DOI:
10.1039/D2RA02136F
(Paper)
RSC Adv., 2022,
12, 20507-20518
Natural-product-inspired design and synthesis of thiolated coenzyme Q analogs as promising agents against Gram-positive bacterial strains: insights into structure–activity relationship, activity profile, mode of action, and molecular docking†
Received
2nd April 2022
, Accepted 5th July 2022
First published on 15th July 2022
Abstract
In an attempt to develop effective and potentially active antibacterial and/or antifungal agents, we designed, synthesized, and characterized thiolated CoQ analogs (CoQ1–8) with an extensive antimicrobial study. The antimicrobial profile of these analogs was determined using four Gram-negative bacteria, three Gram-positive bacteria, and three fungi. Because of the fact that the thiolated CoQ analogs were quite effective on all tested Gram-positive bacterial strains, including Staphylococcus aureus (ATCC® 29213) and Enterococcus faecalis (ATCC® 29212), the first two thiolated CoQ analogs emerged as potentially the most desirable ones in this series. Importantly, after the evaluation of the antibacterial and antifungal activity, we presented an initial structure–activity relationship for these CoQ analogs. In addition, the most promising thiolated CoQ analogs (CoQ1 and CoQ2) having the lowest MIC values on all tested Gram-positive bacterial strains, were further evaluated for their inhibition capacities of biofilm formation after evaluating their in vitro potential antimicrobial activity against each of 20 clinically obtained resistant strains of Gram-positive bacteria. CoQ1 and CoQ2 exhibited potential molecular interactions with S. aureus DNA gyrase in addition to excellent pharmacokinetics and lead-likeness profiles. Our findings offer important implications for a potential antimicrobial drug candidate, in particular for the treatment of infections caused by clinically resistant MRSA isolates.
1. Introduction
1,4-Quinones are important motifs in bioactive molecules that find widespread applications in cancer, microbiology, and material sciences because of two possible main reasons.1–4 Firstly, the 1,4-quinone core has been historically used as a small template for the preparation of biologically active molecules.2,4,5 Secondly, it is now well documented that the insertion of amino and/or thio group(s) into the quinone motif can lead to profound contributions to their physical, chemical, and, in particular, biological properties.6,7 Accordingly, an increasing number of aminated and/or thiolated quinones have been prepared, leading to the discovery of novel bioactive products.8–10 Mono- and/or disubstituted quinone motifs, in particular, are present in various pharmacologically related molecules, including those used as lead molecules for Leber's Hereditary Optic Neuropathy (LHON),11 anticancer,12 antibacterial,13,14 antifungal,15,16 antiHIV,17 and antimalarial therapy.18 It is worth noting that some 1,4-quinones have also found use as functional ligands for transition metals.19–22
What's more, the Coenzyme Qn (CoQn) family, also named as the ubiquinones, has occupied a unique position in the design and synthesis of novel biologically active lead molecules that exert remarkable medicinal activity.23 Besides of versatile properties, not surprisingly, ubiquinone participating in the cellular aerobic respiration process,24 plastoquinone and phylloquinone serving as electron acceptors in electron transport chains in photosynthesis, and vitamin K participating coagulation process and preventing bleeding have also a 1,4-quinone motif.25,26 To date, some important molecules such as idebenone and CoQ10 have been under clinical investigation (Fig. 1).27,28 Of these, idebenone is the only drug in the treatment of emotional disturbances associated with cerebrovascular diseases developed by Takeda Pharmaceutical Company Ltd to have been approved by the European Medicines Agency (EMA) for only under exceptional circumstances.29 In addition, CoQ is an important medicine, in particular CoQ10 which is sold in many countries as a dietary supplement to improve immunotherapy30 has a valuable efficacy on versatile heart-related diseases, hepatitis, and cancer.31
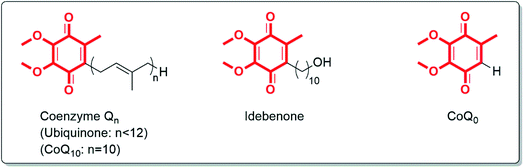 |
| Fig. 1 The most important members of the coenzyme Qn (CoQn) family. | |
In the past 10 years or so, we have prepared a different series of analogs of some important molecules containing 1,4-quinone core to explore the structure–activity relationships (SARs) around this motif as shown in Fig. 2.32 We focused on the insertion of amino (primary or secondary amines) and/or thio (aromatic or alkyl chain thiols) group(s) into the 1,4-quinone motif. On the other hand, by keeping these group(s) within 1,4-quinone moiety, we used the quinone fused with benzene named as naphthoquinone33,34 or vitamin K analogs, or attached to the dimethyl groups named as plastoquinone analogs,35–37 or fused with pyridine named as quinolinequinone analogs.8,38,39 The substituents within the amino and/or thio group(s) and the groups attached to the 1,4-quinone motif for the different series contribute significantly toward biological efficacy.8 Thus, our studies should be useful to predict which modification of the groups attached to the 1,4-quinone motif could enhance biological potency. These results motivated us to design and synthesize new analogs based on the important structure of CoQ0 containing a core methyl-1,4-quinone motif mostly similar to vitamin K. Encouraged by our previous results and literature, apart from the synthesis under mild conditions, the target analogs of CoQ0 was then evaluated for the antimicrobial evaluation to reveal the effect of the modification on their potency. Indeed, the most important members of the CoQ0 with thiols were investigated for their antibiofilm activity, potential antimicrobial activity against each of 20 clinically obtained strains of Methicillin resistant Staphylococcus aureus, and bactericidal time-kill kinetic study. The most active analogs against S. aureus were employed in a molecular docking study within thymidylate kinase TMK, a crucial factor for DNA biosynthesis40,41 and DNA gyrase enzyme which is essential for transcription and replication process of DNA molecule.42,43
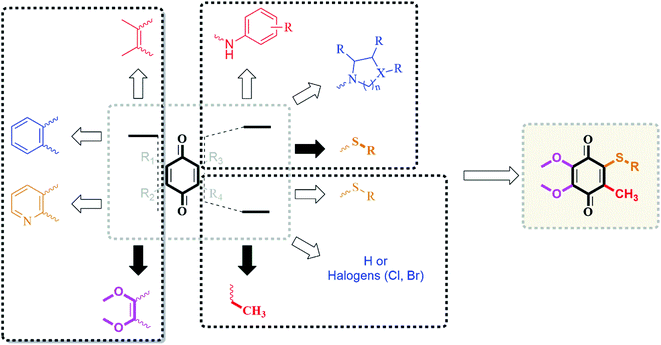 |
| Fig. 2 Design strategy by the incorporation of CoQ0 and alkyl chain thiols as the substrates based on our previous results in the literature. | |
2. Results and discussion
2.1. Library design and synthesis
The reactions between 1,4-quinones and nucleophiles such as amines, thiols, and alcohols44 addressing to the lead molecules have been extensively studied. Depending upon the substitution pattern of 1,4-quinones, in contrast to amines, generally, thiols and alcohols could generate the disubstituted products in addition to monosubstituted products. The thiolated vitamin K3 analogs containing alkyl chain thio groups were recently investigated by our group and proved to be potent, selective, and active for inhibition bacterial formation. Table 1 outlines the synthesis of the thiolated CoQ0 analogs (CoQ1–8) from the reaction of CoQ0 with a range of alkyl chain thiols in good to high yields. The commercially available CoQ0 was directly thiolated by the corresponding alkyl chain thiols in ethanol in one step (Table 1) by adopting the procedure in the literature.10,45 In connection with this, we have obtained only one product because of the presence of the methyl group instead of halogen or hydrogen atom within the 1,4-quinone moiety. All the reactions were monitored by thin-layer chromatography with the disappearance of the CoQ0, then purified via silica gel column chromatography.
