DOI:
10.1039/D2RA01521H
(Paper)
RSC Adv., 2022,
12, 20062-20073
The effective removal of Pb2+ by activated carbon fibers modified by L-cysteine: exploration of kinetics, thermodynamics and mechanism
Received
10th March 2022
, Accepted 12th June 2022
First published on 11th July 2022
Abstract
Herein, we developed a low-cost fabrication route to prepare chemically grafted activated carbon fibers, which effectively removed Pb2+ from solution. Multiple characteristic results indicated that L-cyst-ACF had abundant nitrogen-containing and sulfur-containing functional groups. Based on the XPS and EDS analyses, the capture of Pb2+ was attributed to the abundant adsorption sites on the fiber surface. According to the analysis of the pseudo-second-order kinetic model and the Langmuir isotherm model, the adsorption process could be interpreted as monolayer adsorption and chemisorption, and the equilibrium adsorption capacity was determined to be 136.80 mg g−1 by fitting the pseudo-second-order kinetic model. The maximum adsorption capacity of L-cyst-ACF for Pb2+ was calculated to be 179.53 mg g−1 using the Langmuir model. In addition, the adsorption reaction was endothermic and spontaneous, as evidenced by the thermodynamic parameters. The outcomes of this study provide a low-cost and feasible strategy for the remediation of Pb2+ pollution in the environment.
1 Introduction
The rapid development of modern industry has produced a large amount of wastewater polluted with heavy metals, which has caused great ecological and environmental harm, and finally poses a threat to human health through the food chain.1,2 Heavy metal ions are non-biodegradable carcinogenic factors and tend to accumulate in organisms.3,4 Heavy metals can enter the food chain through plants and pose a serious threat to human health.5 Common heavy metal ions can cause acute and chronic diseases such as digestive inflammation, brain injury and cancers.6 Therefore, the removal of heavy metal ions from industrial wastewater has become a major pro-environmental problem in recent years.
Various technologies to remove heavy metals from water are available, such as solvent extraction,7 ion exchange,8 precipitation9,10 and adsorption.11 Among them, adsorption has the advantages of simple operation, high efficiency, and strong flexibility. Many materials have been used to remove heavy metals such as activated carbon,12,13 polymers,14,15 and nanomaterials.16 However, the practical application of adsorbents is hindered by their low adsorption efficiency for the target metal, the inconvenient process for their synthesis of and their unachievable recycling. Therefore, the development of advanced adsorbents with high adsorption capacity, recyclability and good chemical stability has become a vital research direction in the field of heavy metal remediation.
Activated carbon fibers (ACFs) are a new type of adsorbent developed in recent years to remove pollutants in aqueous solutions. Compared with the traditional activated carbon particles, activated carbon fibers have more suitable pore sizes, better adsorption effect and easy recycling.17–19 Javier20 et al. measured the effect of activated carbon fibers to adsorb both Pb2+-phenol and Cd2+-phenol simultaneously, proving that the selectivity of activated carbon fibers for Pb2+ ions was greater than Cd2+ ions. Deng18 et al. found that a novel thiosemicarbazide-modified adsorbent exhibited a high performance towards Cd(II) and Pb (II). This result explained that sulfur-containing groups and nitrogen-containing groups could effectively adsorb metal ions in solution by chelation and coordination.
In the present study, activated carbon fiber adsorbents grafted with multiple functional groups with high adsorption properties were prepared via a simple amide reaction. The preparation fibers were characterization through multiple technologies such as Fourier transform infrared spectroscopy (FT-IR), X-ray photoelectron spectroscopy (XPS), scanning electron microscopy (SEM) and energy dispersive X-ray analysis (EDX). Meanwhile, the behavior and mechanism of Pb2+ adsorption were comprehensively explored via adsorption kinetics, isotherm models, and thermodynamic parameters. Then, the adsorption performance of L-cyst-ACF for Pb2+ was systematically discussed, which suggested that the adsorption process was spontaneous and endothermic.
2 Materials and methods
2.1 Materials
Activated carbon fibers were obtained from Jiangsu Sutong Carbon Fiber Co., Ltd (Suzhou, China). Thiourea (AR, 99%), N-(3-dimethylaminopropyl)-N′-ethyl-carbodiimide hydrochloride (EDC, AR, 99%), N-hydroxy-succinimide (NHS, AR, 99%), and NaHSO4 (AR, 99%) were purchased from Aladdin Regent Co. Ltd (Shanghai, China). Pb (NO3)2 (AR, 99%) was produced from Sinopharm Chemical Reagent Co, Ltd (Shanghai, China). Na2S (AR, 98%) was procured from Macklin Biochemical Technology Co., Ltd (Shanghai, China). Ethyl alcohol (AR, 99.9%) was bought from Jiangsu Qiangsheng Functional Chemistry Co., Ltd (Suzhou, China). Dichloromethane (AR, 99.5%) was produced from Hangzhou Shuanglin Chemical Reagent Co., Ltd (Hangzhou, China). HNO3 (AR 65%) and H2SO4 (AR 98%) were bought from Sinopharm Chemical Reagent Co, Ltd (Shanghai, China). L-Cysteine hydrochloride monohydrate (AR 99%), KH2PO4 (AR 99.5%), and Na2HPO4 (AR 99%) were all bought from Aladdin Regent Co. Ltd (Shanghai, China).
2.2 Preparation of L-Cysteine-modified activated carbon fiber
Activated carbon fibers were washed with deionized water several times and dried for 12 h at 80 °C until a constant weight. Then, the clean fibers were cut into small pieces and soaked in a HNO3/H2SO4 (3
:
1, v
:
v) solution for acidification. The fibers were washed several times and dried overnight. ACFs were put in an appropriate amount of KH2PO4/Na2HPO4 buffer solution with a pH of 8.5. EDC (1.8 g) and NHS (1.2 g) were added to the solution with constant stirring at room temperature, and then thiourea (1.52 g) was added. After 6 h, ACFs were washed and dried.
Firstly, 1.38 g of sodium bisulfate (NaHSO4) was added to 100 mL of 0.1 m L−1 dilute hydrochloric acid solution, and then 80 mL of ethanol was added. Subsequently, ACF and L-cysteine (1.7563 g) were added to the above mixture, and the system was heated at reflux at 95 °C for 6 h. The product was washed with ethanol and deionized water several times, and then dried at 80 °C in vacuo for 24 h. To reduce the oxidized sulfhydryl group (–SH), ACFs were added to an organic solvent (dichloromethane:ethyl alcohol, v
:
v = 1
:
2), Na2S was added to the mixture as a reducing agent, and then it was stirred at room temperature for a certain of time. Finally, ACFs were filtered and washed several times, and then stored after drying. The specific experimental steps are shown in Fig. 1.
