DOI:
10.1039/D2RA02756A
(Paper)
RSC Adv., 2022,
12, 16604-16614
A windowed carbon nanotube membrane for CO2/CH4 gas mixture penetration separation: insights from theoretical calculation
Received
30th April 2022
, Accepted 12th May 2022
First published on 6th June 2022
Abstract
A new class of species-permselective molecular sieves with functionalized nanowindows has been prepared by modifying the armchair single-walled carbon nanotubes (SWNTs) of a pillared graphene membrane, namely windowed carbon nanotube membrane. The mechanism and characteristics of the windowed carbon nanotube membrane for the selective separation of the CO2/CH4 gas mixture are comprehensively and deeply studied. Selective gas separation has a great dependence not only on the interaction of the gas adsorbing on the graphene membrane and inside the CNT channel but also with the energy barrier for the gas diffusing through the nanowindow. In all the functional nanowindows investigated, CH4 is completely rejected by the N/F-modified nanowindows while maintaining extremely high CO2 permeability. The CO2 permeance of the nanowindows is as high as 109 GPU. It emerged that these windowed carbon nanotube membranes are efficient species-selective molecular sieves possessing excellent CO2/CH4 selectivity and brilliant CO2 capture capability.
1. Introduction
As a mixture containing complex substances, raw natural gas contains several impurities that can increase the gas transmission resistance, which affects its value and prospect in commercial application.1–3 To meet the environmental standards and calorific value specifications of renewable energy supplements, the undesirable constituents, such as CO2, must be removed before natural gas is delivered to the pipeline network.4 To achieve this target, natural gas purification needs to be conducted before its commercial application, which involves the CO2/CH4 gas mixture separation processes. CO2/CH4 gas mixture separation is also vital in landfill gas recovery and improving oil recovery.5,6 Simultaneously, the captured CO2 can be used as a chemical raw material for industrial precursors such as syngas, polycarbonate, and polyurethane. Hence, it is a meaningful work to explore effective materials and methods for CO2/CH4 gas mixture separation and CO2 capture.
Compared with other species purification methods, including supersonic separation,7 adsorption,8 and cryogenic separation,9 membrane separation technology is regarded as superior to its rivals10–12 owing to its advantages of low energy consumption, high efficiency, facile operation, and high tunability. Membranes can be used to separate and capture species according to the different characteristics such as molecular diameter, pore size, permeability coefficient, and charge.13–15 The graphene-based molecular sieve membranes generally exhibited ultrahigh species flux and selectivity, which far exceed other existing membranes by several orders of magnitude.16–20 In this article, graphene-based composite materials are selected as molecular sieve membranes for CO2/CH4 gas mixture separation and CO2 capture.
The permeation and diffusion of the CO2/CH4 gas mixture through carbon molecular sieve membranes can be impacted dramatically by many factors, including the functionalization of the pore rim and surface.21–23 This is because the functionalization of carbonaceous materials can tailor the interaction energy between the molecular sieve and the species, which may facilitate or hinder the diffusion of certain species. For example, Xue et al.24 found that the selectivity of CO2 over N2 can be significantly improved by N-functionalized pores because of the enhanced electrostatic interactions when CO2 permeates through the functionalized pores. Lu et al.25 also reported that the permeability of CO2 can be dramatically changed by N-substitutional doping, which can change the electroneutrality of the polyphenylene membrane, resulting in an enhancement of the diffusion barrier for CO2. Bai et al.18 investigated the CO2/CH4 separation performance of N-functionalized nanoporous graphene membrane through theoretical calculations. They found that the configuration of the pore can be changed significantly by the chemical functionalizations, which can achieve high permeability and selectivity for separating the CO2/CH4 gas mixture. In this article, N/F-functionalized pores are adopted to separate the CO2/CH4 gas mixture and capture CO2 in graphene-based composite materials.
The fast diffusion and separation of species through CNT has been demonstrated in previous studies.18,25 To combine the advantages of CNT and graphene membrane, we proposed a new type of carbon molecular sieve—windowed carbon nanotube membrane—for gas transport and separation. Different styles of functionalized nanowindows are designed on the wall of SWNTs, and a multi-scale computational study was implemented to explore the species separation mechanism and performance of the windowed carbon nanotube membrane. Such a system has two advantages: (1) the graphene sheet can provide an efficient adsorption isolating membrane for the gas, which can adsorb species during gas separation and serve as a storage space and transportation channel for species after gas permeation through the functionalized pores, and the CNT can provide a fast-transport channel for the retentate gas after gas mixture separation; (2) the acceptance of different kinds of gas molecules by CNT and nanowindow after gas separation can yield high species permeability and selectivity.
The theme of this paper is to implement a systematic and comprehensive exploration on the permeation and separation characteristics of CO2/CH4 gas mixtures in a new type of carbon molecular sieve, which are mimicked by windowed carbon nanotube membranes with different window sizes and functional groups using the DFT method and MD simulations. To begin with, the separation mechanism of the CO2/CH4 gas mixture in the windowed carbon nanotube membrane and the corresponding electronic properties are investigated by performing density functional theory (DFT) calculations. Subsequently, the separation characteristics of the CO2/CH4 gas mixture under different conditions are elucidated with molecular dynamics (MD) simulation in detail. Finally, the results are summarized at the end of the study.