Table 1 Construction of the thiolated CoQ0 analogs (CoQ1–8)
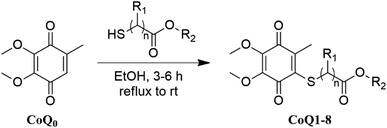
|
ID, yield (%) |
R1 |
R2 |
ID, yield (%) |
R1 |
R2 |
CoQ1 (n = 1), 75 |
H |
CH3 |
CoQ5 (n = 2), 39 |
H |
CH2CH3 |
CoQ2 (n = 1), 52 |
H |
CH2CH3 |
CoQ6 (n = 2), 23 |
H |
(CH2)3CH3 |
CoQ3 (n = 1), 62 |
CH3 |
CH2CH3 |
CoQ7 (n = 1), 79 |
H |
CH2CH(n-C2H5)(CH2)3CH3 |
CoQ4 (n = 2), 36 |
H |
CH3 |
CoQ8 (n = 2), 65 |
H |
(CH2)5CH(CH3)2 |
The structures of the thiolated CoQ0 analogs (CoQ1–8) were examined by 1H and 13C nuclear magnetic resonance (NMR), mass spectroscopy (MS), and Fourier-transform infrared spectroscopy (FTIR). High-resolution mass spectra (HRMS) was registered to confirm the characterization of the thiolated CoQ0 analogs (CoQ1–8). Additionally, the crystal structure of methyl-2-(4,5-dimethoxy-2-methyl-3,6-dioxocyclohexa-1,4-dienylthio)acetate (CoQ1) was determined by single-crystal X-ray diffraction analysis (Fig. 3). Suitable crystals for X-ray diffraction were obtained by slow evaporation of ethanol solutions of the analog (CoQ1). The ORTEP drawings of the CoQ1 was reported at 50% probability level in Fig. 3. The crystal system of the CoQ1 is monoclinic (space group P121/n1) with the unit cell parameters a = 3.9584, b = 23.942, c = 13.7731. The crystallographic and structure refinement data for the analog (CoQ1) are summarized (for details, please see the Tables S2–S6 in the ESI file†). The average values of C–S bond lengths of the analog (CoQ1) are approximately 1.78 Å. The O4–C12, O5–C9 and O1–C1 bond lengths (about 1.44 Å) between sp3 hybridized oxygen and carbon atoms are longer than the O4–C10, O5–C8, O1–C2 bond lengths (about 1.34 Å) between sp3 hybridized oxygen atoms and sp2 hybridized carbon atoms. These bonds are characteristic singlet bonds. However, the bond lengths of C11–O3, C7–O6, and O2–C2 are about 1.21 Å, confirming them as characteristic double bonds. Torsion angle, especially O3–C11–C4–S1 (9.4°), shows sulfur and oxygen atoms are not the same plane but close to each other. This allows to be formed hydrogen bonds between oxygen atom of carbonyl group and methylene protons. Besides, the long range interactions between oxygen (O5) of methoxy group and the methyl protons (C1H1) of the ester group as a hydrogen bond over the quinone ring shows in the Tables S2–S6 in the ESI† file.
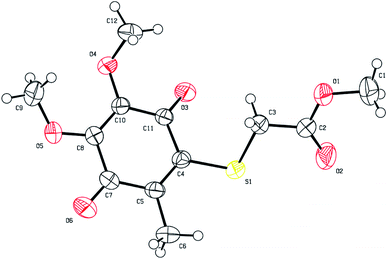 |
| Fig. 3 ORTEP drawings of CoQ1 (2150434) at 50% probability level. | |
2.2. Biological activity
2.2.1. Determination of minimum inhibitory concentrations (MIC) and structure–activity relationships (SARs) study. We investigated in vitro antibacterial and antifungal profile of the obtained thiolated CoQ0 analogs (CoQ1–8) in relation to four Gram-negative bacteria (Pseudomonas aeruginosa ATCC 27853, Escherichia coli ATCC 25922, Klebsiella pneumoniae ATCC 4352, and Proteus mirabilis ATCC 14153), three Gram-positive bacteria (Staphylococcus aureus ATCC 29213, Staphylococcus epidermidis ATCC 12228, and Enterococcus faecalis ATCC 29212), and three fungi (Candida albicans ATCC 10231, Candida parapsilosis ATCC 22019, and Candida tropicalis ATCC 750) using the commercially available reference drugs (ceftazidime, cefuroxime-Na, cefuroxime, amikacin, clotrimazole, and amphotericin B). We used the broth microdilution technique for microbiological tests to determine a minimum inhibitory concentration (MIC) values, listed in Tables 2 and 3 using the Clinical Laboratory Standards Institute (CLSI) recommendations.46,47
Table 2 The minimum inhibitory concentration (MIC) value of the thiolated CoQ analogs (CoQ1–8) for antibacterial activitya
Thiolated CoQ |
Substituents |
Gram-negative bacteria (MIC, μg mL−1) |
Gram-positive bacteria (MIC, μg mL−1) |
General formula |
ID |
R1 |
R2 |
P. aeruginosa |
E. coli |
K. pneumoniae |
P. mirabilis |
S. aureus |
S. epidermidis |
E. faecalis |
“—” means no activity. |
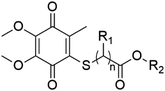 |
CoQ1 (n = 1) |
H |
–CH3 |
625 |
625 |
1250 |
1250 |
2.44 |
4.88 |
78.12 |
CoQ2 (n = 1) |
H |
–CH2CH3 |
1250 |
1250 |
1250 |
1250 |
9.76 |
9.76 |
39.06 |
CoQ3 (n = 1) |
CH3 |
–CH2CH3 |
— |
— |
— |
— |
19.53 |
— |
78.12 |
CoQ4 (n = 2) |
H |
–CH3 |
1250 |
1250 |
— |
1250 |
9.76 |
9.76 |
156.25 |
CoQ5 (n = 2) |
H |
–CH2CH3 |
— |
— |
— |
— |
9.76 |
— |
39.06 |
CoQ6 (n = 2) |
H |
–(CH2)3CH3 |
— |
— |
— |
— |
156.25 |
— |
312.50 |
CoQ7 (n = 1) |
H |
–CH2CH(n-C2H5)(CH2)3CH3 |
— |
— |
— |
— |
156.25 |
— |
— |
CoQ8 (n = 2) |
H |
–(CH2)5CH(CH3)2 |
— |
— |
— |
— |
1250 |
— |
— |
Ceftazidime |
2.44 |
|
|
|
|
|
|
Cefuroxime-Na |
|
4.88 |
4.88 |
2.44 |
1.22 |
|
|
Cefuroxime |
|
|
|
|
|
9.76 |
|
Amikacin |
|
|
|
|
|
|
128.00 |
Table 3 The minimum inhibitory concentration (MIC) value of the thiolated CoQ analogs (CoQ1–8) for antifungal activitya
Thiolated CoQ |
Substituents |
Fungi (MIC, μg mL−1) |
General formula |
ID |
R1 |
R2 |
C. albicans |
C. parapsilosis |
C. tropicalis |
“—” means no activity. |
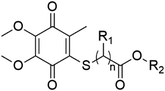 |
CoQ1 (n = 1) |
H |
–CH3 |
625 |
156.25 |
312.50 |
CoQ2 (n = 1) |
H |
–CH2CH3 |
1250 |
312.50 |
312.50 |
CoQ3 (n = 1) |
CH3 |
–CH2CH3 |
— |
78.12 |
— |
CoQ4 (n = 2) |
H |
–CH3 |
1250 |
312.50 |
312.50 |
CoQ5 (n = 2) |
H |
–CH2CH3 |
— |
78.12 |
312.50 |
CoQ6 (n = 2) |
H |
–(CH2)3CH3 |
— |
78.12 |
— |
CoQ7 (n = 1) |
H |
–CH2CH(n-C2H5)(CH2)3CH3 |
— |
156.25 |
— |
CoQ8 (n = 2) |
H |
–(CH2)5CH(CH3)2 |
— |
— |
— |
Clotrimazole |
4.9 |
|
|
Amphotericin B |
|
0.5 |
1 |
Some analogs (CoQ3 and CoQ5–8) having alkyl chain thio groups were totally inactive against all Gram-negative bacterial strains. Surprisingly, CoQ1, CoQ2, and CoQ4 showed weak antibacterial potency against all Gram-negative bacterial strains. On the other hand, to our delight, it can be seen from the data in Table 2 that the thiolated CoQ analog (CoQ1) was the most potent active against S. aureus and S. epidermidis among all obtained analogs, while CoQ2 and CoQ5 were the most active analogs against E. faecalis having MIC value 39.06 μg mL−1. It is noteworthy to mention that three thiolated CoQ (CoQ2, CoQ4, and CoQ5) were the other attractive analogs on S. aureus with MIC value of 9.76 μg mL−1. These analogs were found to be the stronger antibacterial lead molecules against some Gram-positive bacterial strains as compared to reference drugs. Excitingly, when we examined the antibacterial profile of the thiolated CoQ analogs against S. epidermidis strains, apart from CoQ1, we observed that two other analogs (CoQ2 and CoQ4) had the same potent activity with the reference drug Cefuroxime, MIC = 9.76 μg mL−1. Of all examined for antibacterial activity against E. faecalis strains, CoQ1–5 displayed the remarkable activity (MIC range = 39.06–156.25 μg mL−1). In particular, CoQ2 and CoQ5 showed the highest activity as the most potent analogs against E. faecalis, whereas CoQ1 and CoQ3 had 1.6-fold more potent analogs when compared reference standard Amikacin (MIC = 78.12 μg mL−1). Notably, CoQ4 exhibited good antibacterial activity against E. faecalis with MIC value (156.25 μg mL−1).