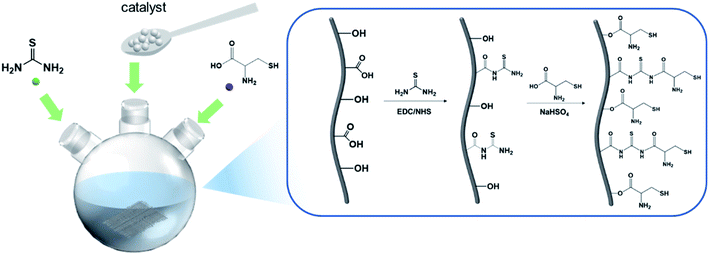 |
| Fig. 1 Process for the preparation of cysteine-modified activated carbon fibers. | |
2.3 Characterization of L-cyst-ACF
The properties of L-cyst-ACF were characterized via a series of performance tests. Fourier-transform infrared spectra (FTIR) were recorded in pressed KBr using a Vertex 70 spectrophotometer (Bruker) in the range of 500–4000 cm−1 at room temperature. The morphological characteristics of L-cyst-ACF were observed via SEM (Hitachi, SU8010). The elemental components of L-cyst-ACF before and after adsorption were identified by X-ray photoelectron spectroscopy (XPS, Thermo Fisher: ESCALAB 250Xi, single Al Kα as the light excitation source). An Oxford X-MaxN scanning electron microscope (SEM)/energy dispersive X-ray spectroscopy (EDS) was used to observe the surface morphology and element distribution of the fiber, respectively. Inductively coupled plasma atomic emission spectrometry (ICP-MS Agilent: 7800, China) was used to detect the Pb(II) concentration.
2.4 Batch adsorption experiments
A sequence of batch adsorption experiments was conducted to investigate the performance of the synthesized adsorbents for the elimination of Pb2+ from aqueous solution. The adsorption experiments were performed in an SHA-B water bath oscillator (RongHua Instrument Manufacturing Co., Ltd, China) at a shaking speed of 120 rpm. Batch experiments were performed to investigate the kinetics, isothermal models, and thermodynamic parameters during the adsorption process. In this investigation, the initial metal ion concentration range was 0–500 mg L−1, adsorbent dose range was from 50 to 150 mg and the system temperatures were 303 K, 313 K and 323 K, respectively. The pH range of 2–6 was adopted in the experiment to investigate the influence of pH. All batch experimental solution concentrations were detected through inductively coupled plasma atomic emission spectrometry. Before the solution concentration test, the test liquids were filtered with a 0.22 μm filter to remove impurities.
The adsorption amount qe (mg g−1) of Pb2+ in adsorption equilibrium was calculated using eqn (1):
|
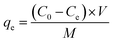 | (1) |
The removal efficiency of Pb2+ at equilibrium was calculated using eqn (2):
|
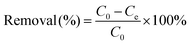 | (2) |
where
C0 and
Ce are the initial concentration and equilibrium concentration (mg L
−1), respectively.
V (L) is the volume of the solution and
M (g) is the weight of the absorbent.
3 Results and discussion
3.1 Characterization of ACF and L-cyst-ACF
The FT-IR spectra of ACF and L-cyst-ACF are shown in Fig. 2. In the spectrum of the activated carbon fibers, the band at 3442 cm−1 is ascribed to the –OH stretching vibration21 and that at 1654 cm−1 is ascribed to the C
O stretching.22 The peaks at 1541 cm−1 and 1388 cm−1 correspond to the C–C and C–O bonds, respectively.23 Similarly, the peak at 3446 cm−1 in the spectrum of L-cyst-ACF represents the –OH stretching vibration, and the two peaks at 1527 cm−1 and 1386 cm−1 can be ascribed to the C–C and C–O groups, respectively.24 The peaks at 2813 cm−1 and 2933 cm−1 are attributed to the stretching vibrations of –CH2 and –CH3, respectively. The sharp peak at 3471 cm−1 and the broad band at around 3100–3300 cm−1 are attributed to the –NH2 vibration.25 Obviously, the sharp peak at 1655 cm−1 can be assigned to the carbonyl stretch of the –NHCO– group, not just the carbonyl group (C
O).24 Meanwhile, a weak peak located at 2401 cm−1 was observed, which can be attributed to the –SH bending vibration.18,26 The small peak at 1267 cm−1 is ascribed to the C
S bond.12 The band at 1000 cm−1 may be caused by the C–N stretching.27 In addition, the peaks at 740 cm−1 and 632 cm−1 correspond to the bending vibration of –NH2 and vibrational C–S bonds, respectively.28
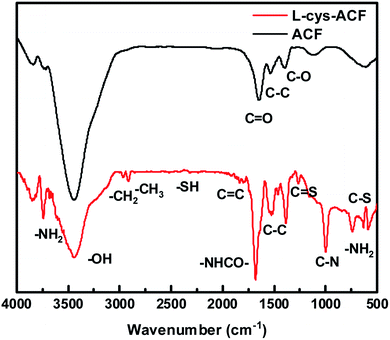 |
| Fig. 2 FT-IR spectra of activated carbon fibers and L-cysteine-modified activated carbon fibers. | |
3.2 Characterization of ACF-SH
The SEM images of ACF and L-cyst-ACF are shown in Fig. 3. The SEM image of ACF (Fig. 3a) displays a regular smooth surface; meanwhile, that of L-cyst-ACF (Fig. 3b) shows an irregular rough surface after modification, which illustrates that organo-functional groups were successfully grafted. Alternatively, a larger specific surface area is also conducive to an improvement in adsorption capability. The changes on the surface of the modified ACF after adsorption are showed in Fig. 3(c and d). It can be observed that some irregular tiny particles covered the surface of the fibers after adsorption, demonstrating the further improved effective adsorption ability of L-cyst-ACF to remove Pb2+ ions.
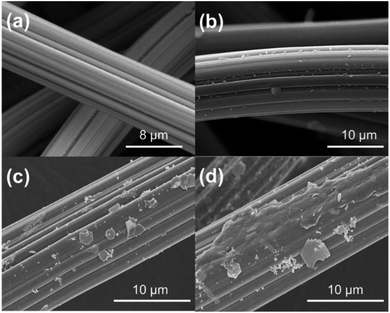 |
| Fig. 3 SEM images of ACF and L-cyst-ACF (a) original ACF, (b) L-cyst-ACF (c and d) after Pb2+ adsorbed on L-cyst-ACF. | |
The energy dispersive X-ray spectroscopy mapping of L-cyst-ACF is displayed in Fig. 4, which authenticated the presence of elements including carbon (C), oxygen (O), sulfur (S) and nitrogen (N) related to the presence of L-cysteine modified on ACF. As shown as Fig. 4(B–F), the successful grafting reaction of functional groups occurred on ACF. According to the data, it can be concluded that O and N are not co-localized with Pb. Fig. 4E and F show strong S and Pb signals, respectively, which are co-localized, suggesting that the primary adsorbent for Pb is S and that N and O did not significantly contribute to the adsorption.