2. Model and computational details
2.1. Model
The windowed carbon nanotube membrane model was envisaged as a perpendicular combination of an armchair (8, 8) windowed SWNT and bilayer graphene sheets, as presented in Fig. 1. The graphene sheets are arranged in the AA mode. The length and diameter of the CNT are set at 2 nm and 1.085 nm, respectively. The CNT decorated with nanowindows works is used as gas diffusion and separation channels, and the graphene sheet is used as a gas adsorption membrane and isolation boundary. Pairs of nanowindows opposite each other are designed on the middle position of the CNT wall as follows: the window is created by removing carbon atoms on pristine CNT and named according to the number of drilled carbon atoms.26
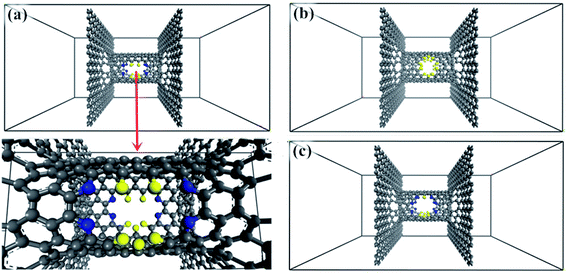 |
| Fig. 1 Construction of windowed carbon nanotube membrane model. (a) 4N5F-pore-13, (b) all-F-pore-16, and (c) 6N4F-pore-16 (gray balls represent carbon atom, blue balls represent nitrogen atom, and yellow balls represent fluorine atom). | |
2.2. DFT calculations
DFT calculations were performed by the DMol3 module in the Material Studio software to calculate the adsorption energy of gas molecules on the graphene membrane, the interaction between the gas molecules and modified CNTs, and the energy barrier of gas molecules passing through the nanowindows.27,28 Periodic boundary conditions on all the models were taken into account. Generalized gradient approximation (GGA) with the Perdew–Burke–Ernzerhof (PBE) functional was conducted to describe the exchange–correlation interaction.29–31 To make dispersion correction for DFT, Grimme's method was adopted.32 A double numeric quality with polarization functions (DNP) basis set was employed to expand the electronic wave functions. Self-consistent field (SCF) was employed with a convergence criterion of 10−6 a.u.33,34 The vacuum thickness for the systems is set to 30 Å to avoid interactions between the adjacent models. All models were continuously optimized until the energy, maximum force, and displacement were less than 1 × 10−5 Ha, 0.002 Ha Å−1, and 0.005 Å, respectively. The interaction energy (Eint) can be defined as |
Eint = Emodel+gas − (Emodel + Egas)
| (1) |
where Emodel+gas, Emodel, and Egas are the total energies after gas molecules interacting with the model, the energy of the model, and the energy of the isolated gas molecule, respectively.
2.3. MD simulations
MD simulations were conducted to elaborate on the CO2/CH4 separation characteristics of windowed carbon nanotube membrane. The windowed carbon nanotube membrane of area 3 nm × 3 nm × 2 nm divided the simulation box into three chambers (Fig. 1). The simulation box of height 6 nm contained 100 molecules for the CO2/CH4 gas mixtures, i.e., 50 for CO2 molecules and 50 for CH4 molecules. Periodic boundary conditions were set in the directions parallel to the graphene sheet to avoid the departure of species outside the simulation box during MD simulation, while reflective wall boundary conditions were applied in the direction perpendicular to the graphene sheet. Each simulation was run for 6 × 107 timesteps with a time step of 1 fs. NVT ensemble was adopted at 298 K controlled by the Nose method. The COMPASS force field in the Forcite module was chosen to express the interatomic interactions.35 The electrostatic interactions were calculated by the Ewald method with the accuracy of 0.001 kcal mol−1, while the van der Waals interaction was treated with the atom-based option with a cutoff distance of 12.5 Å.31,36,37 During MD simulations, the windowed carbon nanotube membrane was modeled as a fully flexible structure. To avert the vertical displacement of the windowed carbon nanotube membrane caused by the collisions with the gas particles, one “central” carbon atom in the graphene membrane is immobilized. In this way, the vibration of other atoms in the windowed carbon nanotube membrane in response to collisions with the gas molecules can be retained by this fixed carbon atom. The simulation data was collected every 5 ps for property analysis. To ensure the accuracy of MD simulations, each simulation was repeated three times under the same settings.
3. Results and discussion
Screened by preliminary MD results, three excellent gas separation nanowindows, namely, 4N5F-pore-13, all-F-pore-16, and 6N4F-pore-16, were selected from a series of functionalized pore configurations, as shown in Fig. 1. The nanowindows have a pore size of 3.553, 3.443, and 3.795 Å, respectively, for 4N5F-pore-13, all-F-pore-16, and 6N4F-pore-16, as shown in Fig. 2. These pore sizes are higher than the kinetic diameter of the CO2 molecule (CO2: 3.3 Å) but lower than the kinetic diameter of the CH4 molecule (CH4: 3.8 Å). The delicate relationship between the gas molecule's kinetic diameter and the pore size makes windowed carbon nanotube membrane an ideal CH4-isolating and CO2-capturing apparatus, which can result in the efficient separation of the CO2/CH4 gas mixture. The atomic distance and bond angle of optimized nanowindows are given in Table 1. The atomic distances of C–C, C–N, and C–F are denoted by lC–C, lC–N, and lC–F, respectively. The bond angles are denoted by ∠C–N–C. Since the substitution of N atoms at the C sites on the nanowindows leads to a distorted pore structure after optimization, lC–C and lC–N are varied in the range of 1.398–1.4822 Å and 1.332–1.359 Å, respectively. ∠C–N–C is varied in the range of 117.937° to 119.344°.