Concerning the three fungal strains, less activity was observed against C. albicans, C. parapsilosis, and C. tropicalis, and these molecules displayed antifungal activity with MIC values ranging between 78.12 and 1250 μg mL−1. However these moderate activity could be achieved by penetrating into the fungal cell via the cellular membrane by passive diffusion with the greater lipophilic characters of these molecules.48 Among the tested thiolated CoQ analogs, two CoQ analogs (CoQ1 and CoQ2) showed strong antibacterial potency. The most promising thiolated CoQ analogs, CoQ1 and CoQ2, with the highest antibacterial efficacy on Gram-positive bacterial strains, were chosen for further evaluation in order to establish their mode of action. Thus, we mainly focused on these two CoQ analogs for antimicrobial potency against clinically obtained Methicillin-resistant strains of Staphylococcus aureus. The in vitro activities of the CoQ1 and CoQ2 against 20 clinically isolates of MRSA are summarized in Fig. 4. The in vitro activity of selected molecules (CoQ1 and CoQ2) had strong antimicrobial efficiency with the MIC50 value 19.53 μg mL−1 on the studied strains. With this promising activity results, we focused further analysis of these potent antimicrobial effective molecules as drug candidate molecules.
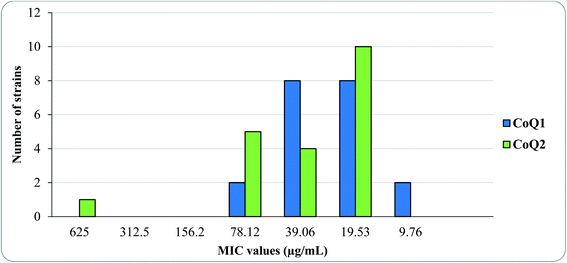 |
| Fig. 4 The MIC distribution of CoQ1 and CoQ2 against 20 clinically obtained Meticillin-Resistant Staphylococcus aureus isolates. | |
In order to understand the effect of alkyl chain moiety of the thiol on the biological profile of the thiolated CoQ analogs, we analyzed the structural features with their MIC values of the CoQ analogs by manipulating the backbone group attached to the oxygen atom while keeping the methylene bridge(s) or methine bridge between the sulfur and carbonyl group of the substrates. Apparently, there is a simple correlation between the alkyl chain of the thiol and biological activity. The presented data clearly showed that either the elongation of the methylene bridge or the insertion of the long-chain alkyl group attached to the oxygen atom starting from methyl, ethyl, and butyl group into the alkyl chain thiol decreased the biological potency, in particular against S. aureus and S. epidermidis. The efficacy against S. aureus and S. epidermidis decreased with the insertion of additional methyl group instead of hydrogen atom in methylene bridge in the thiolated CoQ analogs (CoQ3). In general, we could understand that a manipulation of the methylene bridge (elongation of the methylene bridge or insertion of methyl group) decreases the activity. Besides, changing the methyl to ethyl or butyl group decreased the potency by the elongation of the alkyl chain attached to the oxygen atom.
2.2.2. Time-kill kinetic study. Two most active compounds CoQ1 and CoQ2 were selected from the library after analyzing in vitro antimicrobial activity for further investigation of the mode of the action. Time-kill studies for CoQ1 and CoQ2 were performed on one clinically obtained Methicillin resistant Staphylococcus aureus isolate and the results are given in Fig. 5.
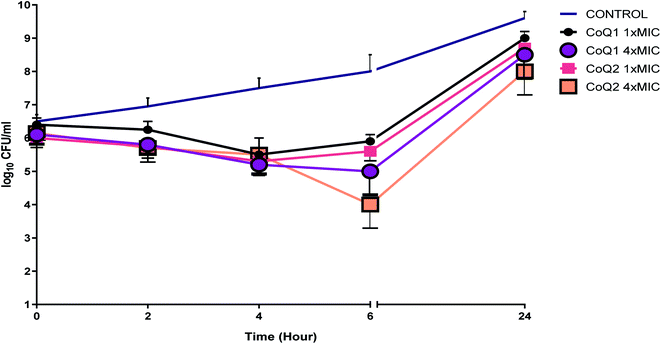 |
| Fig. 5 Time-kill determinations for clinically resistant MRSA isolate after treatment with CoQ1 and CoQ2 at 1× and 4× MIC, respectively. The x-axis represents the killing time, and the y-axis represents the logarithmic MRSA survival. | |
The results of the TKC studies did not show bactericidal activity (with a 3log10 kill determined) for the studied strain at 1× and 4× MIC concentrations within 24 h (Fig. 5). Even if the tested molecules decreased the viable cell count at 6 h for the tested strain, within the 24 h, bacterial regrowth was seen when the tested molecules used alone. In the era of antimicrobial resistance, it may be reasonable to examine the effects of these molecules which have low MIC values but results bacterial regrowth at 24 h when used alone, to show synergistic activity in combination with antibiotics frequently used in clinics.
2.2.3. Evaluation of the in vitro antibiofilm activity. Biofilms are described as a cluster multicellular surface-attached communities of bacteria embedded in self-produced extracellular matrix and they are commonly attached to living or nonliving surfaces and may be widespread in nature, hospital settings, and industry.49,50 The biofilm lifestyle allows the bacteria to withstand hostile environmental conditions like starvation, desiccation and makes them capable to cause a broad range of chronic diseases. Hence, it is considered as a major cause of persistent nosocomial infections. Around 50% of the nosocomial infections are known biofilm-related.51Biofilm development process has 4 stages: (1) attachment of bacterial cells to a suitable biotic/abiotic surface, (2) development of biofilm structure, (3) maturation of biofilm, and (4) dispersion. The investigation of the efficient antibiofilm molecules is targets of these stages especially the first two stages which are highly important for the biofilm development.50,52 For this reason, we purposed to determine the antibiofilm effects of CoQ1 and CoQ2 against the first two stages of clinically obtained MRSA biofilms. When the 1/10× MICs of tested molecules were examined for 1, 2, or 4 hours at 37 °C for MRSA's adherence to the wells of tissue culture microtiter plates, the tested agents inhibited biofilm attachment processes at least 50% for CoQ2 at 4 h. In general, the inhibition rates of adhesion showed a time-dependent effect for MRSA (Fig. 6). When we evaluated the % biofilm formation of the studied strains, the rates of biofilm formation inhibition were dependent on concentration; the highest inhibition rates were shown at 1× MICs for the tested molecule, except for CoQ2 (Fig. 6). For CoQ2 molecule, 1× MIC and 1/10× MIC concentrations showed the same moderate activity against the tested MRSA's biofilms formation.
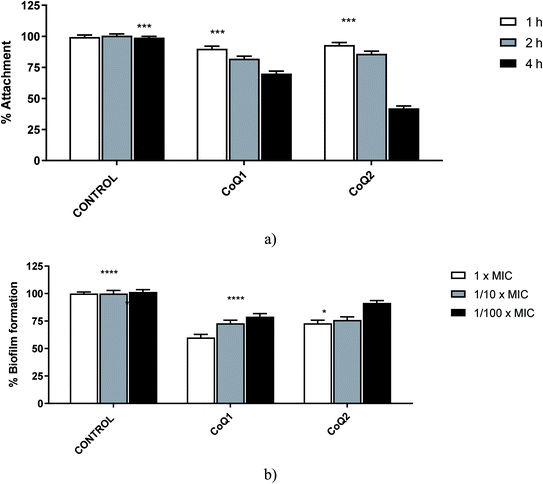 |
| Fig. 6 Inhibition of MRSA. (a) Surface attachment to the wells contained 1/10× MIC of molecules and an inoculum of 1 × 106 CFU/200 μL, incubated for 1, 2, or 4 h at 37 °C for MRSA. (b) Biofilm formation in each well contained 1×, 1/10×, or 1/100× MIC of molecules and an inoculum of 1 × 106 to 1 × 107 CFU/200 μL, incubated for 24 h at 37 °C for MRSA. Control bars indicate microorganisms without molecules accepted as 100%. Six wells were used for the tested molecule. Each experiment is representative of two independent tests. All differences between the control and molecules treated biofilms were statistically significant (****p < 0.005). | |
2.2.4. Molecular docking study. In the field of drug discovery and design, molecular docking calculations have become one of the most important tools to explore ligand/protein interactions. In a trial to elucidate their mechanism of action, we performed a docking study of our lead compounds with S. aureus TMK41 and DNA gyrase.53 The latter is classified as topoisomerase II enzyme that play crucial role in the transcription and replication process of DNA molecule. It has a pivotal role in all types of bacteria except higher eukaryotes. This makes DNA gyrase an attractive target for designing new antimicrobial drugs.43,54 The interactions of CoQ1 and CoQ2 with TMK revealed a weak affinity, hence it is unlikely for TMK to be their molecular target (data not shown). However, their interactions with DNA gyrase showed interesting results that are in accordance with the in vitro output. CoQ1 demonstrated strong molecular interactions with key amino acid residues. Its side chain has been implicated in two strong H-bonds with Arg144 and its quinone has two H–Pi bonds with Ile86. The main difference from the co-crystallized native ligand is only missing an interaction with Asp48. However, CoQ2 quinone scaffold has formed one H-bond with Thr173 and one H–Pi bond with Ile86. The terminal hydrophobic alkyl side chain extends between unfavorable polar Arg144 and Arg84 residues, that is why longer alkyl chain abolishes the activity (Fig. 7).