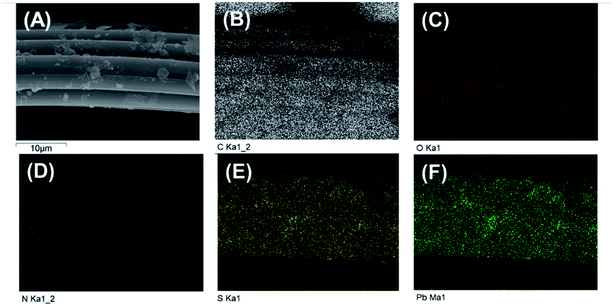 |
| Fig. 4 (A) SEM image of L-cyst-m-ACF after adsorption (B)–(F) EDS mapping of L-cyst-m-ACF of C, O, S, N, and Pb elements after the adsorption of Pb2+. | |
Fig. 5A reveals the chemical composition changes in the modified activated carbon fibers compared with the original fiber according to the XPS analysis. The presence of amine groups confirmed that the grafting reaction occurred. Additionally, the ratio of different elements illustrates that the organic functional groups were successfully grafted, as shown in Fig. 5B. EDS mapping, FT-IR, and XPS analyses were employed to confirm the adsorption of Pb2+ on L-cyst-ACF.
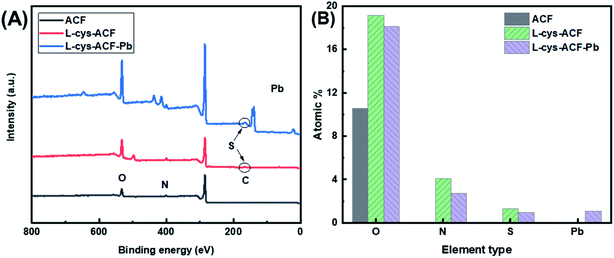 |
| Fig. 5 (a) XPS spectra of base ACF, L-cyst-ACF and L-cyst-ACF-Pb. (b) Composition of fiber samples determined by XPS analysis. | |
3.3 Lead adsorption
3.3.1 Contact time. The exploration of the adsorption equilibrium time is a crucial part of measuring the performance of an adsorbent and it is significance for subsequent research.29 The change in the adsorption of the material with contact time is presented in Fig. 6. It shows that the adsorption capacity of Pb2+ increased rapidly within the first hour, which can be ascribed to the effective complexation of organic groups with Pb2+.30,31 Then, it remained at approximately 125 mg g−1 for Pb2+ after 6 h, indicating that adsorption equilibrium had been achieved.
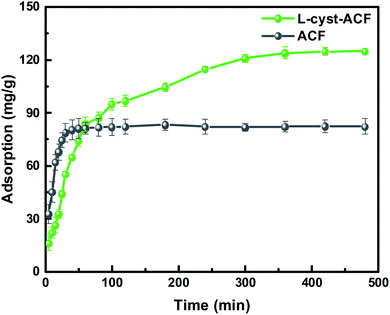 |
| Fig. 6 Effect of contact time on the adsorption capacity of Pb2+ (pH = 5.5, temperature = 303 K, C0 = 100 mg L−1, and V = 100 mL). | |
3.3.2 Different adsorbent dosages. The removal efficiency of Pb2+ with different adsorbent dosages was also investigated. As shown in Fig. 7, the removal rate of Pb2+ increased significantly as the dosage increased. When the dosage increased from 0.25 g L−1 to 0.5 g L−1, the removal of Pb2+ increased from 23.78% to 51.55%. Simultaneously, the degradation rate of Pb2+ did not increase significantly with an increase in the adsorption dosage. However, the removal efficiency of L-cyst-ACF was limited when the adsorption dosage was greater than 0.5 g L−1, indicating that a larger adsorption dosage had a greater impact on the further removal of Pb2+. Thus, we chose the dosage of L-cyst-ACF to be 0.5 g L−1 for the subsequent removal process.
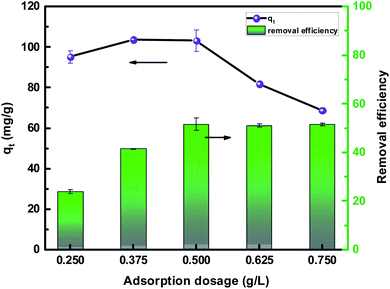 |
| Fig. 7 Effect of different adsorbent dosages on the adsorption capacity and removal efficiency of Pb2+ (C0 = 100 mg L−1, V = 100 mL, contact time = 6 h, and temperature = 303 K). | |
3.3.3 Initial pH. Research has shown that the surface charge of the absorbent material, the degree of ion migration and the state of the adsorbed substance are affected by the pH of the solution during the adsorption process.32 Fig. 8a shows that the amount of adsorption observably increased with an increase in pH. This phenomenon demonstrated that the existence of a large amount of H+ ions competed with Pb2+ ions for the adsorption sites in the material in an acidic environment.31 It is generally believed that lead ions exist as cations in aqueous solutions.33,34 However, Pb2+ ions easily form Pb(OH)2 (precipitation) with OH− when the pH is greater than 6.35 Thus, zeta potential (ζ) measurements at different pH were performed to explore the surface charge of the absorbent, as shown in Fig. 8b. Fig. 8b shows that the surface of the material exhibited a negative charge state as the pH increased. Because the functional groups such as –NH2 and –SH on the material surface lost H− ions as the OH− gradually increased in the solution, and they became the active state.36 This active state was conducive to the adsorption of Pb2+ ions by the material. On the contrary, the hydroxyl, carboxyl, amine and sulfhydryl groups on the surface of L-cyst-ACF tended to be protonated, and they were positively charged when the pH value was low, which led to electrostatic repulsion between the binding site and Pb2+ ions.15,37,38 Therefore, the pH of the solution and the surface charge of the material were the key factors that affected its adsorption capacity.
