticles have an adverse effect on the phase equilibrium of CO2 hydrate formation, but it is independent of particle concentration |
94 |
C |
CH4 |
31.4 |
0.1–10 ppm |
Hydrophilic, hydrophobic carbon nanotubes |
275.15 |
4.6 |
|
The formation rate of hydrophobic carbon nanotubes was increased by 6% at high concentration and by 16.34% at low concentration |
38 |
GO |
CO2 |
|
20–300 ppm |
|
279 |
3–5 |
|
The induction time was shortened by 53–74.3%, the gas consumption increased by 5.1–15.9%, and the optimal concentration was 50 ppm |
95 |
SiO2 |
CH4 |
20–30 |
0.1–0.4 wt% |
Porous medium |
274.15 |
6 |
|
The concentration of 0.1–0.3 wt% has positive effect on the rate of hydrate formation, while the concentration of 0.4 wt% significantly inhibits hydrate formation |
96 |
Al2O3, SiO2, Cu, Ag |
75% CH4 + 25% CO2 |
<50, 10–20, 40–60, <150 |
0.1–0.3 wt% |
|
274.15 |
4 |
|
SiO2 can increase the gas consumption by the most, up to 45%, Cu and Al2O3 by 1–15%, and Ag has no significant effect |
97 |
Al2O3 + GO |
CH4 |
Al2O3: 10–20 |
0.2–0.6 wt% + 100–400 ppm |
|
281.15 |
3 |
THF |
The induction time was shortened and the reaction rate was increased, but the final gas consumption and gas reserves were not affected |
2. Effects on thermodynamics
The formation of gas hydrate is an exothermic process, which will lead to the increase of system temperature, then reduce the driving force of the system and affect the further formation of hydrate. Therefore, improving the heat transfer efficiency of the system and transferring the generated heat in time is conducive to the better formation of hydrate. Nanofluids generally have better heat transfer characteristics. For examples, the thermal conductivity of ethylene glycol solution containing 4 vol% copper oxide (CuO) nanoparticles can be increased by more than 20.0%,12 the thermal conductivity of carbon nanotube oil system with nanoparticle concentration of 1 vol% is increased by 160.0%,13 and the thermal conductivity of aqueous solution containing 2 vol% Cu nanoparticle is increased by 60.0%.14 The improvement of thermal conductivity of nanoparticles is related to the type, size, concentration of nanoparticles and system temperature. The thermal conductivity of common nanofluids such as Al2O3, C, Cu and CuO not only increases with the increase of concentration within a certain concentration range, but also increases with the increase of temperature.11,15–18 However, not all nanoparticles follow this law. For example, within a certain concentration range, the thermal conductivity of TiO2 nanofluids increases with the increase of concentration, but decreases with the increase of temperature.19 In addition, Li et al.11 and Mintsa et al.16 pointed that the small size nanoparticles are more conducive to enhance the heat transfer effect. Actually, the heat transfer effect of the nanofluids is affected by many factors, such as pH of the solution, the particle aggregation effect, the stability of suspension and the use of surfactant, which make it difficult to predict the thermal conductivity of the nanofluids in hydrate system. Consequently, it should be pointed out that, up to date, the mechanism of heat transfer enhancement by the nanoparticles is not fully studied. Although scholars have discussed many possible controlling factors, including Brownian motion, solid–liquid interface layer, ballistic phonon transport and surface charge state,20 the mechanism of heat transfer enhancement by nanoparticles is still unclear. This leads to the difficulty of targeted selection of nanoparticles for the purpose of enhancing heat transfer. The usage of nanoparticles is more like asking for directions than scientific guidance.
In terms of thermodynamics, nanoparticles have slight performance in promoting or inhibiting the thermodynamic phase equilibrium conditions of natural gas hydrate. When studying the effect of carbon nanotubes on the formation of methane hydrate, Park et al.21,22 found that the addition of carbon nanotubes could slightly shift the phase equilibrium curve of methane hydrate to the right, as shown in Fig. 1, which meant that the carbon nanotubes improved the formation conditions of methane hydrate. Yu et al.23 and Zhou et al.24 pointed out that graphite nanoparticles had a negative impact on the phase equilibrium of CO2 hydrate system, which was independent of the concentration of nanoparticles. They drew the phase equilibrium curves as shown in Fig. 2, and summarized two reasons why graphite nanoparticles affect the phase equilibrium. First, the existence of nanoparticles increased the disorder of water molecules, thus increasing the entropy of the system, so the formation of hydrate crystals required more hydrogen bond energy. Second, some graphite nanoparticles had porous structure, which would reduce the activity of water and increase the pressure required for hydrate formation. However, this did not seem to explain the positive effect of carbon nanotubes with similar structure to graphite nanoparticles on phase equilibrium. In fact, carbon nanotubes and graphite nanoparticles are different in their interactions with water molecules at the micro level, which leads to their different effects on phase equilibrium. Due to the H–π interaction between water molecules and π electron clouds of carbon nanotubes, water molecular clusters tend to be closer to carbon nanotubes, so water molecules are arranged more closely and orderly in a local range. At the same time, under the action of carbon nanotubes, the O–O spacing of water molecules around them is shortened and the hydrogen bond is enhanced.25 The graphite nanoparticles will not affect the O–O spacing of the surrounding water molecular clusters, but will attract some hydrogen bonds to the graphite surface, reducing the hydrogen bonds between water molecules,26 leading to a slight thermodynamic inhibition. Similarly, zinc oxide (ZnO) nanoparticles have a slight thermodynamic inhibition.27 This can be explained by the fact that zinc hydroxide (Zn(OH)2) generated by ZnO in water will attract some hydrogen bonds.28 Generally speaking, nanoparticles have only a slight effect on the phase equilibrium of gas hydrate, and most of the discussion on the influence mechanism focuses on the interaction between nanoparticles and water molecules.