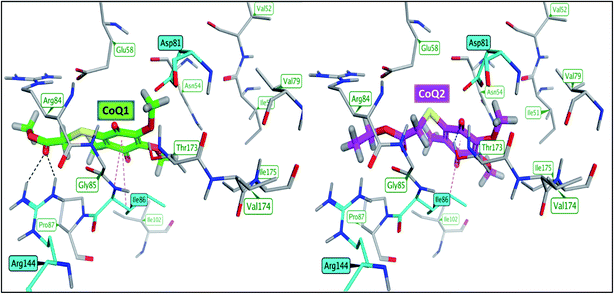 |
| Fig. 7 Binding mode of CoQ1 (left) and CoQ2 (right) into the ligand binding site of S.aureus DNA gyrase (PDB: 3G75). | |
2.2.5. In silico drug-likeness and ADME analysis of CoQ1 and CoQ2. Drug-likeness and ADME prediction of new chemical entities is significant in modern medicinal chemistry. SwissADME server, a free tool for drug likeness prediction, was applied for calculating several molecular and structural features of CoQ1 and CoQ2. Both compounds can be easily transported in the body due to their small molecular weight (286 g mol−1 for CoQ1 and 300 g mol−1 for CoQ2). This small molecular weight makes them ideal lead compounds that can be modified to enhance their affinity towards their target. Their octanol–water partition coefficient (log
P), which indicates lipophilicity, is in the acceptable range (−0.4–5.6) Table 4. They have the same total polar surface area TPSA 104.20 Å2 indicating potential good bioavailability. Furthermore, the number of hydrogen bond acceptors (HBA < 10) and number of hydrogen bond donors (HBD < 5), are in the acceptable ranges. SwissADME calculations revealed that the titled compounds possess lead-likeness properties55 and they obey all of Lipinski,56 Ghose,57 Veber,58 Egan,59 and Muegge60 rules for drug-likeness without any violations (Table 5).
Table 4 Physico-chemical properties and drug-likeness prediction
Property/rule |
CoQ1 |
CoQ2 |
MW |
286 |
300 |
Log P |
2.17 |
2.24 |
TPSA (Å2) |
104.20 |
104.20 |
HBA |
6 |
6 |
HBD |
0 |
0 |
Lipiniski |
Yes, 0 violation |
Yes, 0 violation |
Ghose |
Yes |
Yes |
Veber |
Yes |
Yes |
Egan |
Yes |
Yes |
Muegge |
Yes |
Yes |
Leadlikeness |
Yes |
Yes |
Table 5 ADME prediction
Property |
CoQ1 |
CoQ2 |
BBB permeability |
No |
No |
GI absorption |
High |
High |
Log S |
−2.11 |
−2.36 |
Solubility |
Soluble |
Soluble |
Bioavilability score |
0.56 |
0.56 |
CYP1A2, CYP2C9, CYP2D6, CYP3A4 |
No |
No |
ADME properties of CoQ1 and CoQ2 showed promising profiles as well. In SwissADME, they use a BOILED-Egg model that showed that both compounds can't pass through blood–brain barrier (BBB) which help avoid side effects on central nervous system (CNS). They also likely to have a good gastrointestinal (GI) absorption which is attributed to their ability to passively absorbed by the GIT. They have a quite good solubility61 and bioavailability score.62 Furthermore, they have no interference with metabolic enzymes cytochrome P450 isoforms CYP1A2, CYP2C9, CYP2D6, and CYP3A4.
3. Conclusions
We have successfully demonstrated that the thiolated CoQ analogs show promising structures as a template for the development of antibiotics on a sustainable basis due to the fact that further efforts for optimization are required before these thiolated CoQ analogs can be turned into valuable lead molecules for bacterial infections. In conclusion, we designed and synthesized a series of the thiolated CoQ analogs (CoQ1–8) for their antimicrobial activity. In addition to design and synthesis, we tested in vitro antimicrobial activity assays on seven different bacterial strains and three different fungal strains by using serial dilution method. The obtained results indicated that the antibacterial activity of the CoQ analogs increased by the insertion of alkyl chain thiols into the CoQ core. Extensive antibacterial tests for the most promising molecules (CoQ1 and CoQ2) on bacterial strains, S. aureus, S. epidermidis, and E. faecalis resulted in new potential analogs against bacterial infections. Furthermore, two thiolated CoQ analogs (CoQ1 and CoQ2) were also used for understanding the mode of action by evaluating the time-kill kinetic study. Also, we carried out another study on two analogs, active on S. aureus, via determining its biofilm inhibition capacities. CoQ1 and CoQ2 demonstrated strong molecular interactions with DNA gyrase with optimal lead like pharmacokinetic profile. CoQ1 and CoQ2 demonstrated strong molecular interactions with DNA gyrase with optimal lead like pharmacokinetic profile.
As far as we know, our study is the first record to evaluate the antibiofilm and bactericidal activities of the thiolated CoQ analogs against clinically resistant species. In line with this view, in our study, CoQ1 and CoQ2 showed potent antimicrobial activity against the clinically obtained MRSA strains with the 19.53 μg mL−1 MIC50. Although, in accordance with the time-kill curve studies results, these molecules showed bacterial regrowth at 1× and 4× MIC concentration used within 24 h against Methicillin-resistant S. aureus isolate, these molecules achieved decreased viable cell count at 6 h. So, to achieve synergistic activity, it would be possible to use these molecules in combination with conventional antibiotics, to reduce the antibiotic toxicity and reducing to antimicrobial resistance. Moreover, the results obtained from antibiofilm activity studies reveal that these molecules can be considered for future studies on dispersal of MRSA biofilms. In conclusion, the potential antibacterial activity of the thiolated CoQ analogs (CoQ1–8) afford them a potential to be developed as novel antibacterial drugs.
4. Experimental
4.1. Chemicals and apparatus
Reagents and solvents were of analytical grade and were purchased from commercial suppliers with a minimum purity of 95%), and used as received. Melting points (mp) were determined on electrical melting point (Büchi B-540) and are uncorrected. The Fourier transform infrared spectra were recorded on a Alpha T FTIR spectrometer equipped with single reflection diamond ATR module. 1H NMR and 13C NMR spectra were acquired on a Bruker for the characterization of the purified analogs. The 1H NMR spectra data is expressed in the form: chemical shifts in units of parts per million (ppm) in CDCl3 and coupling constants (J) are in hertz (Hz). Mass spectra were recorded on a BRUKER Microflex LT by MALDI (Matrix Assisted Laser Desorption Ionization)-TOF technique via addition of 1,8,9-anthracenetriol (DIT, dithranol) as matrix. High-resolution mass spectra electrospray ionization (HRMS-ESI) were obtained on a Waters SYNAPT G1 MS by dissolving analogs (2–3 mg) in acetonitrile. Thin layer chromatography (TLC) was performed with silica gel coated aluminum sheets from Merck KGaA. TLC visualization was carried out by using UV light (254 nm). All synthesized thiolated CoQ analogs were purified by column chromatography with a silica gel 60 (63–200 μm particle sized) with appropriate solvent system as eluent.
4.2. X-ray diffraction analysis
Data for the single crystal compounds were obtained with Bruker APEX II QUAZAR three-circle diffractometer. Indexing was performed using APEX2.63 Data integration and reduction were carried out with SAINT.64 Absorption corrections were performed by multi-scan method implemented in SADABS.65 The Bruker SHELXTL66 software package was used for structures solution and structures refinement. Crystal structure validations and geometrical calculations were performed using the Platon software.67 Mercury software68 was used for visualization of the .cif files. The crystallographic and structure refinement data, the selected bond lengths, bond angles, torsion angles, hydrogen bond distances, and angles are given in the Tables S2–S6 in the ESI file†). The crystallographic data have been deposited at the Cambridge Crystallographic Data Centre, and CCDC reference numbers is 2150434 for CoQ1.†
4.3. General procedure for the synthesis of coenzyme Q (CoQ) analogs
To a stirred solution of CoQ (100 mg, 1 eq.) in ethanol (20 mL) was added corresponding thiol (1 eq.) dropwise at room temperature. The reaction mixture was then refluxed until consumption of starting material. The reaction mixture was cooled to ambient temperature. After the reaction mixture was concentrated under reduced pressure, the residue was dissolved with CH2Cl2 (50 mL), and the solution was washed sequentially with water (3 × 30 mL). The organic layer was dried over CaCl2, filtered, and concentrated under reduced pressure, and the residue was purified by means of column chromatography on silica gel to afford desired products (CoQ analogs).