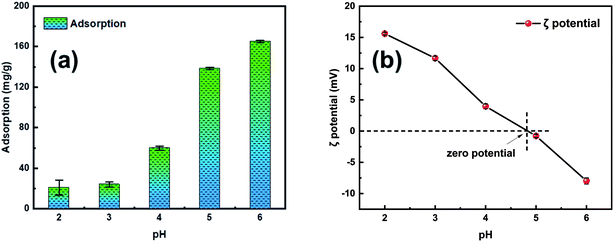 |
| Fig. 8 Effect of initial pH on Pb2+ adsorption and zeta potential of L-cyst-ACF during removal process at different pH. | |
3.4 Adsorption kinetics
To quickly and accurately interpret the adsorption behavior of materials, four types of kinetic models were adopted in this investigation. The linear types of models are expressed as eqn (3)–(6).39,40 |
ln(qe − qt) = ln qe − K1·t
| (3) |
|
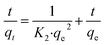 | (4) |
|
 | (5) |
|
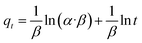 | (6) |
where qe and qt are the adsorption capacity at equilibrium and time t (mg g−1).36 K1 (min−1) and K2 [g·(mg min)−1] are the rate constant. Ki is the rate constant of diffusion particles.41 α [mg (g min)−1] and β (g mg−1) represent the initial constant adsorption and desorption constants, respectively.42
According to the fitting results in Fig. 9 and Table 1, it can be seen that the R2 values of the pseudo-second-order and intra-particle-diffusion kinetic models were better than that of the pseudo-first-order kinetic models and Elovich model, indicating that the possible mechanism of adsorption is chemisorption and the adsorbent surface corresponds to a heterogeneous system. Furthermore, this result reveals that the adsorption is related to the electron exchange between the surface functional groups and Pb2+ ions. The experimental equilibrium adsorption capacity, qe (136.80 mg g−1), obtained by fitting the pseudo-second-order kinetic equation maintained high consistency with the actual test value (∼125 mg g−1).
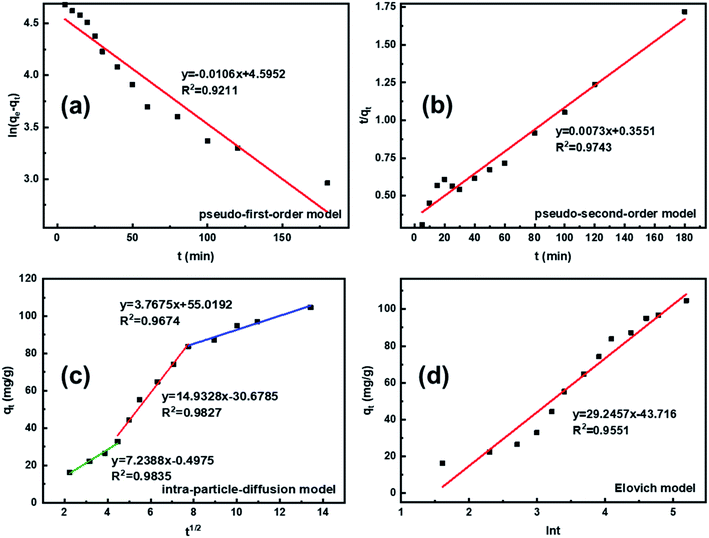 |
| Fig. 9 (a) Plots of pseudo-first-order kinetic model for the adsorption of Pb2+, (b) pseudo-second-order kinetic model for the adsorption of Pb2+, (c) intra-particle-diffusion kinetic model for the adsorption of Pb2+, (d) Elovich kinetic model for the adsorption of Pb2+. Conditions: T = 303 K [adsorbent] = 0.5 g L−1 [metal] = 100 mg L−1, pH = 5.5, and contact time = 6 h. | |
Table 1 The parameters of four types of kinetic models
Kinetic model |
Parameter |
Value |
Pseudo-first-order model |
qe (mg g−1) |
99.01 |
K1 (min−1) |
0.0106 |
R2 |
0.9211 |
Pseudo-second-order model |
qe (mg g−1) |
136.80 |
K2 [g·(mg min)−1] |
0.0073 |
R2 |
0.9743 |
Intraparticle diffusion model |
K1 [mg·(g·min1/2)−1] |
7.2388 |
K2 [mg·(g·min1/2)−1] |
14.9328 |
K3 [mg·(g·min1/2)−1] |
3.7675 |
Elovich model |
α [mg·(g min)−1] |
6.5584 |
β (g mg−1) |
0.0342 |
R2 |
0.9551 |
To investigate the internal diffusion mechanism during the adsorption process, the intra-particle diffusion model proposed by Weber and Morris43 was used to discuss the rate-controlling step. Multi-linearity was observed, as shown in Fig. 9c, and the crucial information obtained is presented in Table 1. As shown in Fig. 9c, the Pb2+ adsorption process involved three steps. The initial linear part was the result of adsorption on the external surface, namely, the attachment of Pb2+ to the surface of L-cyst-ACF.44 The second linear part represents the progressive internal diffusion of particles.12 In the third part, the decreased diffusion rate is due to the reduction in Pb2+ concentration and the enhanced electrostatic repulsion on the material surface.45 The intra-particle diffusion and complex or ion exchange may play a certain role in the adsorption process given that none of the three linear curves passed through the origin of the coordinate.23 Combined with the characterization test and mechanism investigation, we realized that the relatively rough surface on the fiber surface and the partially oxidized thiol functional groups generated sulfonic acid groups, which reduced contact probability between the Pb2+ in solution and active sites on the material surface, affecting the transfer rate of Pb2+ in the surface liquid layer. Comparatively, the ion transport rate in the pore was larger. The α and β constants also indicated that the material had a low adsorption rate and a non-homogeneous surface structure.
3.5 Adsorption isotherms
Two common isothermal models were used to evaluate the adsorption behavior between Pb2+ and L-cyst-ACF. The linear form of the Langmuir isotherm is represented by the following equation:46 |
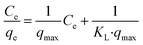 | (7) |
where qe and qmax represent the equilibrium adsorption capacity and monolayer maximum adsorption capacity (mg g−1), respectively.18 The constant KL describes the affinity between the adsorbent and the target substance.12
The Freundlich model is described by the following equation:46,47
|
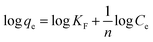 | (8) |
where
KF and 1/
n are empirical constants expressed the adsorption intensity and

.
48
The experimental results obtained by fitting the Langmuir and Freundlich models are shown in Fig. 10. The affinity and adsorption capacity of L-cyst-ACF for Pb2+ at different temperatures were evaluated. The constants for the two models are summarized in Table 2. The Langmuir model is normally used to evaluate the monolayer adsorption characteristics and energy level of a homogeneous material surface.49 The R2 value from the Langmuir model was higher than that from the Freundlich model, which indicates the adsorption of Pb2+ was primarily homogeneous and monolayer adsorption. The low KL value suggests a relatively strong interaction between Pb2+ and L-cyst-ACF. Compared with the results in other works, as shown in Table 3, that herein exhibited a higher adsorption effect. The Freundlich model generally describes the multi-layer adsorption of the adsorbent on a heterogeneous surface and that the energy level of the adsorption site is not constant.43 Meanwhile, the poor fitting data showed that the adsorption was not physical adsorption or multilayer adsorption. Furthermore, the value of 1/n in the three ranges of 0 < 1/n < 1, 1/n = 0 and 1/n > 1 implies favorable, unfavorable and irreversible adsorption, respectively.50 Thus, it can be predicted that the adsorption behavior was favorable and spontaneous according to the fitting results.