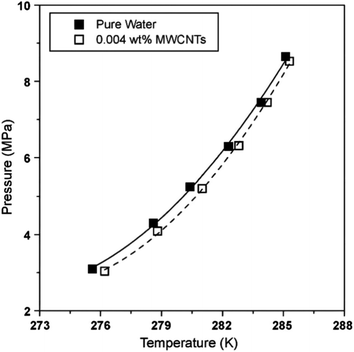 |
| Fig. 1 Phase equilibrium of methane hydrate with or without MWCNTs (■: distilled water, □: 0.004 wt% MWCNTs).21 | |
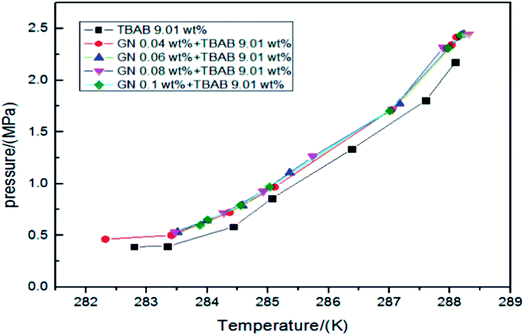 |
| Fig. 2 Phase equilibrium of tetrabutyl ammonium bromide (TBAB) + graphite nanoparticle (GN) varying with different GN concentrations.24 | |
The characteristics of nanoparticles to enhance heat and mass transfer have more application scenarios to be developed in the field of hydrate technology, such as separating binary or multicomponent gases with nanoparticles, and separating CO2/H2 mixture by hydrate method with aluminum oxide (Al2O3) nanoparticles and TBAB. The experimental data are shown in Fig. 3. It can be found that the addition of 0.50 wt% Al2O3 nanoparticles can significantly improve the separation effect.29 At present, there are no more experiments using nanoparticle as hydrate formation promoter for gas separation. Looking for suitable nanoparticles to improve the separation efficiency and promote the carbon capture by hydrate method has become a next research topic. In addition, the nanoparticles also have development potential in the field of hydrate hydrogen storage technology. Carbon nanotubes have been proved to have certain hydrogen storage capacity,30,31 but the hydrogen storage capacity is not enough to support it as a separate hydrogen storage material.32 However, due to the enhanced mass and heat transfer characteristics of carbon nanotubes in the hydrate formation process, the composite hydrogen storage of carbon nanotubes and hydrate has become a potential hydrogen storage method. Dr Yu Chi33 used the aqueous solution of multi walled carbon nanotubes (MWCNTs) to conduct the hydrate hydrogen storage experiment, and found that although the gas storage capacity was slightly improved, the gas storage rate was significantly improved. He made MWCNTs solution into ice powder after freezing to replace liquid water for hydrate formation. The results showed that the gas storage capacity of 1.00 wt% MWCNTs ice powder was 6.4 times higher than that of ordinary ice powder, and when MWCNTs aqueous solution generates hydrate, the hydrate layer formed near carbon nanotubes hinders the improvement of gas storage capacity, which provides a new idea for hydrate hydrogen storage.
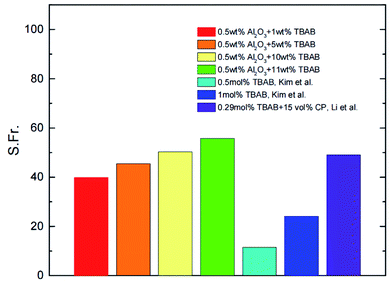 |
| Fig. 3 Comparison of the split fraction for the CO2/H2 hydrate formation with the synergic additives and other additives.29 | |
3. Effects on kinetics of CO2 hydrate formation
In terms of promoting the formation of CO2 hydrate, Firoozabadi et al.34 and Rajabi et al.35 studied the effect of ferroferric oxide (Fe3O4) nanoparticles on the formation process of CO2 hydrate. They found that Fe3O4 nanoparticles can effectively increase gas consumption and shorten induction time in the presence of surfactant. Compared with Fe3O4 nanoparticles cooperating with cetyltrimethyl ammonium bromide (CTAB), the combination of Fe3O4 nanoparticles and SDS performs better. The nanofluid with concentration of 0.15 wt% + 400 ppm can shorten the induction time of CO2 hydrate formation by 70.6%, and increase the gas consumption and apparent rate constant by 160.0% and 120.5% respectively. In addition, in addition, they also found that the presence of magnetic field significantly promoted the formation of CO2 hydrate due to the magnetic properties of Fe3O4 nanoparticles, for instance, the induction time was reduced by 96.6% and the gas consumption increased by 443.9% with the system in the presence of 0.15 wt% Fe3O4 nanoparticles. Mohammadi et al.27 reported the promoting effect of ZnO nanoparticles on the formation of CO2 hydrate. The results showed that ZnO nanoparticles could increase gas consumption by up to 16.0% in the presence of SDS. In addition, they studied the effects of Ag nanoparticles, SDS solution and silver (Ag) + SDS mixed system on the formation of CO2 hydrate. The results showed that the induction time did not change significantly in the three cases.36 Only Ag + SDS mixed system has an effect on the formation process of CO2 hydrate, which can increase the gas consumption by 93.3% and the apparent rate constant by 133.0%. Zhou et al.37 reported that the induction time can be reduced by 80.8% and the gas consumption increased by 12.8% with the system in the presence of 0.40 wt% of graphite nanoparticles. Yan et al.38 found that graphite oxide nanoparticles can shorten the induction time by 53.0%–74.3% and increase the gas consumption by 5.1%–15.9%.
It can be seen that different kinds of nanoparticles have different promoting effects on the formation of CO2 hydrate. In terms of the effect, the Fe3O4 nanoparticles have the best promotion effect, and their promotion effect on CO2 hydrate under the action of magnetic field has great research and development potential. The combination of Ag nanoparticles + SDS can greatly improve the gas consumption, but can not shorten the induction time, which is difficult to meet the industrial application. The increase of gas consumption by graphite and graphene nanoparticles is not significant, which may be related to the fact that they are not used with surfactants. It is worth noting that the nanoparticle, as a new kind of hydrate formation promoter, is expected to become a more efficient and green substitute for traditional hydrate formation promoters. However, the nanoparticle can hardly play an efficient role in promoting the formation of CO2 hydrate without being used in combination with surfactants so far. Therefore, to find efficient and green nanoparticle promoter must be one of the important topics in the future for promoting the conversion of the hydrate-based CO2 capture technology to the industrial application.
4. Effect in drilling fluid and cement slurry
Invasion of drilling fluid into natural gas hydrate reservoir during drilling might cause hydrate dissociation and reformation and then lead to wellbore instability, which is one of the most challenging issues in the drilling industry. Recently, nano-based drilling fluids (NBDF) are widely studied. Adding nanoparticles into drilling fluid can help improve the rheology of drilling fluid, fluid loss stability and wellbore stability, and reduce the invasion of hydrate reservoir. Bég et al.39 found that titanium dioxide (TiO2) nanoparticles could increase the plastic viscosity (PV), yield point (YP), apparent viscosity (AV) of drilling fluids. And 0.60 wt% (w/w) is the optimal concentration of TiO2 nanoparticles, which has the best effect on the rheological properties and lubricity of the drilling fluid. Medhi's team conducted a series of studies on drilling fluids containing various types of nanoparticles, including Al2O3, ZnO, SiO2, ZrO2, CuO, and graphene. All these nanoparticles showed better rheology and filtration properties. When the concentration of graphene particles was 0.80 wt%, the filtration rate decreased by 60.0%.40–44 Researchers are still looking for the most effective nanoparticles or combinations. As a drilling fluid additive, the new nano-lignin amphoteric copolymer synthesized by Chang can reduce the fluid loss by 80%.45 Similarly, the improvement of rheological properties has been reported in some studies on polymers made of nanoparticles.46,47 At the same time, several articles have reported the improvement of the thermal stability of drilling fluids by nanoparticles.40,48,49
Wellbore stability is another important topic in drilling. Many researchers have studied the role of nanomaterials in maintaining wellbore stability. Xu et al.50 designed a nano-emulsion, which was shown to be a good shale self-imbibition control agent, and the swelling rate decreased from 12.9% to 4.7%. Aramendiz and Imqam51 added silica and graphene nanoparticles into drilling fluid and found that it provided sufficient blocking networks between grain boundaries, resulting in a 35.6% reduction in cutting erosion. In another study, Liu et al.52 found that the LAPONITE® nanoparticle can be inserted into fissures to effectively reduce shale swelling.