4.3.1. Methyl-2-(4,5-dimethoxy-2-methyl-3,6-dioxocyclohexa-1,4-dienylthio)acetate (CoQ1). The general procedure was followed using methyl thioglycolate (60 mg, 1 eq.) and CoQ. Purification by column chromatography on silica gel (PET/EtAc, v/v 5
:
1) yielded CoQ1 (75%) as a yellow viscose oil. FTIR (ATR) υ (cm−1): 2947, 2856 (CHaliphatic), 1738, 1661, 1631 (>C
O), 1582, 1436, 1376, 1302, 1257, 1194, 1172, 1128, 1104; 1H NMR (500 MHz, CDCl3) δ (ppm): 3.99 (s, 3H, OCH3), 3.95 (s, 3H, OCH3), 3.83 (s, 2H, SCH2), 3.69 (s, 3H, OCH3), 2.17 (s, 3H, CH3); 13C NMR (125 MHz, CDCl3) δ (ppm): 181.6, 180.1, 169.6 (>C
O), 145.0, 144.8, 143.1, 139.7 (Cq), 61.3, 61.1, 52.6 (OCH3), 34.7 (SCH2), 14.3 (CH3); Anal. calcd for C12H14O6S: C, 50.34; H, 4.93; found: C, 49.92; H, 5.02; MS (MALDI TOF) m/z: 288 [M + 2H]+, Anal. calcd for C12H14O6S (286.05); HRMS (TOF MS ES+) m/z calcd for C12H15O6S [M + H]+: 287.0589; found: 287.0591; calcd for C12H14O6SNa [M + Na]+: 309.0409; found: 309.0414.
4.3.2. Ethyl-2-(4,5-dimethoxy-2-methyl-3,6-dioxocyclohexa-1,4-dienylthio)acetate (CoQ2). The general procedure was followed using ethyl thioglycolate (66 mg, 1 eq.) and CoQ. Purification by column chromatography on silica gel (PET/EtAc, v/v 5
:
1) yielded CoQ2 (52%) as a yellow viscose oil. FTIR (ATR) υ (cm−1): 2993, 2959, 2936, 2856 (CHaliphatic), 1742, 1664, 1649, 1628 (>C
O), 1582, 1296, 1259, 1175, 1129, 1106, 1025, 1003; 1H NMR (500 MHz, CDCl3) δ (ppm): 4.15 (q, J = 7.1 Hz, 2H, OCH2), 4.01 (s, 3H, OCH3), 3.97 (s, 3H, OCH3), 3.83 (s, 2H, SCH2), 2.19 (s, 3H, CH3), 1.24 (t, J = 7.1 Hz, 3H, OCH2CH3); 13C NMR (125 MHz, CDCl3) δ (ppm): 181.6, 180.1, 169.1 (>C
O), 145.0, 144.8, 143.1, 139.9 (Cq), 61.6 (OCH2), 61.3, 61.2 (OCH3), 34.9 (SCH2), 14.3, 14.1 (CH3); Anal. calcd for C13H16O6S: C, 51.99; H, 5.37; found: C, 51.85; H, 5.12; HRMS (TOF MS ES+) m/z calcd for C13H17O6S [M + H]+: 301.0746; found: 301.0746; calcd for C13H16O6SNa [M + Na]+: 323.0565; found: 323.0575.
4.3.3. Ethyl-2-(4,5-dimethoxy-2-methyl-3,6-dioxocyclohexa-1,4-dienylthio)propanoate (CoQ3). The general procedure was followed using ethyl-2-mercaptopropanoate (73 mg, 1 eq.) and CoQ. Purification by column chromatography on silica gel (PET/EtAc, v/v 5
:
1) yielded CoQ3 (62%) as a yellow viscose oil. FTIR (ATR) υ (cm−1): 2983, 2944, 2848 (CHaliphatic), 1733, 1657, 1634 (>C
O), 1584, 1451, 1376, 1300, 1247, 1198, 1130, 1093; 1H NMR (500 MHz, CDCl3) δ (ppm): 4.35 (q, J = 7.1 Hz, 1H, SCHCH3), 4.25–4.06 (m, 2H, OCH2), 4.02 (s, 3H, OCH3), 4.00 (s, 3H, OCH3), 2.19 (s, 3H, CH3), 1.52 (d, J = 7.1 Hz, 3H, CHCH3), 1.21 (t, J = 7.1 Hz, 3H, OCH2CH3); 13C NMR (125 MHz, CDCl3) δ (ppm): 182.0, 180.0, 171.8 (>C
O), 145.2, 144.9, 144.4, 140.3 (Cq), 61.4 (OCH2), 61.3, 61.1 (OCH3), 43.3 (SCH), 16.7, 14.6, 14.1 (CH3); Anal. calcd for C14H18O6S: C, 53.49; H, 5.77; found: C, 53.22; H, 5.56; HRMS (TOF MS ES+) m/z calcd for C14H19O6S [M + H]+: 315.0902; found: 315.0901.
4.3.4. Methyl-3-(4,5-dimethoxy-2-methyl-3,6-dioxocyclohexa-1,4-dienylthio)propanoate (CoQ4). The general procedure was followed using methyl 3-mercaptopropanoate (66 mg, 1 eq.) and CoQ. Purification by column chromatography on silica gel (PET/EtAc, v/v 5
:
1) yielded CoQ4 (36%) as a yellow viscose oil. FTIR (ATR) υ (cm−1): 2962, 2919, 2848 (CHaliphatic), 1738, 1659, 1631 (>C
O), 1579, 1430, 1354, 1302, 1242, 1187, 1128, 1097; 1H NMR (500 MHz, CDCl3) δ (ppm): 4.02 (s, 3H, OCH3), 4.01 (s, 3H, OCH3), 3.69 (s, 3H, OCH3), 3.32 (t, J = 7.0 Hz, 2H, SCH2), 2.68 (t, J = 7.0 Hz, 2H, CH2(C
O)), 2.18 (s, 3H, CH3); 13C NMR (125 MHz, CDCl3) δ (ppm): 181.8, 180.3, 171.9 (>C
O), 145.1, 144.8, 143.6, 140.8 (Cq), 61.4, 61.2, 51.9 (OCH3), 35.5 (SCH2), 29.2 (CH2(C
O)), 14.6 (CH3); Anal. calcd for C13H16O6S: C, 51.99; H, 5.37; found: C, 51.73; H, 5.39; HRMS (TOF MS ES+) m/z calcd for C13H17O6S [M + H]+: 301.0746; found: 301.0745.
4.3.5. Ethyl-3-(4,5-dimethoxy-2-methyl-3,6-dioxocyclohexa-1,4-dienylthio)propanoate (CoQ5). The general procedure was followed using ethyl 3-mercaptopropanoate (74 mg, 1 eq.) and CoQ. Purification by column chromatography on silica gel (PET/EtAc, v/v 5
:
1) yielded CoQ5 (39%) as a yellow viscose oil. FTIR (ATR) υ (cm−1): 2985, 2947, 2848 (CHaliphatic), 1730, 1655, 1634 (>C
O), 1580, 1452, 1370, 1298, 1247, 1191, 1131, 1097; 1H NMR (500 MHz, CDCl3) δ (ppm): 4.06 (q, J = 7.1 Hz, 2H, OCH2), 3.94 (s, 3H, OCH3), 3.93 (s, 3H, OCH3), 3.23 (t, J = 7.0 Hz, 2H, SCH2), 2.57 (t, J = 7.0 Hz, 2H, CH2(C
O)), 2.10 (s, 3H, CH3), 1.18 (t, J = 7.1 Hz, 3H, OCH2CH3); 13C NMR (125 MHz, CDCl3) δ (ppm): 181.8, 180.2, 171.4 (>C
O), 145.1, 144.8, 143.5, 140.9 (Cq), 61.3 (OCH2), 61.2, 60.8 (OCH3), 35.7 (SCH2), 29.2 (SCH2(C
O)), 14.6, 14.2 (CH3); Anal. calcd. for C14H18O6S: C, 53.49; H, 5.77; found: C, 53.77; H, 5.89; HRMS (TOF MS ES+) m/z calcd for C14H19O6S [M + H]+: 315.0902; found: 315.0904.