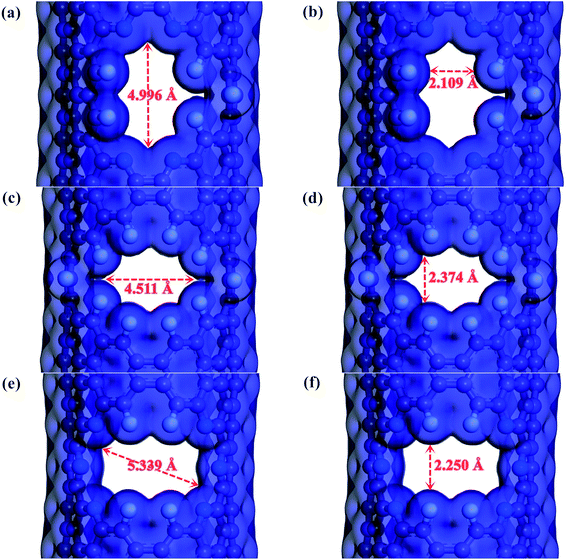 |
| Fig. 2 Window electron density isosurface of the windowed carbon nanotube membrane model: (a, b) the maximum and minimum internal distance of the 4N5F-pore-13, respectively; (c, d) the maximum and minimum internal distance of the all-F-pore-16, respectively; (e, f) the maximum and minimum internal distance of the 6N4F-pore-16, respectively. Atom color code: carbon, grey; nitrogen, blue; fluorine, cyan. | |
Table 1 Summary of structural parameters of various nanowindows
Model |
lC–C (Å) |
lC–N (Å) |
lC–F (Å) |
∠C–N–C (°) |
4N5F-window-13 |
1.398–1.465 |
1.333–1.350 |
1.337–1.338 |
117.952–118.988 |
all-F-window-16 |
1.400–1.480 |
None |
1.340–1.343 |
None |
6N4F-window-16 |
1.412–1.482 |
1.332–1.359 |
1.332 |
117.937–119.344 |
3.1. Analysis of interaction energy between the gas molecule and the model
3.1.1. Adsorption energy of the gas molecule on the graphene surface. The knowledge of the adsorbed states of CO2 and CH4 on the graphene surface is very important to clarify the mechanisms of CO2/CH4 gas mixture diffusive separation on graphene materials and to obtain the design guidelines for carbon molecular sieves with CO2/CH4 gas mixture separation. The adsorbed states for different kinds of gas molecules when the configuration has the lowest energy are presented in Fig. 3. The DFT calculation results demonstrate that the CO2 molecule is favorably adsorbed parallel to the graphene membrane, while the CH4 molecule prefers to be adsorbed at the top of the carbon atom of the graphene membrane. The optimized configuration of CO2 adsorption on the graphene surface is exhibited in Fig. 3(a). The C atom of CO2 is located in the middle of the C–C bond of the benzene ring and the O atom of CO2 approaches the center of the benzene ring. The adsorption energy of the CO2 molecule is about −7.60 kcal mol−1. The shortest distance between the carbon atom of CO2 and the graphene surface is predicted to be 3.23 Å. The adsorption configuration of the CH4 molecule on the graphene plane is also displayed in Fig. 3(b). The C atom of CH4 is located on top of the C atom of the graphene membrane and three H atoms of CH4 point toward the graphene membrane. The calculated adsorption energy and height of the CH4 molecule are −7.42 kcal mol−1 and 3.34 Å, respectively. The adsorption of CO2 and CH4 on the graphene surface are thus categorized into physisorption. The discrepancy of gas adsorption energy results in the selective adsorption of the CO2 molecule by the graphene membrane, which can promote the subsequent permeation of the CO2 molecule in the CNT channel and inhibit the approach of the CH4 molecule around the molecule sieves.
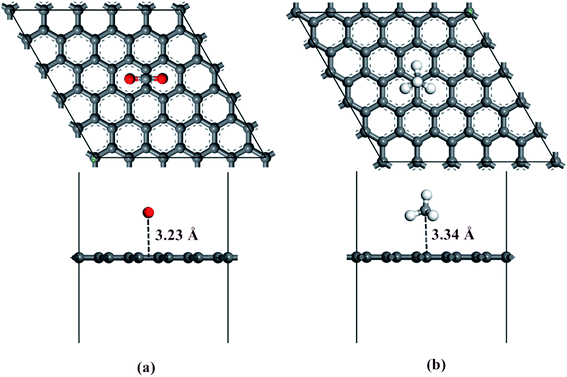 |
| Fig. 3 The optimized configurations of CO2 and CH4 physisorption on the graphene surface. The adsorption of (a) CO2 and (b) CH4 on the graphene surface. Atom color code: carbon, grey; hydrogen, white; oxygen, red. | |
3.1.2. Interaction energy between the gas molecule and the windowed carbon nanotube channel. The interaction energy between the gas molecule and CNT also dramatically affects the diffusion order of the species in molecular sieves. The central position of CNT is defined as zero point, and the direction of the coordinate axis is along the CNT. When diffusing in the CNT, the CO2 molecule is parallel to the CNT, while three H atoms of the CH4 molecule in a plane are parallel to the graphene membrane. Note that the interaction energy between the gas molecules and the windowed carbon nanotube channel shows a decreasing trend with the diffusion of the gas molecule to the middle of the channel, as shown in Fig. 4. The values of the interaction energy between the gas molecules and windowed carbon nanotube channels have a good symmetry corresponding to the nanowindows. The energy minimization of the gas molecule is achieved at or near the middle of CNTs, which is caused by the vdW forces and electrostatic interactions between the gas molecules and defects formed on the wall of the CNTs. The results show that the interaction between the CO2 molecule and CNTs is stronger in all the windowed carbon nanotube models. The discrepancy in the interaction energy allows CO2 to enter the CNTs before CH4.