Although so many NBDFs have been studied, the addition of nanoparticles in drilling fluid is still controversial. As we mentioned above, one of the important problems is that the contribution of the nanoparticles to hydrate formation may increase the risk of flow blockage (or clogging). Therefore, it is necessary to look for the nanoparticles which can inhibit hydrate formation as drilling fluid additives. Wang et al.53 found that the hydrophilic silicon dioxide (SiO2) nanoparticles at 4.00 wt% had a significant inhibition effect on hydrate formation, the induction time increased by 194.0% and the hydrate formation decreased by 10.0%. They pointed out that the inhibition was related to the hydrophilicity. Similarly, Guo et al.54 concluded that although CaCO3 nanoparticles promoted the formation of hydrate, the hydrophilic CaCO3 nanoparticles after modification inhibited the formation of hydrate. Xu et al.55 conducted wellbore simulation experiments and showed that the inhibition effect of the hydrophilic SiO2 on hydrate in the wellbore increased with the increase in particle size.
Cementing technology is important in the aspect of stabilizing the well wall. The heat released from the hydration process of cement slurry will lead to the increase of hydrate reservoir temperature and the decomposition of hydrate, which threatens the drilling safety.56,57 Merey58 summarized several requirements for a new type of cement slurry for drilling hydrate reservoirs: (1) lower heat of hydration than conventional cements. (2) Lower thermal conductivity than conventional cements. (3) Superior anti-gas migration performance than conventional cements. (4) Low density for oceanic hydrate deposits as well as high compressive strength. Recently, many researchers have studied the enhancement of cement slurry by nanoparticles.59–61 Among them, nano-silica are the most studied particles. Nano-silica particles affect cement slurry in the thickening time, compressive strength, fluid loss, porosity and permeability within the cement.62 Maagi et al.63 pointed out that the effect of nanoparticles on cement pastes depends on particle concentration and temperature. Compared with drilling fluid, the types of nanoparticles used in cement slurry are not extensive. The study of the effect of cement slurry on hydrate reservoir is still the emphasis.
5. Affecting mechanism of nanoparticles on hydrate formation
The main factors affecting the stability of nanoparticle suspension include nanoparticle size, concentration, dispersant, solution viscosity and solution pH.64,65 If the attraction between particles is greater than the repulsion force, the nanoparticles may collide and coalesce, so as to reduce the stability of the suspension. Conversely, if the particles have high enough repulsion force, the suspension always remain stable. Therefore, for stable nanofluids or colloids, the repulsion force between particles must be dominant. Adding surfactants is an effective method to stabilize nanofluids or colloids. Water soluble surfactants have a significant positive impact on the stability of nanofluids that promote hydrate formation.66–68 Cox et al.69 studied the effect of nanoparticles on the formation characteristics of CH4 hydrate through the combination of MDS and experiments, and proved that nanoparticles can promote the formation of CH4 hydrate in a way similar to the nucleation of heterogeneous ice for more hydrophilic or water-soluble guest molecules (such as THF or CO2). Bai et al.70 studied the particle effect during the formation and decomposition of CO2 hydrate, and found that the good flow characteristics in the initial stage of hydrate nucleus in the reactor are conducive to the continuous formation of hydrate; and once the hydrate fine particles coalesce, the fluid fluidity in the reactor will be reduced, which is not conducive to the continuous formation of hydrate. Nahri et al.71 studied the effects of amorphous carbon, iron oxide ferric oxide (Fe2O3), SiO2 and amino-coated SiO2 on the nucleation and aggregation rate of THF hydrate. It was found that due to the aggregation of nanoparticles in solution, the growth of hydrate was inhibited, and the ammonia coated SiO2 showed the prominent the inhibitory effect. Cendales et al.72 from the team led by Peter Englezos of the University of British Columbia in Canada pointed out through experiments that the cellulose nanocrystals did not promote the formation of CO2 hydrate. These studies give the results of the influence of nanoparticles on hydrate formation from both positive and negative aspects, and provide directional guidance for the follow-up research of nanoparticles on hydrate formation, that is, what kind of nanoparticles and what kind of nano solution system are conducive to hydrate formation.
Alireza Bozorgian73 used CTAB as surfactant to study the effect of ZnO nanoparticles on CO2 storage by hydrate method. The results showed that when the concentration of ZnO nanoparticles in the solution is 0.1%, the CO2 consumption during hydrate formation increases by about 26.0%, and the CO2 storage capacity of hydrate increases by about 22.0%. Song et al.74 from Qingdao Institute of energy and process, Chinese Academy of Sciences, carried out a study on the effect of carbon nanotubes doped with SDS on the formation of CH4 hydrate. It was found that, on one hand, the defect sites generated during acid treatment improved the stability and dispersion of nanotubes in the aqueous phase, on the other hand, due to the continuous Brownian motion of nanoparticles, adding nanotube particles to SDS solution with a concentration lower than 0.4 mmol L−1 significantly shortened the induction time of CH4 hydrate formation. In addition, they also found that since the nanoparticles are easy to agglomerate in the liquid phase, the concentration of nanoparticles must be kept at a low level, otherwise it would be detrimental to the formation of hydrate. Then, Wang et al.75 continued to study the effect of grafting Ag nanoparticles onto SDS coated nano microspheres on the formation of CH4 hydrate. It was found that the formation time of CH4 hydrate was only one tenth of that of SDS system without Ag nanoparticles, which once again proved the promoting effect of nanoparticles on the formation of hydrate. Zhang et al.76 studied the formation characteristics of CO2 hydrate when graphene oxide and nano graphite particles are combined with SDS, and found that graphene oxide and nano graphite particles can significantly promote the formation of CO2 hydrate when combined with SDS. Under the same working conditions, the addition amount of the graphene oxide is less, but a more optimized promotion effect can be obtained.