4.3.6. Butyl-3-(4,5-dimethoxy-2-methyl-3,6-dioxocyclohexa-1,4-dienylthio)propanoate (CoQ6). The general procedure was followed using butyl 3-mercaptopropanoate (89 mg, 1 eq.) and CoQ. Purification by column chromatography on silica gel (PET/EtAc, v/v 5
:
1) yielded CoQ6 (23%) as a yellow viscose oil. FTIR (ATR) υ (cm−1): 2958, 2937, 2874 (CHaliphatic), 1732, 1697, 1657 (>C
O), 1583, 1455, 1298, 1248, 1197, 1134, 1101, 1066; 1H NMR (500 MHz, CDCl3) δ (ppm): 4.01 (t, J = 6.7 Hz, 2H, OCH2), 3.94 (s, 3H, OCH3), 3.93 (s, 3H, OCH3), 3.23 (t, J = 6.9 Hz, 2H, SCH2), 2.58 (t, J = 6.9 Hz, 2H, CH2(C
O)), 2.10 (s, 3H, CH3), 1.57–1.47 (m, 2H, CH2), 1.36–1.22 (m, 2H, CH2), 0.86 (t, J = 7.4 Hz, 3H, CH3); 13C NMR (125 MHz, CDCl3) δ (ppm): 181.8, 180.2, 171.5 (>C
O), 145.1, 144.8, 143.5, 140.9 (Cq), 64.7 (OCH2), 61.3, 61.2 (OCH3), 35.7 (SCH2), 30.6 (SCH2(C
O)), 29.2, 19.1 (CH2), 14.5, 13.7 (CH3); Anal. calcd for C16H22O6S: C, 56.12; H, 6.48; found: C, 55.76; H, 6.33; HRMS (TOF MS ES+) m/z calcd for C16H23O6S [M + H]+: 343.1215; found: 343.1215.
4.3.7. 2-Ethylhexyl-2-(4,5-dimethoxy-2-methyl-3,6-dioxocyclohexa-1,4-dienylthio)acetate (CoQ7). The general procedure was followed using 2-ethylhexyl thioglycolate (112 mg, 1 eq.) and CoQ. Purification by column chromatography on silica gel (PET/EtAc, v/v 5
:
1) yielded CoQ7 (79%) as a yellow viscose oil. FTIR (ATR) υ (cm−1): 2931, 2860 (CHaliphatic), 1733, 1658, 1634 (>C
O), 1582, 1457, 1374, 1294, 1249, 1198, 1132, 1099; 1H NMR (500 MHz, CDCl3) δ (ppm): 4.10–3.93 (m, 8H, OCH2 + OCH3), 3.87 (s, 2H, SCH2), 2.19 (s, 3H, CH3), 1.63–1.46 (m, 1H, CH), 1.41–1.13 (m, 8H, CH2), 0.96–0.82 (m, 6H, CH3); 13C NMR (125 MHz, CDCl3) δ (ppm): 181.6, 180.1, 169.4 (>C
O), 145.0, 144.8, 142.9, 139.9 (Cq), 68.0 (OCH2), 61.3, 61.1 (OCH3), 38.7 (SCH2), 34.9 (CH), 30.2, 28.9, 23.6, 22.9 (CH2), 14.3, 14.0, 10.9 (CH3); Anal. calcd for C19H28O6S: C, 59.35; H, 7.34; found: C, 59.65; H, 7.39; HRMS (TOF MS ES+) m/z calcd for C19H29O6S [M + H]+: 385.1685; found: 385.1685.
4.3.8. 6-Methylheptyl-3-(4,5-dimethoxy-2-methyl-3,6-dioxocyclohexa-1,4-dienylthio)propanoate (CoQ8). The general procedure was followed using isooctyl-3-mercaptopropionate (120 mg, 1 eq.) and CoQ. Purification by column chromatography on silica gel (PET/EtAc, v/v 5
:
1) yielded CoQ8 (65%) as a yellow viscose oil. FTIR (ATR) υ (cm−1): 2956, 2926, 2874 (CHaliphatic), 1733, 1658, 1634 (>C
O), 1579, 1455, 1365, 1296, 1248, 1197, 1132, 1097; 1H NMR (500 MHz, CDCl3) δ (ppm): 4.12–4.08 (m, 2H, OCH2), 4.07–3.92 (m, 6H, OCH3), 3.34–3.30 (m, 2H, SCH2), 2.8–2.64 (m, 2H, SCH2CH2), 2.17 (s, 3H, CH3), 1.78–1.48 (m, 4H, CH2), 1.45–1.20 (m, 4H, CH2), 1.20–1.10 (m, 1H, CH), 0.95–0.80 (m, 6H, CH3); 13C NMR (125 MHz, CDCl3) δ (ppm): 181.7, 180.2, 171.5 (>C
O), 145.1, 144.8, 143.4, 140.9 (Cq), 63.4 (OCH2), 61.3, 61.2 (OCH3), 36.6 (SCH2), 29.2 (SCH2(C
O)), 27.4, 25.1, 23.3, 22.9, 22.2 (CH2 and CH), 19.7, 14.6, 14.1 (CH3); Anal. calcd for C20H30O6S: C, 60.28; H, 7.59; found: C, 60.54; H, 7.29; MS (MALDI TOF) m/z: 398 [M–H]+, Anal. calcd for C20H30O6S (398.51); HRMS (TOF MS ES+) m/z calcd for C20H31O6S [M + H]+: 399.1841; found: 399.1840.
4.4. Biological evaluation
4.4.1. MIC evaluation. MICs of the molecules were examined by the broth microdilution technique approved by Clinical and Laboratory Institute (CLSI).46,47 The inoculum of the tested four Gram negative (Pseudomonas aeruginosa ATCC 27853, Escherichia coli ATCC 25922, Klebsiella pneumoniae ATCC 4352, Proteus mirabilis ATCC 14153), three Gram positive (Staphylococcus aureus ATCC 29213, Staphylococcus epidermidis ATCC 12228, Enterococcus faecalis ATCC 29212), and three yeast (Candida albicans ATCC 10231, Candida parapsilosis ATCC 22019, Candida tropicalis ATCC 750) were prepared by CLSI recommendations. The stock solutions of the tested molecules were prepared in DMSO. Serial two fold dilutions ranging from 1250 to 0.06 μg mL−1 were prepared in Mueller Hinton Broth for the tested bacteria and RPMI-1640 medium for the yeast, respectively.According to the antimicrobial activity results, we aimed to identify in vitro activities of the CoQ1 and CoQ2 against clinically obtained strains by the broth microdilution dilution technique as described by the CLSI recommendations.46,47 For this assay, 20 nonduplicate, nosocomially acquired Methicillin-resistant Staphylococcus aureus isolated from blood specimens between April and September 2017 were obtained from the Department of Infectious Diseases and Clinical Microbiology, Faculty of Medicine, Istanbul Medipol University. All strains were identified using API STAPH (bioM'erieux). Then, all the tested S. aureus isolates were chosen by using oxacillin susceptibility to determine the methicillin resistant isolates, approved by CLSI (MIC ≥ 4 μg mL−1).46 The MIC was defined as the lowest concentration of tested extracts giving complete inhibition of visible growth. Experiments were performed in triplicate.
4.4.2. Determination of time-kill curves. The bactericidal activity of selected molecules (CoQ1 and CoQ2) was tested by the time-killing curve (TKC) method at one and four times the MIC against one (1) MRSA clinical strain. Molecules-free controls were included for the tested strain. Inocula were quantified spectrophotometrically and added to the flasks to yield a final concentration of 1 × 106 CFU mL−1. The test tubes containing MHB with and without (growth control) molecules in a final volume of 10 mL were incubated in a 37 °C calibrated shaking water bath, and viable counts were determined at 0, 2, 4, 6, and 24 h intervals after inoculation, by subculturing 0.1 mL serial dilutions onto TSA plates. All tests were performed in duplicate. The lower limit of detection for time-kill assay was 1log10 CFU per mL. Bactericidal activity was defined as a ≥3log10 CFU per mL decrease from the initial inoculum.
4.4.3. Determination of the antibiofilm activities. Biofilm attachment and inhibition of biofilm formation assays were performed as previously described method with some modifications.37 For biofilm attachment, an overnight culture of strong, biofilm-producing clinically MRSA isolate was diluted 1/50 to obtain 1 × 106 to 1 × 107 CFU/200 mL for bacteria in TSB supplement with 1% glucose. Then the strain was added to each well of 96-well tissue culture microtiter plates with 1/10× MIC of tested molecules. The plates were allowed to incubate for 1, 2, and 4 h at 37 °C. The positive control was studied strain in the using medium alone. After incubation, each well was washed with PBS solution three times and measured at OD 595 nm.For inhibition of the biofilm formation, the tested strain was incubated in its medium and molecules at 1× and 1/10× in addition to 1/100× MIC in 37 °C for 24 h in microtiter plates. Six wells were used for each molecules. The positive control was the tested strain in its medium without molecules. After incubation, each well was washed with PBS solution three times and measured at OD 595 nm.