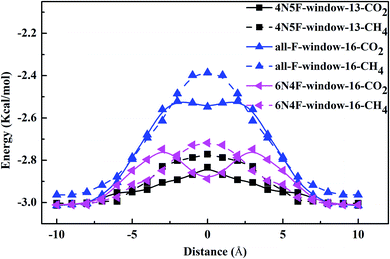 |
| Fig. 4 The interaction energy curve between different gas molecules and windowed carbon nanotube channels. | |
3.1.3. Interaction energy between the gas molecule and the nanowindow. The interaction energy between the gas molecule and the nanowindow was also calculated by eqn (1) provided in Section 2. The line from the carbon atom of the gas molecule to the center of the nanowindow is defined as the X axis, which is also known as the adsorption height. Fig. 5 comprehensively shows the resulting interaction energies of CO2 and CH4 molecules with three different nanowindows. Different values on the X axis indicate the distance of the gas molecules from the nanowindow. The negative values on the X axis represent gas molecules that move within the nanotube cavity. The result reflects that all the nanowindows show attractive potential energy wells to the CO2 molecules, which indicates that it is a spontaneous process for CO2 molecules to approach the nanowindow. Therefore, CO2 molecules must overcome the attractive potential energy wells to successfully penetrate the nanowindows. Meanwhile, we can monitor that the CO2 molecules frequently penetrate forward or return back the 4N5F-pore-13 and 6N4F-pore-16 during CO2/CH4 gas mixtures separation (see Fig. 7). Accordingly, the highest adsorption energy is achieved for CO2 molecules passing through 6N4F-pore-16, whereas the lowest one is achieved for CO2 molecules passing through all-F-pore-16. The Eint for CO2 molecules passing through 4N5F-pore-13, all-F-pore-16, and 6N4F-pore-16 are −0.2305, −0.1797, and −0.2422 eV, respectively. Comparing the all-F-pore-16, the Eint for 4N5F-pore-13 and 6N4F-pore-16 significantly increases about 28% and 35%, respectively. In contrast, the Eint dramatically increases when the carbon atom of the CH4 molecules is close to the center of the pore, which means that CH4 molecules must overcome the repulsive potential energy barrier to penetrate the nanowindows. The specifically high attractive potential energy between the CH4 molecules and nanowindows can obviously increase the CO2 molecules crossing the probability through the single-atom-thick nanowindows so as to obtain excellent CO2 selectivity.
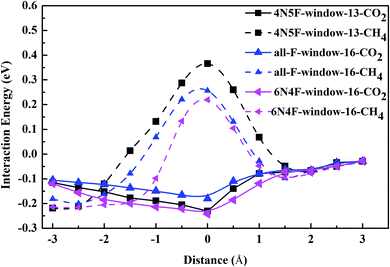 |
| Fig. 5 The interaction energy curve between different gas molecules and nanowindows during gas permeation through the nanowindows. | |
The electron density isosurfaces of the CO2 and CH4 molecules interacting with different nanowindows are also measured to elucidate the origin of the energy difference during gas penetration through the windows in more detail. As shown in Fig. 6, CO2 molecules show no overlap with all three nanowindows, whereas CH4 molecules show obvious overlap with all three nanowindows significantly, resulting in a large energy difference when different kinds of gas molecules pass through the functionalized nanowindows. The linear interpenetration of CO2 passing the nanowindows is observed in all windowed carbon molecular sieves, while CH4 is completely rejected by all the nanowindows. Due to its smaller size, CO2 diffusion is favored energetically compared to CH4 (Fig. 5). Moreover, CO2 passes through the nanowindows by orienting its molecular axis along the window centers, as shown in Fig. 6. Considering the permeation mode of linear molecules in CNTs, CO2 molecules typically rotate in the direction of the CNT channel and then perpendicularly through the nanowindow, which is favored in the entropic selectivity. In these circumstances, the CH4 molecule is completely blocked out of the nanowindow.
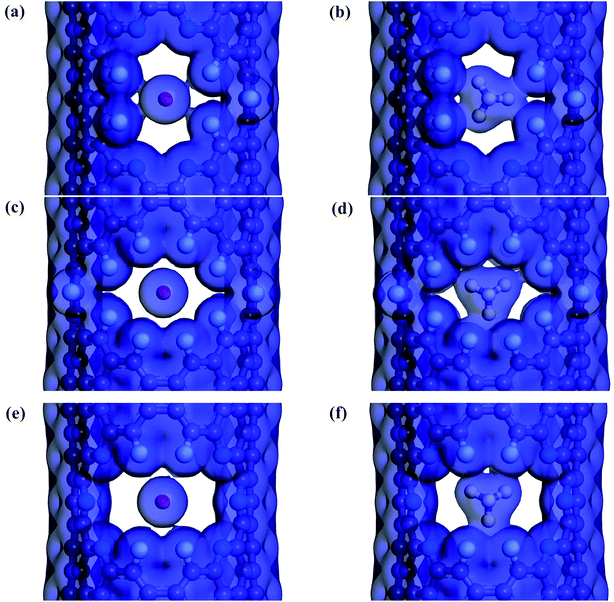 |
| Fig. 6 Electron density isosurface for different kinds of gases penetrating through the nanowindows of (a) and (b) 4N5F-pore-13, (c) and (d) all-F-pore-16, and (e) and (f) 6N4F-pore-16, respectively (isovalue of 0.02 e Å−3). Atom color code: carbon, grey; nitrogen, blue; fluorine, cyan. | |
To sum up, according to the detailed investigation and comparison of the interaction energy between the gas molecules and the windowed carbon nanotube membranes, we can find that the preferential adsorption and permeation of CO2 contribute partially to the gas mixture separation, while the interaction energy difference in the process of the penetrating nanowindow is the most important determinant in the separation process. Based on the energy barrier information, MD simulation was then performed to study the gas separation process for various molecular sieve models.