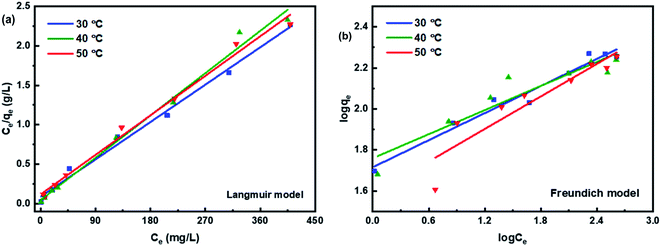 |
| Fig. 10 Adsorption isotherms from (a) Langmuir and (b) Freundlich isotherm models for the adsorption of Pb2+ on the L-cyst-ACF composite. Conditions: [adsorbent] = 0.5 g L−1 [metal] = 100 mg L−1, contact time = 6 h, and pH = 5.5. | |
Table 2 The parameters of the isotherm models
Isotherm models |
Parameters |
303 K |
313 K |
323 K |
Langmuir model |
qmax (mg g−1) |
161.03 |
167.78 |
179.53 |
KL (L mg−1) |
0.1021 |
0.1342 |
0.0481 |
R2 |
0.992 |
0.991 |
0.991 |
Freundlich model |
KF (L mg−1) |
51.9481 |
57.4619 |
38.4964 |
1/n |
0.2201 |
0.196 |
0.2644 |
R2 |
0.967 |
0.883 |
0.857 |
Table 3 The comparison of the effect of different adsorption materials
Adsorbent |
Fitting model |
qmax mg g−1 |
Ref. |
Sulfur-ferromagnetic nanoparticles |
Langmuir |
66.45 |
51 |
Chitosan-gelatin aerogels |
Langmuir |
11.1 |
52 |
Agro waste biochar |
Freundlich |
119.8 |
53 |
Microwave-assisted rice straw activated carbon |
Langmuir |
152.39 |
54 |
Nonwoven polyethylene-coated polypropylene fiber |
Langmuir |
63.36 |
55 |
Activated carbon nanofiber |
Freundlich |
120.3 |
56 |
Nitrogen-doped carbon nanofibers |
Langmuir |
88 |
57 |
L-Cysteine-modified activated carbon fiber |
Langmuir |
179.53 |
This study |
3.6 Adsorption thermodynamics
The standard Gibbs free energy change (ΔGθ), entropy change (ΔSθ) and enthalpy change (ΔHθ) were calculated using eqn (9) and (10) to recognize the energetics and spontaneity involved in the procedure for the combination of Pb2+ and the adsorbent. |
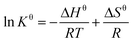 | (9) |
where Kθ represents the constant associated with the thermodynamic equilibrium, which is replaced by KL from the Langmuir fitting.43 R and T are the universal gas constant [8.314 J (mol−1 K−1)] and the temperature, respectively.13
The thermodynamic parameters of adsorption provide an effective method for in-depth understanding of the nature of the adsorption process. The changes on Gibbs free energy (ΔG), enthalpy (ΔH), and entropy (ΔS) values during Pb2+ adsorption was given in Table 4. Accordingly, the calculated adsorption enthalpy and entropy for of Pb2+ adsorption were positive, which indicated that the affinity between metal ions and the material surface was sufficient to make it adhere, and the randomness of the solid-liquid interface increased during this process. Otherwise, negative ΔG value showed the feasibility and spontaneity of the adsorption process, indicating endothermic process which was also predicted before. The endothermic nature of adsorption could be attributed to the increasing temperature that accelerated the diffusion of Pb2+ and the increase of system disorder, which lead to the positive progress of adsorption.
Table 4 Thermodynamic parameters for the adsorption of Pb2+
Parameter |
298 K |
308 K |
318 K |
ΔGθ (kJ mol−1) |
−5.75 |
−5.23 |
−8.15 |
ΔHθ (kJ·mol−1) |
|
18.83 |
|
ΔSθ (J mol−1 K−1) |
|
43.56 |
|
3.7 Adsorption mechanism
The adsorption of metal ions on the surface of carbon materials is primarily related to their pore structure and surface groups. According to Fig. 11, the XPS spectra of L-cyst-ACF before and after adsorbing were recorded to gain insight into the mechanism of action between Pb2+ and L-cyst-ACF. The peak positions of Pb 4f 7/2 and Pb 4f 5/2 were detected at 143.78 and 139.03 eV, respectively, which indicate the successful adsorption of Pb2+.58 As shown in Fig. 11c, the binding energies of C 1s appeared at 284.74, 286.02 and 287.16 eV, which are ascribed to the C–C, C–O, and C
O bonds, respectively.59 Subsequently, the peaks located at 284.63 and 285.35 eV are assigned to C–C and C–O, respectively, suggesting that both bonds almost did not play a role in the adsorption process. Notably, the peak position at 287.16 eV shifted to a higher position at 288.33 eV, which illustrates that the adsorption depends on the C
O bond.