However, some recent studies have shown that the increase of nanoparticle concentration is helpful to improve the gas consumption during hydrate formation. Chaturvedi et al.77 from India used Stober sol gel to synthesize SiO2 nanoparticles in one step, and studied the effect of SiO2 nanoparticles on the separation and capture of CO2 in the flue gas by hydrate method. It was found that SiO2 nanoparticles effectively increased the total gas consumption of about 37%, and considered that the consumption of gas was positively correlated with the concentration of nanoparticles. Lu et al.78 prepared graphite nanofluids and studied the effect of graphite nanofluids on the formation of CH4 hydrate. It was found that graphite nanofluids can obtain higher gas consumption than ZnO, CuO and Fe3O4 nanofluids. In addition, graphite nanofluids significantly improved the hydrate formation rate. At the same time, they also used the self-made graphene oxide nanofluid hydrate to separate the methane of low concentration coalbed methane (CBM). After two-stage separation, the CH4 concentration increased from 30 mol% to 76 mol% while effectively improving the formation rate of CH4 hydrate.79 In addition, Liu et al.80 also studied the effect of the graphene oxide on CO2 hydrate formation and concluded that the graphene oxide significantly promoted CO2 hydrate. Jiao et al.81 from China University of petroleum (East China) did the opposite, studied the influence of SiO2 nanoparticles on the decomposition characteristics of CO2 hydrate, and found that SiO2 nanoparticles promoted the decomposition of CO2 hydrate. The variation law of thermal conductivity in the decomposition process has been fluctuating with the law of gas production from hydrate decomposition, and the thermal conductivity of the system has increased by 2.4–5.5% compared with pure CO2 hydrate. The decrease of activation energy is the fundamental reason why nanoparticles promote the decomposition of CO2 hydrate. Therefore, it was considered that the activation energy of the system is negatively correlated with the thermal conductivity of the system, that is, the greater the thermal conductivity, the shorter the hydrate decomposition relaxation time and the faster the mass transfer. This study not only verified the promoting effect of nanoparticles on the formation and decomposition of CO2 hydrate, but also tried to reveal the influence mechanism of nanoparticles on the formation and decomposition of CO2 hydrate from the physicochemical level. Min et al.82 from J. W. Lee team of Korea Institute of advanced science and technology used molecular dynamics simulation (MDS) studied the effect of hydrophobic nanoparticles on the formation of CH4–THF binary hydrate. It was pointed out that the hydrate cell formed on the inner wall of hydrate provided heterogeneous station sites conducive to hydrate formation. The disordered arrangement of water molecules at the gas–liquid interface reduced the free energy of the adjacent region, thus reducing the driving force of hydrate formation, while hydrophobic nanoparticles were located at the water oil interface to separate the water phase from gas molecules, thus preventing the continuous formation of hydrate. Hu et al.83 from Shenzhen Research Institute of Tsinghua University studied the molecular mechanism of CH4 hydrate nucleation on the surface of corroded iron and found that only when iron was above the gas–liquid interface, iron particles with different specific surface areas could significantly promote the formation of CH4 hydrate, and the promotion effect of iron particles after corrosion was more obvious. This was because the existence of iron particles increased the curvature of the gas–liquid interface, which reduced the energy barrier when CH4 molecules transfer from the interface to the volume liquid by 54.0%, resulting in the increase of CH4 concentration in the solution, which was the common influence of the gas–liquid–solid (iron particles) three-phase interface on the formation of CH4 hydrate.
It is certain that the above research has important enlightenment significance for revealing the influence mechanism of nanoparticles on the formation and decomposition of gas hydrate, but it is undeniable that the current research on the influence of nanoparticles on the formation and decomposition of gas hydrate is still very fragmented, and the micro mechanism of influence is still unclear. In particular, the synthesis of nanoparticles or the development of nanofluids that can significantly promote hydrate formation and the micro mechanism of their targeted promotion on hydrate formation are still blank, which restricts the industrial development of hydrate technology to a great extent. Therefore, it is necessary to carry out the research on the synthesis of nanoparticles that can promote the formation of CO2 hydrate in the future. On one hand, the synthesis method of nanoparticles is proposed through the combination of experimental research, microanalysis and molecular dynamics simulation. On the other hand, the micro mechanism of promoting the formation of gas hydrate by nanoparticles from the perspective of mass and heat transfer at the three-phase interface of gas liquid solid (nanoparticles) is revealed based on the effect of nanoparticles on the thermal conductivity of hydrate reaction system.
6. Conclusions
Nanoparticles have attracted researchers' attention because of their higher thermal conductivity and specific surface area, which can effectively promote the heat and mass transfer in the formation of gas hydrate. By investigating the relevant studies that have been carried out currently, it is found that the research focuses on the effects of the nanoparticles on the thermodynamics, kinetics and interface reaction of the gas hydrate formation. The promoting effect of nanoparticles on the formation of gas hydrate is mainly reflected in that they can not only effectively improve the thermal conductivity of the solution system and provide a larger specific surface area to promote mass transfer, but also the structural defects of nanoparticles can provide hydrate crystal nucleation sites and shorten the induction time of hydrate crystal nucleation. However, it is also found the nanoparticle can hardly play an efficient role in promoting the formation of gas hydrate without being used in combination with surfactants due to that nanoparticles are easy to agglomerate or even agglomerate in the solution for their adhesion characteristics. In addition, the current research on the influence of nanoparticles on the formation and decomposition of gas hydrate is still very fragmented, and the micro mechanism of influence is still unclear, and this calls for more systematic and specific research in the future. Meanwhile, we also found that the modification of nanoparticles (such as adding some hydrophobic groups) might bring about the improvement on the dynamic influence of nanoparticles on the formation of gas hydrate. Therefore, if the nanoparticles can be modified in advance to achieve a targeted role in promoting the formation of gas hydrate according to the characteristics of synthetic gas hydrate, it will be of great significance to realize the commercial application of gas hydrate technology in the future.
Abbreviation
NM | Nanoparticle material |
T | Temperature |
P | Pressure |
CTAB | Cetyltrimethyl ammonium bromide |
SDS | Sodium dodecyl sulfate |
SO | Sodium oleate |
SDBS | Sodium dodecyl benzene sulfonate |
THF | Tetrahydrofuran |
GO | Graphite oxide |
Conflicts of interest
There are no conflicts to decare.
Acknowledgements
The authors greatly appreciate financial support from the Key Program of National Natural Science Foundation of China (51736009), the Natural Science Foundation of Guangdong Province, China (2019A1515011490), Guangdong Special Support Program-Local Innovation and Entrepreneurship Team Project (2019BT02L278), Special Project for Marine Economy Development of Guangdong Province (GDME-2018D002), Fundamental Research & Applied Fundamental Research Major Project of Guangdong Province (2019B030302004, 2020B0301 03003), Science and Technology Apparatus Development Program of the Chinese Academy of Sciences (YZ201619), Frontier Sciences Key Research Program of the Chinese Academy of Sciences (QYZDJSSW-JSC033).