3.2. The CO2/CH4 separation performance
The filtered molecular numbers of the gas through different functionalized nanowindows as a function of time is shown in Fig. 7 and the final snapshots of the CO2/CH4 gas mixture separation through the nanowindows are presented in Fig. 8. Several interesting phenomena can be concluded: (1) among the various N/F modified nanowindows investigated, only CO2 diffusion is observed; CH4 is completely rejected by the nanowindows. (2) For all time-dependent CO2 penetration curves (Fig. 7), the uphill straight line reflects the same penetration trend of CO2 in the initial time region of the pre-equilibrium stage. (3) The permeability of CO2 increases significantly with the increase in the effective pore size. The maximum permeability of CO2 can be observed with 6N4F-pore-16. (4) The fluctuation of the CO2 permeation curve also increases with the expansion of the effective pore size, which is due to the increased possibility of the crossing-back motions of the gas molecules from the feed side to the permeate side. The MD results qualitatively agree well with the speculation provided by DFT calculations. Gas separation through the windowed carbon nanotube membranes involves adsorption and diffusion, and the selectivity is mainly dictated by the interaction energy between the gas molecules and nanowindows. Also, the aggregation of the diffusing particles around the graphene surface region was observed in this study. The permeated CO2 molecules spend almost all of their time next to the graphene surface, where they exhibit pressure-independent surface diffusion.
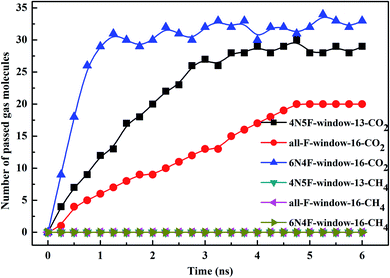 |
| Fig. 7 The number of diffusing species in different windowed carbon nanotube membranes as a function of the MD simulation time. | |
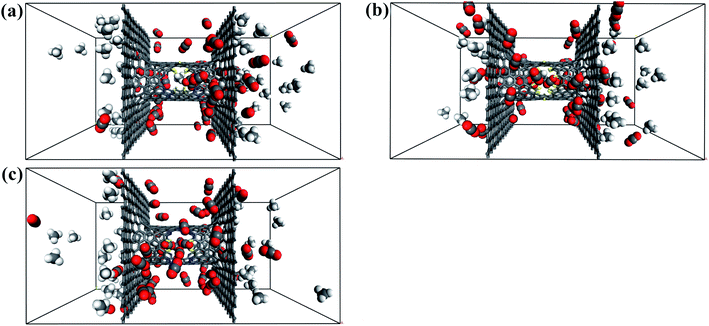 |
| Fig. 8 Final snapshots of the CO2/CH4 gas mixture separating through the windowed carbon nanotube membranes (a) 4N5F-pore-13, (b) all-F-pore-16, and (c) 6N4F-pore-16. | |
Gas permeance was employed as another criterion to estimate the performance of the windowed carbon nanotube membrane, which was calculated using eqn (2)
|
 | (2) |
where
Px represents the permeance of gas x,
Nx represents the permeate rate of the gas x, and
S represents the area of the windowed carbon molecular sieve. Δ
Px is set by default to 1 × 10
5 Pa.
38 The permeance of the species with different nanowindows is listed in
Table 2. Compared to previous studies on porous graphene or windowed carbon nanotube gas separation systems, our gas permeability is increased by 10
4–10
7 orders of magnitude.
18,22,39,40 In addition, the separated gas flows to different permeate sides to avoid mixing with the gas in the original feed side, ensuring that the gas mixture can be separated continuously.
Table 2 The permeance of species with different nanowindows
Molecules |
4N5F-window-13 |
all-F-window-16 |
6N4F-window-16 |
CO2 |
1.50 × 109 GPU |
9.31 × 108 GPU |
1.71 × 109 GPU |
CH4 |
0 |
0 |
0 |
3.3. MD simulation of the CO2/CH4 separation mechanism of the windowed carbon nanotube membrane
To test and verify the CO2/CH4 separation mechanism of the windowed carbon nanotube membrane obtained by DFT calculation, the adsorption regions of gas molecules in the different models were studied, and the final number distribution of the gas molecules along the direction perpendicular to the graphene sheet is plotted in Fig. 9. According to the results of gas separation, five gas adsorption regions are formed in the windowed carbon nanotube membrane (region I to region V). For CO2 molecules with higher adsorption intensity, apart from being captured by CNTs, several molecules are adsorbed on the graphene surface (the graphene membrane is located at z = −10 and 10 Å). These CO2 molecules firstly reach region I and then migrate to the vicinity of the functionalized carbon nanotube mouth or are directly adsorbed into the functionalized carbon nanotube before they cross the nanowindows. Meanwhile, region III accumulated by the blockage of CO2 molecules near the nanowindows of the windowed carbon nanotube membrane also plays an important role in CO2 diffusion characteristics. These CO2 molecules have a good barrier effect on the penetration of the CH4 molecule. Nearly all the permeated CO2 molecules are adsorbed in region II and region IV. The penetration of CO2 molecules through the nanowindow leads to a large number of CH4 molecules adsorbing in region I and region V, and there is no CH4 molecule crossing event that occurs in the nanowindow, thus significantly improving the CO2/CH4 selectivity. According to the study on the adsorption mechanism, the windowed carbon nanotube membranes show preferential adsorption toward CO2, which is mainly attributed to vdW force and electrostatic interaction, which gives CO2 molecules more chances to approach the nanowindows. The competitive adsorption mechanisms are beneficial for CO2 permeation. It can also be seen from the separation results in Fig. 9(a) and (c) that regions II–IV are basically CO2 molecules, and the CH4 molecules in them only represent that they happen to be in the CNT channels. After gas separation, the retentate gas in permeate side (10–30 Å regions) are basically CH4 molecules. In terms of the gas separation effect, 4N5F-pore-13 and 6N4F-pore-16 are ideal.