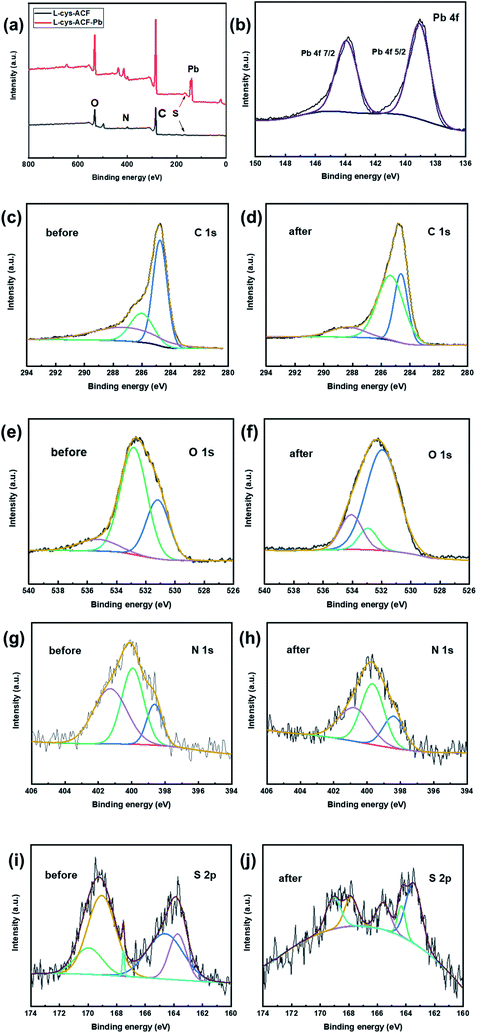 |
| Fig. 11 XPS spectra of (a) full survey (b) Pb 4f and (c and d) C 1s (e and f) O 1s (g and h) N 1s and (i and j) S 2p before and after adsorption, respectively. | |
The high-resolution XPS spectra of O 1s and N 1s before and after the adsorption of Pb2+ are showed in Fig. 11e–h. According to Fig. 11e, three peaks appeared at 531.17, 532.82, and 535.29 eV, which are attributed to the C–O, O
C–NH–, and O
C–O bonds, respectively.60 Then, it was clearly demonstrated that the bonding energy positions at 531.94 and 532.93 eV did not change, which suggests that the covalent bonds of C–O and O
C–NH– are not involved in the adsorption process. The peak at 535.29 eV shifted to 534.07 eV, which illustrates that the carboxyl group (O
C–O–) played a key role during the adsorption process. According to reports, a variety of carbonyl-containing oxygen functional groups, carboxylate and epoxide groups in the adsorbent structure act as electron donors, which lead to surface complexation with metal ions, contributing to the uptake of Pb2+.15,37,61
There are three main binding energy positions at 398.63, 399.94, and 401.28 eV in Fig. 11g, which correspond to –NH, C–N, and O
C–NH–, respectively.62 Obviously, these binding energy positions did not exhibit a visible change, as shown in Fig. 11h, which indicates that nitrogen-containing functional groups did not participate in the adsorption process. There are many chemical environments of S in the modified fiber material,26 such as S–S, C–S, –SH, C
S, and SO32−, which were accurately found at 163.74, 164.61, 167.53, 169.05, and 169.95 eV in Fig. 11i, respectively.26,27 As shown in Fig. 11j, the spectra of S–S and C–S could be fitted with two peaks at 163.45 and 164.31 eV, which represent that these two bonds did not participate in the adsorption of Pb2+. Surprisingly, the binding energy positions at 167.53, 169.05, and 169.95 shifted to lower positions, as shown in Fig. 11j (∼165.57 eV, ∼167.85 eV, and ∼169.08 eV), revealing that –SH, C
S, and SO32−, respectively, produced coordinated chelation and electrostatic attraction toward Pb2+. According to the hard soft acid base (HSAB) theory,63 a metal–ligand complex can be formed between Pb2+ (soft acid with empty track) and surface functional groups, such as –SH, O
C–O–, and C
S (soft base with free electrons) via covalent bonding. Therefore, the formation of metal–organic complexes greatly improved the adsorption properties of the material.
3.8 Reusability and cycling performance
It was very important to evaluate the cycling performance of L-cyst-ACF considering the cost of adsorbents used in practical production. Therefore, adsorption–desorption cycle experiments were carried out on L-cyst-ACF herein, focusing on the reusability and removal efficiency changes. EDTA-2Na salt solution with a concentration of 0.5 mol L−1 was used to desorb L-cyst-ACF saturated with adsorbed species in this study. The changes in the adsorption capacity and adsorption efficiency of the material after 5 adsorption–desorption cycles are summarized in Fig. 12. With an increase in the number of regeneration cycles, the adsorption of lead ions decreased from about 130 mg g−1 to only 80 mg g−1, indicating that the adsorption capacity of L-cyst-ACF for Pb2+ decreased after the fiber underwent multiple elution cycles. We speculated that the functional groups on the fiber surface were lost and some were oxidized and failed during repeated experimental operations. In general, L-cyst-ACF exhibited ideal reusability and has certain prospects in the treatment of lead pollution.
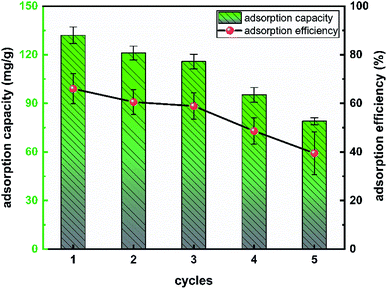 |
| Fig. 12 Reusability performance of L-cyst-ACF-adsorbed Pb2+. | |
4 Conclusion
An activated carbon fiber material grafted with L-cysteine was prepared via an amide reaction and the batch adsorption experiments showed that L-cyst-ACF was effective for the removal of Pb2+ from aqueous solution. Adsorption experiments were carried out to investigate different factors such as initial concentration, pH, adsorbent dosage, contact time, and temperature. The experimental results showed that the adsorption effect was better when the pH of the solution was between 5 and 6; a higher temperature was conducive to the adsorption process; the adsorption efficiency was the best when the dosage of adsorbent was 0.5 g L; the adsorption reached equilibrium within 6 h, and qe was 136.80 mg g−1. In addition, L-cyst-ACF had a certain cycling performance after multiple regeneration treatments.
Compared with the pseudo-first-order kinetic equation, it was concluded that the fitting of the pseudo-second-order kinetic equation was more suitable in the adsorption process, implying that the adsorption process was chemisorption. The data from the isothermal models showed that the Langmuir model fitted well, which indicated that the material adsorption process was monolayer adsorption. The thermodynamic analysis showed that ΔG was negative at all temperatures, which indicated that the adsorption process was endothermic and spontaneous. The positive ΔS indicated that the system disorder gradually increased during the adsorption process. Finally, the XPS analysis illustrated that abundant –SH, –COOH, and C
S groups on the fiber surface were the crucial factor for the significant improvement in the adsorption capacity.
Conflicts of interest
The authors declare no competing financial interest.
Acknowledgements
This work was supported by the Zhejiang Province Public Welfare Project (NO. LGG20E030009). The work was also supported by the Jiaxing Science and Technology Plan Project (NO. 2019AD32008).
References
- R. M. Ali, H. A. Hamad, M. M. Hussein and G. F. Malash, Potential of using green adsorbent of heavy metal removal from aqueous solutions: Adsorption kinetics, isotherm, thermodynamic, mechanism and economic analysis, Ecol. Eng., 2016, 91, 317–332 CrossRef.
- C. Men, R. Liu, F. Xu, Q. Wang, L. Guo and Z. Shen, Pollution characteristics, risk assessment, and source apportionment of heavy metals in road dust in Beijing, China, Sci. Total Environ., 2018, 612, 138–147 CrossRef CAS PubMed.
- X. Bi, Z. Li, G. Sun, J. Liu and Z. Han, In vitro bioaccessibility of lead in surface dust and implications for human exposure: A comparative study between industrial area and urban district, J. Hazard. Mater., 2015, 297, 191–197 CrossRef CAS PubMed.