4.5. Statistical analysis
All experiments were performed in two independent assays. Two-way ANOVA-Tukey's multiple comparison test was used to compare differences between control and antimicrobials treated biofilms. P value <0.005 was considered as statistically significant.
4.6. Molecular docking
S. aureus DNA gyrase X-ray crystal structure (PDB code 3G75) [9] was retrieved from Protein Data Bank to be utilized as a model in the present study. The protein structure was prepared using QuickPrep module of MOE (version 2019.01, Chemical Computing Group Inc., Montreal, QCstate abbrev, Canada). Only one monomer, chain A, was selected and water residues were deleted. The docking study was conducted using the rigid-receptor method.69,70 The co-crystallized ligand B48 was defined as the center of the binding site. Using the MOE build suite, the chemical structures were drawn, and then energy-minimized using the MOE default force field.71 All other docking options were kept at their default values. Fifteen docking positions were generated for each ligand. The generated docking positions were visualized using MOE.
4.7. In silico drug-likeness and ADMET analysis
The online SwissADME server was employed to predict the drug-likeness and ADME parameters.72
Conflicts of interest
The authors declare no conflict of interest.
Acknowledgements
This work was financially supported by the Scientific Research Projects Coordination Unit of Istanbul University (Project numbers: FBA-2021-37260) for supplying the equipment and materials.
References
- I. Abraham, R. Joshi, P. Pardasani and R. T. Pardasani, J. Braz. Chem. Soc., 2011, 22, 385–421 CrossRef CAS.
- C. E. Sansom, L. Larsen, N. B. Perry, M. V. Berridge, E. W. Chia, J. L. Harper and V. L. Webb, J. Nat. Prod., 2007, 70, 2042–2044 CrossRef CAS PubMed.
- S. Nojima, C. Schal, F. X. Webster, R. G. Santangelo and W. L. Roelofs, Science, 2005, 307, 1104–1106 CrossRef CAS PubMed.
- D. Tasdemir, R. Brun, V. Yardley, S. G. Franzblau and P. Rüedi, Chem. Biodiversity, 2006, 3, 1230–1237 CrossRef CAS PubMed.
- H. Bauer, K. Fritz-Wolf, A. Winzer, S. Kuhner, S. Little, V. Yardley, H. Vezin, B. Palfey, R. H. Schirmer and E. Davioud-Charvet, J. Am. Chem. Soc., 2006, 128, 10784–10794 CrossRef CAS PubMed.
- L. S. Lara, G. C. Lechuga, C. D. S. Moreira, T. B. Santos, V. F. Ferreira, D. R. da Rocha and M. C. S. Pereira, Molecules, 2021, 26(2), 423 CrossRef CAS PubMed.
- M. Yamashita, J. Sawano, R. Umeda, A. Tatsumi, Y. Kumeda and A. Iida, Chem. Pharm. Bull., 2021, 69, 661–673 CrossRef CAS PubMed.
- N. Bayrak, H. I. Ciftci, M. Yildiz, H. Yildirim, B. Sever, H. Tateishi, M. Otsuka, M. Fujita and A. F. Tuyun, Chem.-Biol. Interact., 2021, 345, 109555 CrossRef CAS PubMed.
- A. T. Jannuzzi, M. Yıldız, N. Bayrak, H. Yıldırım, D. Shilkar, V. Jayaprakash and A. F. TuYuN, Chem.-Biol. Interact., 2021, 349, 109673 CrossRef CAS PubMed.
- H. Yildirim, N. Bayrak, M. Yildiz, E. M. Kara, B. O. Celik and A. F. Tuyun, J. Mol. Struct., 2019, 1195, 681–688 CrossRef CAS.
- C. Varricchio, K. Beirne, P. Aeschlimann, C. Heard, M. Rozanowska, M. Votruba and A. Brancale, J. Med. Chem., 2020, 63, 13638–13655 CrossRef CAS PubMed.
- K. W. Wellington, RSC Adv., 2015, 5, 20309–20338 RSC.
- J. S. Novais, M. F. Carvalho, M. S. Ramundo, C. O. Beltrame, R. B. Geraldo, A. K. Jordao, V. F. Ferreira, H. C. Castro and A. M. S. Figueiredo, Sci. Rep., 2020, 10, 19631 CrossRef CAS PubMed.
- F. R. F. Dias, J. S. Novais, T. A. D. S. Devillart, W. A. da Silva, M. O. Ferreira, R. D. Loureiro, V. R. Campos, V. F. Ferreira, M. C. B. V. de Souza, H. C. Castro and A. C. Cunha, Eur. J. Med. Chem., 2018, 156, 1–12 CrossRef CAS PubMed.
- K. W. Wellington, N. B. P. Nyoka and L. J. McGaw, Drug Dev. Res., 2019, 80, 386–394 CrossRef CAS PubMed.
- P. Ravichandiran, M. Maslyk, S. Sheet, M. Janeczko, D. Premnath, A. R. Kim, B. H. Park, M. K. Han and D. J. Yoo, Chemistryopen, 2019, 8, 589–600 CrossRef CAS PubMed.
- A. Alfadhli, A. Mack, L. Harper, S. Berk, C. Ritchie and E. Barklis, Bioorg. Med. Chem., 2016, 24, 5618–5625 CrossRef CAS PubMed.
- O. P. S. Patel, R. M. Beteck and L. J. Legoabe, Eur. J. Med. Chem., 2021, 210, 113084 CrossRef CAS PubMed.
- A. Kosiha, K. M. Lo, C. Parthiban and K. P. Elango, Mater. Sci. Eng., C, 2019, 94, 778–787 CrossRef CAS PubMed.
- A. Kosiha, C. Parthiban and K. P. Elango, J. Coord. Chem., 2018, 71, 1560–1574 CrossRef CAS.
- P. Jayasudha, R. Manivannan, S. Ciattini, L. Chelazzi and K. P. Elango, Sens. Actuators, B, 2017, 242, 736–745 CrossRef CAS.
- C. Parthiban and K. P. Elango, Sens. Actuators, B, 2017, 245, 321–333 CrossRef CAS.
- J. Wang, F. Xia, W.-B. Jin, J.-Y. Guan and H. Zhao, Bioorg. Chem., 2016, 68, 214–218 CrossRef CAS PubMed.
- P. J. O'Brien, Chem.-Biol. Interact., 1991, 80, 1–41 CrossRef.
- I. N. Okeke, K. P. Klugman, Z. A. Bhutta, A. G. Duse, P. Jenkins, T. F. O'Brien, A. Pablos-Mendez and R. Laxminarayan, Lancet Infect. Dis., 2005, 5, 568–580 CrossRef PubMed.
- G. J. Basset, S. Latimer, A. Fatihi, E. Soubeyrand and A. Block, Mini-Rev. Med. Chem., 2017, 17, 1028–1038 CrossRef CAS PubMed.
- V. Weissig, Trends Mol. Med., 2020, 26, 40–57 CrossRef CAS PubMed.
- G. M. Enns, J. Child Neurol., 2014, 29, 1235–1240 CrossRef PubMed.
- T. Meier and G. Buyse, J. Neurol., 2009, 256, 25–30 CrossRef CAS PubMed.
- E. M. Yubero-Serrano, L. Gonzalez-Guardia, O. Rangel-Zuñiga, N. Delgado-Casado, J. Delgado-Lista, P. Perez-Martinez, A. Garcia-Rios, J. Caballero, C. Marin, F. M. Gutierrez-Mariscal, F. J. Tinahones, J. M. Villalba, I. Tunez, F. Perez-Jimenez and J. Lopez-Miranda, Age, 2013, 35, 159–170 CrossRef CAS PubMed.
- S. Pepe, S. F. Marasco, S. J. Haas, F. L. Sheeran, H. Krum and F. L. Rosenfeldt, Mitochondrion, 2007, 7, S154–S167 CrossRef CAS PubMed.
- A. F. Tuyun, M. Yildiz, N. Bayrak, H. Yildirim, E. M. Kara, A. T. Jannuzzi and B. O. Celik, Drug Dev. Res., 2019, 80, 1098–1109 CrossRef CAS PubMed.