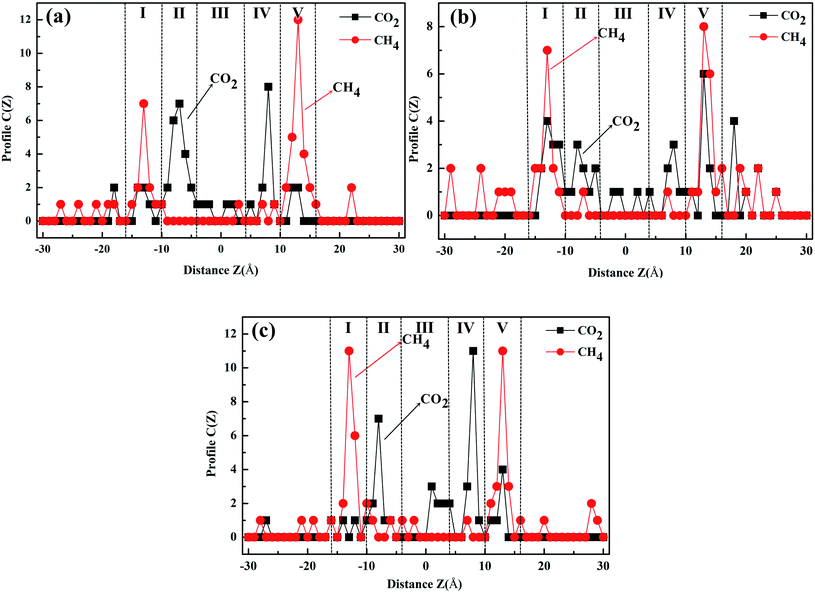 |
| Fig. 9 The final number distribution curves of gas molecules along the direction perpendicular to the graphene sheet for the windowed carbon nanotube membrane with different nanowindows. (a) 4N5F-pore-13, (b) all-F-pore-16, and (c) 6N4F-pore-16. | |
3.4. Effect of the pressure difference on the CO2 permeability
In this section, the effect of pressure difference on CO2/CH4 permeation and separation in the windowed carbon nanotube membrane is investigated by MD simulations. For comparison, we calculated the number and permeance of species crossing the nanowindows when equimolar CO2/CH4 gas mixture was placed on both sides of the windowed carbon nanotube membrane, as displayed in Fig. 10 and Table 3, respectively. The striking selective permeation of CO2 passing through the nanowindows is observed in the simulations. This result means that the high surface affinity of the model and the small kinetic diameter of the CO2 molecule are able to ensure the selective penetration of CO2, regardless of the starting configuration of the gas mixture. The final snapshots of the CO2/CH4 gas mixture separating through the nanowindows are presented in Fig. 11. However, the results reported in Table 3 show that the permeability of CO2 through the nanowindows decreased. This is because when the pressure difference on both sides of the model disappears, the fluidity of the species is weakened, inhibiting the diffusion and permeation of the gas. Moreover, the smaller the pore size of the nanowindow, the more obvious the downward trend of permeability. This is because the smaller the pore size, the smaller the adsorption force acting on the CO2 molecules. The all-F-pore-16 has the least affinity for CO2 molecules, which leads to the most significant reduction in the number of gas molecules that permeate when the molecular motion slows down. This proves once again the important role of nanowindows in gas separation, especially for models with small-sized nanowindows.
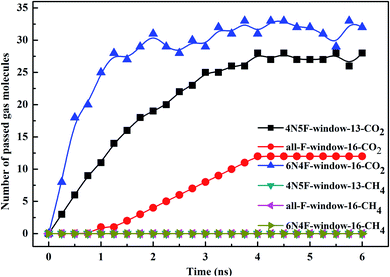 |
| Fig. 10 The number of diffusing species in different windowed carbon nanotube membranes obtained without pressure difference. | |
Table 3 The permeance of species with different nanowindows
Molecules |
4N5F-window-13 |
all-F-window-16 |
6N4F-window-16 |
CO2 |
1.40 × 109 GPU |
6.21 × 108 GPU |
1.66 × 109 GPU |
CH4 |
0 |
0 |
0 |
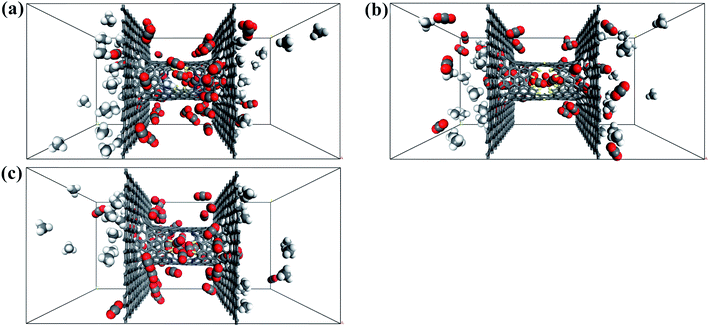 |
| Fig. 11 Final snapshot of the CO2/CH4 gas mixture separating through the windowed carbon nanotube membranes. (a) 4N5F-pore-13, (b) all-F-pore-16, and (c) 6N4F-pore-16. | |
4. Conclusion
In this article, a comprehensive exploration of the CO2/CH4 gas mixture separation mechanism and the property of windowed carbon nanotube membrane are provided. All the simulation results manifest that the windowed carbon nanotube membrane with N/F atoms functionalized on the pore rim can act as a filtration membrane for the separation or enrichment of CO2/CH4 gas mixtures with remarkably high CO2 permeability and selectivity. The differences in the interaction between the gas molecules and the windowed carbon nanotube membrane, especially the strong repulsive interaction between CH4 molecules and nanowindows, resulted in 100% CO2/CH4 selectivity. It can be deduced that 6N4F-pore-16 has the best permeability on the diffusion of CO2 molecules in all the simulated windowed carbon nanotube membrane models. Detailed analysis of the simulated systems reveals that the difference in the interaction potential energy with graphene sheet and CNT, and the electron density overlap of the nanowindow facilitate CO2/CH4 gas mixture separation through the windowed carbon nanotube membrane.