- V. Matovic, A. Buha, D. Ethukic-Cosic and Z. Bulat, Insight into the oxidative stress induced by lead and/or cadmium in blood, liver and kidneys, Food Chem. Toxicol., 2015, 78, 130–140 CrossRef CAS PubMed.
- M. Thakur, S. Praveen, P. R. Divte, R. Mitra, M. Kumar and C. K. Gupta, et al. Metal tolerance in plants: Molecular and physicochemical interface determines the “not so heavy effect” of heavy metals, Chemosphere, 2022, 287(Pt 1), 131957 CrossRef CAS PubMed.
- M. Hanna-Attisha, J. LaChance, R. C. Sadler and A. Champney Schnepp, Elevated Blood Lead Levels in Children Associated With the Flint Drinking Water Crisis: A Spatial Analysis of Risk and Public Health Response, Am. J. Public Health, 2016, 106(2), 283–290 CrossRef PubMed.
- R. Alizadeh, R. K. Kazemi and M. R. Rezaei, Ultrafast removal of heavy metals by tin oxide nanowires as new adsorbents in solid-phase extraction technique, Int. J. Environ. Sci. Technol., 2017, 15(8), 1641–1648 CrossRef.
- M. Nemati, S. M. Hosseini, F. Parvizian, N. Rafiei and B. Van der Bruggen, Desalination and heavy metal ion removal from water by new ion exchange membrane modified by synthesized NiFe2O4/HAMPS nanocomposite, Ionics, 2019, 25(8), 3847–3857 CrossRef CAS.
- M. C. Benalia, L. Youcef, M. G. Bouaziz, S. Achour and H. Menasra, Removal of Heavy Metals from Industrial Wastewater by Chemical Precipitation: Mechanisms and Sludge Characterization, Arabian J. Sci. Eng., 2022, 47(5), 5587–5599 CrossRef CAS.
- H. Alijani, M. H. Beyki, Z. Shariatinia, M. Bayat and F. Shemirani, A new approach for one step synthesis of magnetic carbon nanotubes/diatomite earth composite by chemical vapor deposition method: Application for removal of lead ions, Chem. Eng. J., 2014, 253, 456–463 CrossRef CAS.
- Z.-f. Cao, X. Wen, J. Wang, F. Yang, H. Zhong and S. Wang, et al. In situ nano-Fe3O4/triisopropanolamine functionalized graphene oxide composites to enhance Pb2+ ions removal, Colloids Surf., A, 2019, 561, 209–217 CrossRef CAS.
- J. Qu, Y. Liu, L. Cheng, Z. Jiang, G. Zhang and F. Deng, et al. Green synthesis of hydrophilic activated carbon supported sulfide nZVI for enhanced Pb(II) scavenging from water: Characterization, kinetics, isotherms and mechanisms, J. Hazard. Mater., 2021, 403, 123607 CrossRef CAS PubMed.
- E. Santoso, R. Ediati, Y. Kusumawati, H. Bahruji, D. O. Sulistiono and D. Prasetyoko, Review on recent advances of carbon based adsorbent for methylene blue removal from waste water, Mater. Today Chem., 2020, 16, 100233 CrossRef CAS.
- Y. Yang, Y. Xie, L. Pang, M. Li, X. Song and J. Wen, et al. Preparation of reduced graphene oxide/poly(acrylamide) nanocomposite and its adsorption of Pb(II) and methylene blue, Langmuir, 2013, 29(34), 10727–10736 CrossRef CAS PubMed.
- L. X. Zhang, S. Y. Tang, F. X. He, Y. Liu, W. Mao and Y. T. Guan, Highly efficient and selective capture of heavy metals by poly(acrylic acid) grafted chitosan and biochar composite for wastewater treatment, Chem. Eng. J., 2019, 378, 122215 CrossRef CAS.
- A. A. Alqadami, M. Naushad, A. L. ZA, M. Alsuhybani and M. Algamdi, Excellent adsorptive performance of a new nanocomposite for removal of toxic Pb(II) from aqueous environment: Adsorption mechanism and modeling analysis, J. Hazard. Mater., 2020, 389, 121896 CrossRef CAS PubMed.
- P. S. Carraro, L. Spessato, L. H. S. Crespo, J. T. C. Yokoyama, J. M. Fonseca and K. C. Bedin, et al. Activated carbon fibers prepared from cellulose and polyester–derived residues and their application on removal of Pb2+ ions from aqueous solution, J. Mol. Liq., 2019, 289, 111150 CrossRef CAS.
- S. Deng, P. Wang, G. Zhang and Y. Dou, Polyacrylonitrile-based fiber modified with thiosemicarbazide by microwave irradiation and its adsorption behavior for Cd(II) and Pb(II), J. Hazard. Mater., 2016, 307, 64–72 CrossRef CAS PubMed.
- S. He, C. Lu and S. Zhang, Facile and efficient route to polyimide-TiO2 nanocomposite coating onto carbon fiber, ACS Appl. Mater. Interfaces, 2011, 3(12), 4744–4750 CrossRef CAS PubMed.
- J. A. Arcibar-Orozco, J. R. Rangel-Mendez and P. E. Diaz-Flores, Simultaneous Adsorption of Pb(Ii)-Cd(Ii), Pb(Ii)-Phenol, and Cd(Ii)-Phenol by Activated Carbon Cloth in Aqueous Solution, Water, Air, Soil Pollut., 2014, 226(1), 2197 CrossRef.
- N. Wang, Y. Qiu, T. Xiao, J. Wang, Y. Chen and X. Xu, et al. Comparative studies on Pb(II) biosorption with three spongy microbe-based biosorbents: High performance, selectivity and application, J. Hazard. Mater., 2019, 373, 39–49 CrossRef CAS PubMed.
- G. Xu, Y. J. Xie, J. Cao, M. L. Tao and W. Q. Zhang, Highly selective and efficient chelating fiber functionalized by bis(2-pyridylmethyl)amino group for heavy metal ions, Polym. Chem., 2016, 7(23), 3874–3883 RSC.
- L. Sharma and R. Kakkar, Hierarchical Porous Magnesium Oxide (Hr-MgO) Microspheres for Adsorption of an Organophosphate Pesticide: Kinetics, Isotherm, Thermodynamics, and DFT Studies, ACS Appl. Mater. Interfaces, 2017, 9(44), 38629–38642 CrossRef CAS PubMed.
- G. B. Adebayo, H. I. Adegoke and S. Fauzeeyat, Adsorption of Cr(VI) ions onto goethite, activated carbon and their composite: kinetic and thermodynamic studies, Appl. Water Sci., 2020, 10(9), 213 CrossRef CAS.