References
- X. S. Li, C. G. Xu, Y. Zhang, X. K. Ruan, G. Li and Y. Wang, Investigation into gas production from natural gas hydrate: A review, Appl. Energy, 2016, 172, 286–322 CrossRef CAS.
- D. Sloan and C. A. Koh, Clathrate Hydrates of Natural Gases, 3rd edn, 2007 Search PubMed.
- C. X. Cheng, F. Wang, Y. J. Tian, X. H. Wu, J. L. Zheng, J. Zhang, L. W. Li, P. L. Yang and J. F. Zhao, Review and prospects of hydrate cold storage technology, Renew. Sustain. Energy Rev., 2020, 117, 109492 CrossRef CAS.
- J. N. Zheng, F. B. Cheng, Y. P. Li, X. Lv and M. J. Yang, Progress and trends in hydrate based desalination (HBD) technology: A review, Chinese J. Chem. Eng., 2019, 27, 2037–2043 CrossRef CAS.
- Y. Zhang, L. Zhao, S. Deng, R. K. Zhao, X. H. Nie and Y. N. Liu, Effect of Nanobubble Evolution on Hydrate Process: A Review, J. Therm. Sci., 2019, 28, 948–961 CrossRef CAS.
- S. S. Fan, S. L. You, X. M. Lang, Y. H. Wang, W. T. Li, Y. Z. Liu and Z. Zhou, Separation and capture carbon dioxide by clathrate-hydrate membranes: a review, Chem. Ind. Eng. Prog., 2020, 39, 1211–1218 Search PubMed.
- S. M. Hamidinejad, R. K. M. Chu, B. Zhao, C. B. Park and T. Filleter, Enhanced Thermal Conductivity of Graphene Nanoplatelet-Polymer Nanocomposites Fabricated via Supercritical Fluid-Assisted in Situ Exfoliation, ACS Appl. Mater. Interfaces, 2018, 10, 1225–1236 CrossRef CAS PubMed.
- M. Barahoei, A. Z. Hezave, S. Sabbaghi and S. Ayatollahi, Copper Oxide Nano-Fluid Stabilized by Ionic Liquid for Enhancing Thermal Conductivity of Reservoir Formation: Applicable for Thermal Enhanced Oil Recovery Processes, Chem. Ind. Chem. Eng. Q., 2016, 22, 211–225 CrossRef CAS.
- J. P. Li, D. Q. Liang, K. H. Guo, R. Z. Wang and S. S. Fan, Formation and dissociation of HFC134a gas hydrate in nano-copper suspension, Energy Convers. Manag., 2006, 47, 201–210 CrossRef CAS.
- S. H. Ma, Z. Pan, P. Li, Y. G. Wu, B. F. Li, J. K. Kang and Z. E. Zhang, Experimental Study on Preparation of Natural Gas Hydrate by Crystallization, Sh. Build. China, 2017, 58, 226–232 Search PubMed.
- D. L. Li, H. Peng and D. Q. Liang, Thermal conductivity enhancement of clathrate hydrate with nanoparticles, Int. J. Heat Mass Transf., 2017, 104, 566–573 CrossRef CAS.
- S. Lee, S. Choi, S. Li and J. A. Eastman, Measuring Thermal Conductivity of Fluids Containing Oxide Nanoparticles, Jounal Heat Transf, 1999, 121, 280–289 CrossRef CAS.
- S. U. S. Choi, Z. G. Zhang, W. Yu, F. E. Lockwood and E. A. Grulke, Anomalous thermal conductivity enhancement in nanotube suspensions, Appl. Phys. Lett., 2001, 79, 2252–2254 CrossRef CAS.
- Q. Li and Y. M. Xuan, Convective heat transfer and flow characteristics of Cu-water nanofluid, Sci. China, 2002, 45, 408–416 CAS.
- N. Kumar, S. S. Sonawane and S. H. Sonawane, Experimental study of thermal conductivity, heat transfer and friction factor of Al2O3 based nanofluid, Int. Commun. Heat Mass Transf., 2018, 90, 1–10 CrossRef CAS.
- H. A. Mintsa, G. Roy, C. T. Nguyen and D. Doucet, New temperature dependent thermal conductivity data for water-based nanofluids, Int. J. Therm. Sci., 2009, 48, 363–371 CrossRef CAS.
- M. B. Xing, J. L. Yu and R. X. Wang, Experimental study on the thermal conductivity enhancement of water based nanofluids using different types of carbon nanotubes, Int. J. Heat Mass Transf., 2015, 88, 609–616 CrossRef CAS.
- M. T. Naik and G. Ranga Janardhana, Temperature dependent thermal conductivity enhancement of copper oxide nanoparticles dispersed in propylene
glycol-water base fluid, Int. J. Nanoparticles, 2010, 3, 149–159 CrossRef CAS.
- W. Duangthongsuk and S. Wongwises, Measurement of temperature-dependent thermal conductivity and viscosity of TiO2-water nanofluids, Exp. Therm. Fluid Sci., 2009, 33, 706–714 CrossRef CAS.
- X. Q. Wang and A. S. Mujumdar, Heat transfer characteristics of nanofluids: a review, Int. J. Therm. Sci., 2007, 46, 1–19 CrossRef.
- S. S. Park, S. B. Lee and N. J. Kim, Effect of multi-walled carbon nanotubes on methane hydrate formation, J. Ind. Eng. Chem., 2010, 16, 551–555 CrossRef CAS.
- S. S. Park, E. J. An, S. B. Lee, W. gee Chun and N. J. Kim, Characteristics of methane hydrate formation in carbon nanofluids, J. Ind. Eng. Chem., 2012, 18, 443–448 CrossRef CAS.
- Y. S. Yu, S. D. Zhou, X. Sen Li and S. L. Wang, Effect of graphite nanoparticles on CO2 hydrate phase equilibrium, Fluid Phase Equilib, 2016, 414, 23–28 CrossRef CAS.
- S. D. Zhou, K. Jiang, Y. L. Zhao, Y. D. Chi, S. L. Wang and G. Z. Zhang, Experimental Investigation of CO2 Hydrate Formation in the Water Containing Graphite Nanoparticles and Tetra-n-butyl Ammonium Bromide, J. Chem. Eng. Data, 2018, 63, 389–394 CrossRef CAS.
- M. K. Tripathy, D. K. Mahawar and K. R. S. Chandrakumar, Effect of nano-confinement on the structure and properties of water clusters: An ab initio study, J. Chem. Sci., 2020, 132, 7 CrossRef CAS.