Conflicts of interest
There are no conflicts to declare.
Acknowledgements
We thank the financial support from the National Natural Science Foundation of China (11774248 and 11905076) and the Fundamental Research Funds for the Central Universities, Southwest Minzu University (2020PTJS28002). Meanwhile, we are grateful to the support of our calculation from Analytical & Testing Center Sichuan University, P. R. China.
References
- H. Zhou, C. Qu, G. Lu, Z. Li and Y. Feng, Deliquification of Low-Productivity Natural Gas Wells with In Situ Generated Foams and Heat, Energy Fuel., 2021, 35, 9873–9882 CrossRef CAS.
- X. Wu, S. Niu and C. Li, The study of the dynamic response of the natural gas pipeline aerial crossing during pigging process: a review, J. Fluid Struct., 2021, 105, 103339 CrossRef.
- M. Fakhroleslam, R. B. Boozarjomehry, A. M. Sahlodin, G. Sin and S. S. Mansouri, Dynamic Simulation of Natural Gas Transmission Pipeline Systems through Autoregressive Neural Networks, Ind. Eng. Chem. Res., 2021, 60, 9851–9859 CrossRef CAS.
- S. Chen, M. Tian, Z. Tao, Y. Fu and B. Xiao, Effect of swing on removing CO2 from offshore natural gas by adsorption, Chem. Eng. J., 2019, 382, 122932 CrossRef.
- T. B. Thomasen, C. Scheutz and P. Kjeldsen, Treatment of landfill gas with low methane content by biocover systems, Waste Manag., 2019, 84, 29–37 CrossRef CAS PubMed.
- L. C. Burrows, F. Haeri, P. Cvetic, S. Sanguinito and R. M. Enick, A Literature Review of CO2, Natural Gas, and Water-Based Fluids for Enhanced Oil Recovery in Unconventional Reservoirs, Energy Fuel., 2020, 34, 5331–5380 CrossRef CAS.
- S. N. Shoghl, R. Nazerifard and A. Naderifar, Improvement of Recovery of Gaseous Fluids Using the Replacement of Supersonic Separator instead of Joule-Thomson Valve in Dehydration/NGL Recovery Unit with Computational Fluid Dynamic Modeling, Chem. Eng. Res. Des., 2019, 148, 1–10 CrossRef.
- B. Bulfin, L. Buttsworth, A. Lidor and A. Steinfeld, High-purity nitrogen production from air by pressure swing adsorption combined with SrFeO3 redox chemical looping, Chem. Eng. J., 2021, 421, 127734 CrossRef CAS.
- A. M. Yousef, W. M. El-Maghlany, Y. A. Eldrainy and A. Attia, Upgrading biogas to biomethane and liquid CO2: a novel cryogenic process, Fuel, 2019, 251, 611–628 CrossRef CAS.
- W. Qiu, F. S. Li, S. Fu and W. J. Koros, Isomer-Tailored Carbon Molecular Sieve Membranes with High Gas Separation Performance, ChemSusChem, 2020, 1, 1–11, DOI:10.1002/cssc.202001567.
- Y. Yang, H. Wang, K. Zhang, J. P. H. Li and T. Li, Engineering Plasticization Resistant Gas Separation Membranes Using Metal-Organic Nanocapsules, Chem. Sci., 2020, 11, 1–9 RSC.
- E. Ghasemnejad-Afshar, S. Amjad-Iranagh, M. Zarif and H. Modarress, Effect of side branch on gas separation performance of triptycene based PIM membrane: a molecular simulation study, Polym. Test., 2020, 83, 106339 CrossRef CAS.
- C. Z. Sun, M. S. H. Boutilie, H. Au, P. Poesio, B. F. Bai, R. Karnik and N. G. Hadjiconstantinou, Mechanisms of Molecular Permeation through Nanoporous Graphene Membranes, Langmuir, 2014, 30, 675–682 CrossRef CAS PubMed.
- Y. Hou, Z. Xu and X. Yang, Interface-Induced Affinity Sieving in Nanoporous Graphenes for Liquid-Phase Mixtures, J. Phys. Chem. C, 2016, 120, 4053–4060 CrossRef CAS.
- S. Wang, Z. Tian, S. Dai and D. E. Jiang, Effect of Pore Density on Gas Permeation through Nanoporous Graphene Membranes, Nanoscale, 2018, 10, 14660–14666 RSC.
- M. Shan, Q. Xue, N. Jing, C. Ling, T. Zhang and Z. Yan, Influence of chemical functionalization on the CO2/N2 separation performance of porous graphene membranes, Nanoscale, 2012, 4, 5477–5482 RSC.
- H. Liu, S. Dai and D. E. Jiang, Insights into CO2/N2 separation through nanoporous graphene from molecular dynamics, Nanoscale, 2013, 5, 9984–9987 RSC.
- C. Sun, B. Wen and B. Bai, Application of nanoporous graphene membranes in natural gas processing: molecular simulations of CH4/CO2, CH4/H2S and CH4/N2 separation, Chem. Eng. Sci., 2015, 138, 616–621 CrossRef CAS.