- N. Bayrak, H. Yildirim, A. F. Tuyun, E. M. Kara, B. O. Celik, G. K. Gupta, H. I. Ciftci, M. Fujita, M. Otsuka and H. R. Nasiri, Lett. Drug Des. Discovery, 2017, 14, 647–661 CAS.
- H. Yildirim, N. Bayrak, A. F. Tuyun, E. M. Kara, B. O. Celik and G. K. Gupta, RSC Adv., 2017, 7, 25753–25764 RSC.
- E. Mataraci-Kara, N. Bayrak, H. Yildirim, M. Yildiz, M. Ataman, B. Ozbek-Celik and A. F. Tuyun, Med. Chem. Res., 2021, 30, 1728–1737 CrossRef CAS.
- N. Bayrak, M. Yildiz, H. Yildirim, E. M. Kara, B. O. Celik and A. F. Tuyun, J. Mol. Struct., 2020, 1219, 128560 CrossRef CAS.
- E. M. Kara, N. Bayrak, H. Yildirim, M. Yildiz, B. O. Celik and A. F. Tuyun, Folia Microbiol., 2020, 65, 785–795 CrossRef CAS PubMed.
- H. I. Ciftci, N. Bayrak, M. Yıldız, H. Yıldırım, B. Sever, H. Tateishi, M. Otsuka, M. Fujita and A. F. Tuyun, Bioorg. Chem., 2021, 114, 105160 CrossRef CAS PubMed.
- E. Mataraci-Kara, N. Bayrak, M. Yildiz, H. Yildirim, B. Ozbek-Celik and A. F. Tuyun, Drug Dev. Res., 2021, 83(3), 628–636 Search PubMed.
- C. M. Petit and K. K. Koretke, Biochem. J., 2002, 363, 825–831 CrossRef CAS PubMed.
- T. A. Keating, J. V. Newman, N. B. Olivier, L. G. Otterson, B. Andrews, P. A. Boriack-Sjodin, J. N. Breen, P. Doig, J. Dumas, E. Gangl, O. M. Green, S. Y. Guler, M. F. Hentemann, D. Joseph-McCarthy, S. Kawatkar, A. Kutschke, J. T. Loch, A. R. McKenzie, S. Pradeepan, S. Prasad and G. Martínez-Botella, ACS Chem. Biol., 2012, 7, 1866–1872 CrossRef CAS PubMed.
- S. Keinan, W. D. Paquette, J. J. Skoko, D. N. Beratan, W. Yang, S. Shinde, P. A. Johnston, J. S. Lazo and P. Wipf, Org. Biomol. Chem., 2008, 6, 3256–3263 RSC.
- C. Acar, G. Yalçın, T. Ertan-Bolelli, F. Kaynak Onurdağ, S. Ökten, F. Şener and İ. Yıldız, Bioorg. Chem., 2020, 94, 103368 CrossRef CAS PubMed.
- M. Cerone, E. Uliassi, F. Prati, G. U. Ebiloma, L. Lemgruber, C. Bergamini, D. G. Watson, T. D. M. Ferreira, G. S. H. R. Cardoso, L. A. S. Romeiro, H. P. de Koning and M. L. Bolognesi, Chemmedchem, 2019, 14, 621–635 CrossRef CAS PubMed.
- C. Ibis, A. F. Tuyun, Z. Ozsoy-Gunes, H. Bahar, M. V. Stasevych, R. Y. Musyanovych, O. Komarovska-Porokhnyavets and V. Novikov, Eur. J. Med. Chem., 2011, 46, 5861–5867 CrossRef CAS PubMed.
- Clinical and Laboratory Standards Institute (CLSI), Performance Standards for Antimicrobial Susceptibility Testing, Clinical and Laboratory Standards Institute, 950 West Valley Road, Suite 2500, Wayne, Pennsylvania 19087, USA, 2020 Search PubMed.
- Clinical and Laboratory Standards Institute (CLSI), Reference Method for Broth Dilution Antifungal Susceptibility Testing of Yeasts; Approved Standard-Second Edition, Wayne, PA, USA, 1997 Search PubMed.
- K. Yilancioglu, Z. B. Weinstein, C. Meydan, A. Akhmetov, I. Toprak, A. Durmaz, I. Iossifov, H. Kazan, F. P. Roth and M. Cokol, J. Chem. Inf. Model., 2014, 54, 2286–2293 CrossRef CAS PubMed.
- E. Mataraci-Kara and B. Ozbek-Celik, J. Chemother., 2018, 30, 82–88 CrossRef CAS PubMed.
- R. Mishra, A. K. Panda, S. De Mandal, M. Shakeel, S. S. Bisht and J. Khan, Front. Microbiol., 2020, 11, 566325 CrossRef PubMed.
- R. Roy, M. Tiwari, G. Donelli and V. Tiwari, Virulence, 2018, 9, 522–554 CrossRef CAS PubMed.
- J. W. Costerton, Z. Lewandowski, D. E. Caldwell, D. R. Korber and H. M. Lappin-Scott, Annu. Rev. Microbiol., 1995, 49, 711–745 CrossRef CAS PubMed.
- J. Sun, P.-C. Lv, Y. Yin, R.-J. Yuan, J. Ma and H.-L. Zhu, PLoS One, 2013, 8, e69751 CrossRef CAS PubMed.
- T. Khan, K. Sankhe, V. Suvarna, A. Sherje, K. Patel and B. Dravyakar, Biomed. Pharmacother., 2018, 103, 923–938 CrossRef CAS PubMed.
- S. J. Teague, A. M. Davis, P. D. Leeson and T. Oprea, Angew. Chem., Int. Ed. Engl., 1999, 38, 3743–3748 CrossRef CAS PubMed.
- C. A. Lipinski, F. Lombardo, B. W. Dominy and P. J. Feeney, Adv. Drug Delivery Rev., 2001, 46, 3–26 CrossRef CAS PubMed.
- A. K. Ghose, V. N. Viswanadhan and J. J. Wendoloski, J. Comb. Chem., 1999, 1, 55–68 CrossRef CAS PubMed.
- D. F. Veber, S. R. Johnson, H.-Y. Cheng, B. R. Smith, K. W. Ward and K. D. Kopple, J. Med. Chem., 2002, 45, 2615–2623 CrossRef CAS PubMed.
- W. J. Egan, K. M. Merz Jr and J. J. Baldwin, J. Med. Chem., 2000, 43, 3867–3877 CrossRef CAS PubMed.
- I. Muegge, S. L. Heald and D. Brittelli, J. Med. Chem., 2001, 44, 1841–1846 CrossRef CAS PubMed.
- J. S. Delaney, J. Chem. Inf. Comput. Sci., 2004, 44, 1000–1005 CrossRef CAS PubMed.
- Y. C. Martin, J. Med. Chem., 2005, 48, 3164–3170 CrossRef CAS PubMed.
- Bruker, APEX2, version 2014.1-1, Bruker AXS Inc., Madison, WI, 2014 Search PubMed.
- Bruker, SAINT, version 8.34A, Bruker AXS Inc., Madison, WI, 2013 Search PubMed.
- Bruker, SADABS, version 2012/2, Bruker AXS Inc., Madison, WI, 2012 Search PubMed.
- Bruker, SHELXTL, version 6.14, Bruker AXS Inc., Madison, WI, 2000 Search PubMed.
- A. L. Spek, Acta Crystallogr., Sect. D: Biol. Crystallogr., 2009, 65, 148–155 CrossRef CAS PubMed.
- C. F. Macrae, P. R. Edgington, P. McCabe, E. Pidcock, G. P. Shields, R. Taylor, M. Towler and J. van De Streek, J. Appl. Crystallogr., 2006, 39, 453–457 CrossRef CAS.
- H. Tateishi, M. Tateishi, M. O. Radwan, T. Masunaga, K. Kawatashiro, Y. Oba, M. Oyama, N. Inoue-Kitahashi, M. Fujita, Y. Okamoto and M. Otsuka, Chem. Pharm. Bull., 2021, 69, 1123–1130 CrossRef CAS PubMed.
- M. O. Radwan, D. Takaya, R. Koga, K. Iwamaru, H. Tateishi, T. F. S. Ali, A. Takaori-Kondo, M. Otsuka, T. Honma and M. Fujita, Bioorg. Med. Chem., 2020, 28, 115409 CrossRef CAS PubMed.
- D. Mingle, M. Ospanov, M. O. Radwan, N. Ashpole, M. Otsuka, S. A. Ross, L. A. Walker, A. G. Shilabin and M. A. Ibrahim, Med. Chem. Res., 2021, 30, 98–108 CrossRef CAS PubMed.
- A. Daina, O. Michielin and V. Zoete, Sci. Rep., 2017, 7, 42717 CrossRef PubMed.
|
This journal is © The Royal Society of Chemistry 2022 |