- P. C. Sanfelix, S. Holloway, K. W. Kolasinski and G. R. Darling, The structure of water on the (0 0 0 1) surface of graphite, Surf. Sci., 2003, 532–535, 166–172 CrossRef.
- M. Mohammadi, A. Haghtalab and Z. Fakhroueian, Experimental study and thermodynamic modeling of CO2 gas hydrate formation in presence of zinc oxide nanoparticles, J. Chem. Thermodyn., 2016, 96, 24–33 CrossRef CAS.
- R. A. Reichle, K. G. McCurdy and L. G. Hepler, Zinc Hydroxide: Solubility Product and Hydroxy-complex Stability Constants from 12.5–75 °C, Can. J. Chem., 1975, 53, 3841–3845 CrossRef CAS.
- Z. Y. Li, Z. M. Xia, X. Sen Li, Z. Y. Chen, J. Cai, G. Li and T. Lv, Hydrate-Based CO2 Capture from Integrated Gasification Combined Cycle Syngas with Tetra-n-butylammonium Bromide and Nano-Al2O3, Energy and Fuels, 2018, 32, 2064–2072 CrossRef CAS.
- P. Chen, X. Wu, J. Lin and K. L. Tan, High H2 uptake by alkali-doped carbon nanotubes under ambient pressure and moderate temperatures, Science, 1999, 285, 91–93 CrossRef CAS PubMed.
- C. Liu, Y. Y. Fan, M. Liu, H. T. Cong, H. M. Cheng and M. S. Dresselhaus, Hydrogen storage in single-walled carbon nanotubes at room temperature, Science, 1999, 286, 1127–1129 CrossRef CAS PubMed.
- C. Liu, Y. Chen, C. Z. Wu, S. T. Xu and H. M. Cheng, Hydrogen storage in carbon nanotubes revisited, Carbon, 2010, 48, 452–455 CrossRef CAS.
- C. Yu, Study of a Novel Hydrate-based Hybrid Hydrogen Storage Technology, South China University of Technology, 2018 Search PubMed.
- S. R. Firoozabadi, M. Bonyadi and A. Lashanizadegan, Experimental investigation of Fe3O4 nanoparticles effect on the carbon dioxide hydrate formation in the presence of magnetic field, J. Nat. Gas Sci. Eng., 2018, 59, 374–386 CrossRef CAS.
- S. Rajabi Firoozabadi and M. Bonyadi, A comparative study on the effects of Fe3O4 nanofluid, SDS and CTAB aqueous solutions on the CO2 hydrate formation, J. Mol. Liq., 2020, 300, 112251 CrossRef CAS.
- A. Mohammadi, M. Manteghian, A. Haghtalab, A. H. Mohammadi and M. Rahmati-Abkenar, Kinetic study of carbon dioxide hydrate formation in presence of silver nanoparticles and SDS, Chem. Eng. J., 2014, 237, 387–395 CrossRef CAS.
- S. D. Zhou, Y. S. Yu, M. M. Zhao, S. L. Wang and G. Z. Zhang, Effect of Graphite Nanoparticles on Promoting CO2 Hydrate Formation, Energy
and Fuels, 2014, 28, 4694–4698 CrossRef CAS.
- S. Yan, W. J. Dai, S. L. Wang, Y. C. Rao and S. D. Zhou, Graphene oxide: An effective promoter for CO2 hydrate formation, Energies, 2018, 11, 1–13 Search PubMed.
- O. A. Bég, D. E. S. Espinoza, A. Kadir, M. Shamshuddin and A. Sohail, Experimental study of improved rheology and lubricity of drilling fluids enhanced with nano-particles, Appl. Nanosci., 2018, 8, 1069–1090 CrossRef.
- S. Medhi, S. Chowdhury, D. K. Gupta and A. Mazumdar, An investigation on the effects of silica and copper oxide nanoparticles on rheological and fluid loss property of drilling fluids, J. Pet. Explor. Prod. Technol., 2020, 10, 91–101 CrossRef CAS.
- S. Medhi, S. Chowdhury, J. S. Sangwai and D. K. Gupta, Effect of Al2O3 nanoparticle on viscoelastic and filtration properties of a salt-polymer-based drilling fluid, Energy Sources, Part A Recover. Util. Environ. Eff., 2019, 1–13 Search PubMed.
- S. Medhi, S. Chowdhury, A. Kumar, D. K. Gupta, Z. Aswal and J. S. Sangwai, Zirconium oxide nanoparticle as an effective additive for non-damaging drilling fluid: A study through rheology and computational fluid dynamics investigation, J. Pet. Sci. Eng., 2020, 187, 106826 CrossRef CAS.
- S. Medhi, D. K. Gupta and J. S. Sangwai, Impact of zinc oxide nanoparticles on the rheological and fluid-loss properties, and the hydraulic performance of non-damaging drilling fluid, J. Nat. Gas Sci. Eng., 2021, 88, 103834 CrossRef CAS.
- S. Medhi, S. Chowdhury, N. Bhatt, D. K. Gupta, S. Rana and J. S. Sangwai, Analysis of high performing graphene oxide nanosheets based non-damaging drilling fluids through rheological measurements and CFD studies, Powder Technol, 2021, 377, 379–395 CrossRef CAS.
- X. Chang, J. Sun, Z. Xu, F. Zhang, J. Wang, K. Lv and Z. Dai, A novel nano-lignin-based amphoteric copolymer as fluid-loss reducer in water-based drilling fluids, Colloids Surfaces A Physicochem. Eng. Asp., 2019, 583, 123979 CrossRef CAS.
- J. O. Oseh, M. N. A. Mohd Norddin, I. Ismail, A. O. Gbadamosi, A. Agi and H. N. Mohammed, A novel approach to enhance rheological and filtration properties of water–based mud using polypropylene–silica nanocomposite, J. Pet. Sci. Eng., 2019, 181, 106264 CrossRef CAS.
- A. Kazemi-Beydokhti and S. H. Hajiabadi, Rheological investigation of smart polymer/carbon nanotube complex on properties of water-based drilling fluids, Colloids Surfaces A Physicochem. Eng. Asp., 2018, 556, 23–29 CrossRef CAS.
- M. Keshavarz Moraveji, A. Ghaffarkhah, F. Agin, M. Talebkeikhah, A. Jahanshahi, A. Kalantar, S. F. Amirhosseini, M. Karimifard, S. I. Mortazavipour, A. A. Sehat and M. Arjmand, Application of amorphous silica nanoparticles in improving the rheological properties, filtration and shale stability of glycol-based drilling fluids, Int. Commun. Heat Mass Transf., 2020, 115, 104625 CrossRef CAS.