- K. Celebi, J. Buchheim, R. M. Wyss, A. Droudian, P. Gasser, I. Shorubalko, J. I. Kye, C. Lee and H. G. Park, Ultimate permeation across atomically thin porous graphene, Science, 2014, 344, 289–292 CrossRef CAS PubMed.
- G. Lei, C. Liu, H. Xie and F. Song, Separation of the hydrogen sulfifide and methane mixture by the porous graphene membrane: effect of the charges, Chem. Phys. Lett., 2014, 599, 127–132 CrossRef CAS.
- X. Chang, L. Zhu, Q. Xue, X. Li, T. Guo, X. Li and M. Ma, Charge controlled switchable CO2/N2 separation for g-C10N9 membrane: Insights from molecular dynamics simulations, J. CO2 Util., 2018, 26, 294–301 CrossRef CAS.
- Y. Wang, Q. Yang, C. Zhong and J. Li, Theoretical investigation of gas separation in functionalized nanoporous graphene membranes, Appl. Surf. Sci., 2017, 407, 532–539 CrossRef CAS.
- S. Deng, H. Hu, G. Zhuang, X. Zhong and J. Wang, A strain-controlled C2N monolayer membrane for gas separation in PEMFC application, Appl. Surf. Sci., 2018, 441, 408–414 CrossRef CAS.
- M. Shan, Q. Xue, N. Jing, C. Ling, T. Zhang, Z. Yan and J. Zheng, Influence of chemical functionalization on the CO2/N2 separation performance of porous graphene membranes, Nanoscale, 2012, 4, 5477–5482 RSC.
- R. Lu, Z. Meng, D. Rao, Y. Wang, Q. Shi, Y. Zhang and K. Deng, A promising monolayer membrane for oxygen separation from harmful gases: nitrogen-substituted polyphenylene, Nanoscale, 2014, 6, 9960–9964 RSC.
- H. L. Du, J. Y. Li, J. Zhang, G. Su, X. Y. Li and Y. L. Zhao, Separation of Hydrogen and Nitrogen Gases with Porous Graphene Membrane, J. Phys. Chem. C, 2011, 115, 23261–23266 CrossRef CAS.
- B. Delley, An all-electron numerical method for solving the local density functional for polyatomic molecules, J. Chem. Phys., 1990, 92, 508–517 CrossRef CAS.
- B. Delley, From molecules to solids with the DMol3 approach, J. Chem. Phys., 2000, 113, 7756–7764 CrossRef CAS.
- J. P. Perdew, K. Burke and M. Ernzerhof, Generalized gradient approximation made simple, Phys. Rev. Lett., 1996, 77, 3865–3868 CrossRef CAS PubMed.
- Y. Tao, Q. Xue, Z. Liu, M. Shan, C. Ling, T. Wu and X. Li, Tunable hydrogen separation in porous graphene membrane: first-principle and molecular dynamic simulation, ACS Appl. Mater. Interfaces, 2014, 6, 8048–8058 CrossRef CAS PubMed.
- A. M. Brockway and J. Schrier, Noble gas separation using PG-ESX (X=1,2,3) nanoporous two-dimensional polymers, J. Phys. Chem. C, 2013, 117, 393–402 CrossRef CAS.
- S. Grimme, Semiempirical GGA-type density functional constructed with a long-range dispersion correction, J. Comput. Chem., 2006, 27, 1787–1799 CrossRef CAS PubMed.
- T. Zhang, Q. Z. Xue, M. X. Shan, Z. Y. Jiao, X. Y. Zhou, C. C. Ling and Z. F. Yan, Adsorption and Catalytic Activation of O2 Molecule on the Surface of Au-Doped Graphene under an External Electric Field, J. Phys. Chem. C, 2012, 116, 19918–19924 CrossRef CAS.
- Z. L. Liu, Q. Z. Xue, T. Zhang, C. C. Ling and M. X. Shan, Carbon Doping of Hexagonal Boron Nitride by using CO Molecules, J. Phys. Chem. C, 2013, 117, 9332–9339 CrossRef CAS.
- H. Sun, COMPASS: an ab initio force-field optimized for condensed-phase applications overview with details on alkane and benzene compounds, J. Phys. Chem. B, 1998, 102, 7338–7364 CrossRef CAS.
- M. Shan, Q. Xue, N. Jing, C. Ling, T. Zhang and Z. Yan, Influence of chemical functionalization on the CO2/N2 separation performance of porous graphene membranes, Nanoscale, 2012, 4, 5477–5482 RSC.
- P. Ryan, O. K. Farha, L. J. Broadbelt and R. Q. Snurr, Computational screening of metal-organic frameworks for xenon/krypton separation, AIChE J., 2011, 57, 1759–1766 CrossRef CAS.
- Z. Meng, X. Zhang, Y. Zhang, H. Gao, Y. Wang, Q. Shi, D. Rao, Y. Liu, K. Deng and R. Lu, Graphdiyne as a high-efficiency membrane for separating oxygen from harmful gases: a first-principles study, ACS Appl. Mater. Interfaces, 2016, 8, 28166–28170 CrossRef CAS PubMed.
- H. Liu, V. R. Cooper, S. Dai and D. Jiang, Windowed Carbon Nanotubes for Efficient CO2 Removal from Natural Gas, J. Phys. Chem. Lett., 2012, 3, 3343–3347 CrossRef CAS.
- S. Wang, S. Dai and D. Jiang, Continuously Tunable Pore Size for Gas Separation via a Bilayer Nanoporous Graphene Membrane, ACS Appl. Nano Mater., 2018, 2, 379–384 CrossRef.
|
This journal is © The Royal Society of Chemistry 2022 |