- R. Rafati, S. R. Smith, A. Sharifi Haddad, R. Novara and H. Hamidi, Effect of nanoparticles on the modifications of drilling fluids properties: A review of recent advances, J. Pet. Sci. Eng., 2018, 161, 61–76 CrossRef CAS.
- J. G. Xu, Z. Qiu, X. Zhao, Y. Zhang, G. Li and W. Huang, Application of Nano-Polymer Emulsion for Inhibiting Shale Self-Imbibition in Water-Based Drilling Fluids, J. Surfactants Deterg., 2018, 21, 155–164 CrossRef CAS.
- J. Aramendiz and A. Imqam, Water-based drilling fluid formulation using silica and graphene nanoparticles for unconventional shale applications, J. Pet. Sci. Eng., 2019, 179, 742–749 CrossRef CAS.
- F. Liu, G. C. Jiang, K. Wang and J. Wang, Laponite nanoparticle as a multi-functional additive in water-based drilling fluids, J. Mater. Sci., 2017, 52, 12266–12278 CrossRef CAS.
- R. Wang, T. Liu, F. Ning, W. Ou, L. Zhang, Z. Wang, L. Peng, J. Sun, Z. Liu, T. Li, H. Sun and G. Jiang, Effect of hydrophilic silica nanoparticles on hydrate formation: Insight from the experimental study, J. Energy Chem., 2019, 30, 90–100 CrossRef.
- D. Guo, W. Ou, F. Ning, B. Fang, Y. Liang, S. Ud Din and L. Zhang, Effects of hydrophilic and hydrophobic nano-CaCO3 on kinetics of hydrate formation, Energy Sci. Eng., 2022, 10, 507–524 CrossRef CAS.
- M. Xu, X. Fang, F. Ning, W. Ou, L. Zhang and D. Wang, Effect of hydrophilic silica nanoparticles on hydrate formation during methane gas migration in a simulated wellbore, Petroleum, 2021, 7, 485–495 CrossRef.
- Q. Feng, X. jie Liu, Z. gang Peng, Y. Zheng, J. hua Huo and H. Liu, Preparation of low hydration heat cement slurry with micro-encapsulated thermal control material, Energy, 2019, 187, 116000 CrossRef CAS.
- M. Zheng, X. Wang, Z. Wang, K. Zhou, K. Wang, G. Dong and T. Liu, Influence of cement slurry heat release on physical properties of marine hydrate reservoirs during well cementing, E3S Web of Conferences, 2021, 228, p. 01017, DOI:10.1051/e3sconf/202122801017.
- Ş. Merey, Drilling of gas hydrate reservoirs, J. Nat. Gas Sci. Eng., 2016, 35, 1167–1179 CrossRef.
- M. T. Baig, M. K. Rahman, A. Al-majed and K. Fahd, Application of Nanotechnology in Oil Well Cementing, J. Peroleum Technol., 2017, 1–3 Search PubMed.
- G. Quercia, H. J. H. Brouwers, A. Garnier and K. Luke, Influence of olivine nano-silica on hydration and performance of oil-well cement slurries, Mater. Des., 2016, 96, 162–170 CrossRef CAS.
- M. Bayanak, S. Zarinabadi, K. Shahbazi and A. Azimi, Effects of Nano Silica on oil well cement slurry charactreistics and control of gas channeling, South African J. Chem. Eng., 2020, 34, 11–25 CrossRef.
- A. Thakkar, A. Raval, S. Chandra, M. Shah and A. Sircar, A comprehensive review of the application of nano-silica in oil well cementing, Petroleum, 2020, 6, 123–129 CrossRef.
- M. T. Maagi, S. D. Lupyana and J. Gu, Effect of Nano-SiO2, Nano-TiO2 and Nano-Al2O3 Addition on Fluid Loss in Oil-Well Cement Slurry, Int. J. Concr. Struct. Mater., 2019, 13, 62 CrossRef.
- X. F. Peng, X. L. Yu, L. F. Xia and X. Zhong, Influence factors on suspension stability of nanofluids, Zhejiang Daxue Xuebao, Gongxueban, 2007, 41, 577–580 CAS.
- G. J. Zhang, Y. L. Zhai, G. R. Bao and L. Li, Analysis of Factors Influencing Stability and Viscosity of Nanofluids, J. Mater. Sci. Eng., 2020, 38, 74–80, 87 Search PubMed.
- S. Witharana, J. A. Weliwita, C. Haisheng and W. Liang, Recent Advances in Thermal Conductivity of Nanofluids, Recent Pat. Nanotechnol., 2013, 7, 198–207 CrossRef CAS PubMed.
- B. M. Gu, B. Hou, Z. X. Lu, Z. L. Wang and S. F. Chen, Thermal conductivity of nanofluids containing high aspect ratio fillers, Int. J. Heat Mass Transf., 2013, 64, 108–114 CrossRef CAS.
- C. W. Pang, J. Y. Jung and Y. T. Kang, Thermal conductivity enhancement of Al2O3 nanofluids based on the mixtures of aqueous NaCl solution and CH3OH, Int. J. Heat Mass Transf., 2013, 56, 94–100 CrossRef CAS.
- S. J. Cox, D. J. F. Taylor, T. G. A. Youngs, A. K. Soper, T. S. Totton, R. G. Chapman, M. Arjmandi, M. G. Hodges, N. T. Skipper and A. Michaelides, Formation of Methane Hydrate in the Presence of Natural and Synthetic Nanoparticles, J. Am. Chem. Soc., 2018, 140, 3277–3284 CrossRef CAS PubMed.
- Y. J. Bai, Y. Q. Huang, G. S. Cao, X. H. Nan, Q. C. Cheng, L. Wang and T. Du, Microparticle effect of carbon dioxide hydrate crystal nucleus in reaction kettle, J. Renew. Mater., 2021, 9, 651–669 CAS.
- S. Y. Nahri, J. L. Nielsen and Y. Chen, Effect of nanoparticles on the nucleation and agglomeration rates of hydrate growth using THF–water clathrates, Pet. Sci., 2020, 17, 467–476 CrossRef CAS.
- J. E. Cendales, M. Yin and P. Englezos, Thermodynamics and kinetics of CO2 hydrate formation in the presence of cellulose nanocrystals with statistical treatment of data, Fluid Phase Equilib, 2021, 529, 112863 CrossRef CAS.
- A. Bozorgian, Investigation of the effect of Zinc Oxide Nano-particles and Cationic Surfactants on Carbon Dioxide Storage capacity, Adv. J. Chem. B Nat. Prod. Med. Chem., 2021, 3, 54–61 CAS.
- Y. M. Song, F. Wang, G. Q. Liu, S. J. Luo and R. B. Guo, Promotion Effect of Carbon Nanotubes-Doped SDS on Methane Hydrate Formation, Energy and Fuels, 2017, 31, 1850–1857 CrossRef CAS.
- F. Wang, Y. M. Song, G. Q. Liu, G. Guo, S. J. Luo and R. B. Guo, Rapid methane hydrate formation promoted by Ag&SDS-coated nanospheres for energy storage, Appl. Energy, 2018, 213, 227–234 CrossRef CAS.
- X. Y. Zhang and S. D. Zhou, Effect of GO/GN and SDS compound system on formation characteristics of CO2 hydrate, Nat. Gas Chem. Ind., 2021, 46, 2–7 Search PubMed.
- K. R. Chaturvedi, A. Pandey, R. Kumar and T. Sharma, Role of hydrogen to promote hydrate formation of flue gas mixture of CO2 and N2 in silica nanofluid of single-step origin, J. Environ. Chem. Eng., 2021, 9, 106591 CrossRef CAS.
- Y. Y. Lu, B. Bin Ge and D. L. Zhong, Investigation of using graphite nanofluids to promote methane hydrate formation: Application to solidified natural gas storage, Energy, 2020, 199, 117424 CrossRef CAS.
- J. B. Li, D. L. Zhong and J. Yan, Improving gas hydrate-based CH4 separation from low-concentration coalbed methane by graphene oxide nanofluids, J. Nat. Gas Sci. Eng., 2020, 76, 103212 CrossRef CAS.
- N. Liu, L. T. Chen, C. X. Liu, L. Yang and D. P. Liu, Experimental study of carbon dioxide hydrate formation in the presence of graphene oxide, Energy, 2020, 211, 118994 CrossRef CAS.
- L. J. Jiao, R. C. Wan and Z. L. Wang, Experimental Investigation of CO2 Hydrate Dissociation in Silica Nanoparticle System with Different Thermal Conductivity, Int. J. Thermophys., 2021, 42, 1–18 CrossRef.
- J. Min, D. W. Kang, W. Lee and J. W. Lee, Molecular Dynamics Simulations of Hydrophobic Nanoparticle Effects on Gas Hydrate Formation, J. Phys. Chem. C, 2020, 124, 4162–4171 CrossRef CAS.
- P. Hu, W. Ke and D. Y. Chen, Molecular mechanism for methane hydrate nucleation on corroded iron surface, Chem. Eng. Sci., 2022, 249, 117303 CrossRef CAS.
- S. Said, V. Govindaraj, J. M. Herri, Y. Ouabbas, M. Khodja, M. Belloum, J. S. Sangwai and R. Nagarajan, A study on the influence of nanofluids on gas hydrate formation kinetics and their potential: Application to the CO2 capture process, J. Nat. Gas Sci. Eng., 2016, 32, 95–108 CrossRef CAS.
- Y. J. Wu, T. Q. Tang, L. Shi and Y. R. He, Rapid hydrate-based methane storage promoted by bilayer surfactant-coated Fe3O4 nanoparticles under a magnetic field, Fuel, 2021, 303, 121248 CrossRef CAS.
- H. Pahlavanzadeh, S. Rezaei, M. Khanlarkhani, M. Manteghian and A. H. Mohammadi, Kinetic study of methane hydrate formation in the presence of copper nanoparticles and CTAB, J. Nat. Gas Sci. Eng., 2016, 34, 803–810 CrossRef CAS.
- M. Abdi-Khanghah, M. Adelizadeh, Z. Naserzadeh and H. Barati, Methane hydrate formation in the presence of ZnO nanoparticle and SDS: Application to transportation and storage, J. Nat. Gas Sci. Eng., 2018, 54, 120–130 CrossRef CAS.
- M. Mohammadi, A. Haghtalab and Z. Fakhroueian, Experimental study and thermodynamic modeling of CO2 gas hydrate formation in presence of zinc oxide nanoparticles, J. Chem. Thermodyn., 2016, 96, 24–33 CrossRef CAS.
- S. Arjang, M. Manteghian and A. Mohammadi, Effect of synthesized silver nanoparticles in promoting methane hydrate formation at 4.7 MPa and 5.7 MPa, Chem. Eng. Res. Des., 2013, 91, 1050–1054 CrossRef CAS.
- M. Rahmati-Abkenar, M. Manteghian and H. Pahlavanzadeh, Experimental and theoretical investigation of methane hydrate induction time in the presence of triangular silver nanoparticles, Chem. Eng. Res. Des., 2017, 120, 325–332 CrossRef CAS.
- M. Rahmati-Abkenar, M. Manteghian and H. Pahlavanzadeh, Nucleation of ethane hydrate in water containing silver nanoparticles, Mater. Des., 2017, 126, 190–196 CrossRef CAS.
- J. W. Choi, J. T. Chung and Y. T. Kang, CO2 hydrate formation at atmospheric pressure using high efficiency absorbent and surfactants, Energy, 2014, 78, 869–876 CrossRef CAS.
- Y. Liu, X. R. Liao, C. R. Shi, Z. Ling and L. L. Jiang, Promoting and Inhibitory Effects of Hydrophilic/Hydrophobic Modified Aluminum Oxide Nanoparticles on Carbon Dioxide Hydrate Formation, Energies, 2020, 13, 5380 CrossRef CAS.
- J. Pasieka, S. Coulombe and P. Servio, Investigating the effects of hydrophobic and hydrophilic multi-wall carbon nanotubes on methane hydrate growth kinetics, Chem. Eng. Sci., 2013, 104, 998–1002 CrossRef CAS.
- Z. C. Cheng, Y. C. Zhao, W. G. Liu, Y. Liu, L. L. Jiang and Y. C. Song, Kinetic analysis of nano-SiO2 promoting methane hydrate formation in porous medium, J. Nat. Gas Sci. Eng., 2020, 79, 103375 CrossRef CAS.
- S. Said, V. Govindaraj, J. M. Herri, Y. Ouabbas, M. Khodja, M. Belloum, J. S. Sangwai and R. Nagarajan, A study on the influence of nanofluids on gas hydrate formation kinetics and their potential: Application to the CO2 capture process, J. Nat. Gas Sci. Eng., 2016, 32, 95–108 CrossRef CAS.
- A. M. Javidani, S. Abedi-Farizhendi, A. Mohammadi, H. Hassan, A. H. Mohammadi and M. Manteghian, The effects of graphene oxide nanosheets and Al2O3 nanoparticles on the kinetics of methane + THF hydrate formation at moderate conditions, J. Mol. Liq., 2020, 316, 113872 CrossRef CAS.
|
This journal is © The Royal Society of Chemistry 2022 |
Click here to see how this site uses Cookies. View our privacy policy here.