- Q. Jiang, W. Xie, S. Han, Y. Wang and Y. Zhang, Enhanced adsorption of Pb(II) onto modified hydrochar by polyethyleneimine or H3PO4: An analysis of surface property and interface mechanism, Colloids Surf., A, 2019, 583 Search PubMed.
- S. Pan, Y. Zhang, H. Shen and M. Hu, An intensive study on the magnetic effect of mercapto-functionalized nano-magnetic Fe3O4 polymers and their adsorption mechanism for the removal of Hg(II) from aqueous solution, Chem. Eng. J., 2012, 210, 564–574 CrossRef CAS.
- P. L. Yap, Y. L. Auyoong, K. Hassan, F. Farivar, D. N. H. Tran and J. Ma, et al. Multithiol functionalized graphene bio-sponge via photoinitiated thiol-ene click chemistry for efficient heavy metal ions adsorption, Chem. Eng. J., 2020, 395, 124965 CrossRef CAS.
- Y. Huang, Y. Gong, J. Tang and S. Xia, Effective removal of inorganic mercury and methylmercury from aqueous solution using novel thiol-functionalized graphene oxide/Fe-Mn composite, J. Hazard. Mater., 2019, 366, 130–139 CrossRef CAS PubMed.
- K. M. Mena Aguilar, Y. Amano and M. Machida, Ammonium persulfate oxidized activated carbon fiber as a high capacity adsorbent for aqueous Pb(II), J. Environ. Chem. Eng., 2016, 4(4), 4644–4652 CrossRef CAS.
- J. Mao, M. Ge, J. Huang, Y. Lai, C. Lin and K. Zhang, et al. Constructing multifunctional MOF@rGO hydro-/aerogels by the self-assembly process for customized water remediation, J. Mater. Chem. A, 2017, 5(23), 11873–11881 RSC.
- X. Yang, Y. Wan, Y. Zheng, F. He, Z. Yu and J. Huang, et al. Surface functional groups of carbon-based adsorbents and their roles in the removal of heavy metals from aqueous solutions: A critical review, Chem. Eng. J., 2019, 366, 608–621 CrossRef CAS PubMed.
- L. M. Cui, Y. G. Wang, L. Gao, L. H. Hu, L. G. Yan and Q. Wei, et al. EDTA functionalized magnetic graphene oxide for removal of Pb(II), Hg(II) and Cu(II) in water treatment: Adsorption mechanism and separation property, Chem. Eng. J., 2015, 281, 1–10 CrossRef CAS.
- C. J. Madadrang, H. Y. Kim, G. Gao, N. Wang, J. Zhu and H. Feng, et al. Adsorption behavior of EDTA-graphene oxide for Pb (II) removal, ACS Appl. Mater. Interfaces, 2012, 4(3), 1186–1193 CrossRef CAS PubMed.
- K. G. Sreejalekshmi, K. A. Krishnan and T. S. Anirudhan, Adsorption of Pb(II) and Pb(II)-citric acid on sawdust activated carbon: Kinetic and equilibrium isotherm studies, J. Hazard. Mater., 2009, 161(2–3), 1506–1513 CrossRef CAS PubMed.
- N. N. Wang, X. J. Xu, H. Y. Li, J. L. Zhai, L. Z. Yuan and K. X. Zhang, et al. Preparation and Application of a Xanthate-Modified Thiourea Chitosan Sponge for the Removal of Pb(II) from Aqueous Solutions, Ind. Eng. Chem. Res., 2016, 55(17), 4960–4968 CrossRef CAS.
- P. L. Yap, K. Hassan, Y. L. Auyoong, N. Mansouri, F. Farivar and D. N. H. Tran, et al. All-in-One Bioinspired Multifunctional Graphene Biopolymer Foam for Simultaneous Removal of Multiple Water Pollutants, Adv. Mater. Interfaces, 2020, 7(18), 2000664 CrossRef CAS.
- L. Zhou, L. Ji, P. C. Ma, Y. Shao, H. Zhang and W. Gao, et al. Development of carbon nanotubes/CoFe2O4 magnetic hybrid material for removal of tetrabromobisphenol A and Pb(II), J. Hazard. Mater., 2014, 265, 104–114 CrossRef CAS PubMed.
- L. Zhang, W. Li, H. Cao, D. Hu, X. Chen and Y. Guan, et al. Ultra-efficient sorption of Cu(2+) and Pb(2+) ions by light biochar derived from Medulla tetrapanacis, Bioresour. Technol., 2019, 291, 121818 CrossRef CAS PubMed.
- W. Rudzinski and W. Plazinski, Kinetics of dyes adsorption at the solid-solution interfaces: a theoretical description based on the two-step kinetic model, Environ. Sci. Technol., 2008, 42(7), 2470–2475 CrossRef CAS PubMed.
- J. Wang and X. Guo, Adsorption kinetic models: Physical meanings, applications, and solving methods, J. Hazard. Mater., 2020, 390, 122156 CrossRef CAS PubMed.
- J. Ai, F. Y. Chen, C. Y. Gao, H. R. Tian, Q. J. Pan and Z. M. Sun, Porous Anionic Uranyl-Organic Networks for Highly Efficient Cs(+) Adsorption and Investigation of the Mechanism, Inorg. Chem., 2018, 57(8), 4419–4426 CrossRef CAS PubMed.
- S. Kim, C. M. Park, M. Jang, A. Son, N. Her and M. Yu, et al. Aqueous removal of inorganic and organic contaminants by graphene-based nanoadsorbents: A review, Chemosphere, 2018, 212, 1104–1124 CrossRef CAS PubMed.
- M. A. Al-Ghouti and D. A. Da'ana, Guidelines for the use and interpretation of adsorption isotherm models: A review, J. Hazard. Mater., 2020, 393, 122383 CrossRef CAS PubMed.
- Z. Liu, X. Zhong, Y. Wang, Z. Ding, C. Wang and G. Wang, et al. An Efficient Adsorption of Manganese Oxides/Activated Carbon Composite for Lead(II) Ions from Aqueous Solution, Arabian J. Sci. Eng., 2017, 43(5), 2155–2165 CrossRef.
- G. Özsin, M. Kılıç, E. Apaydın-Varol and A. E. Pütün, Chemically activated carbon production from agricultural waste of chickpea and its application for heavy metal adsorption: equilibrium, kinetic, and thermodynamic studies, Appl. Water Sci., 2019, 9(3), 56 CrossRef.
- J. Wang and X. Guo, Adsorption isotherm models: Classification, physical meaning, application and solving method, Chemosphere, 2020, 258, 127279 CrossRef CAS PubMed.
- S. M. Khadivi, L. Edjlali, A. Akbarzadeh and K. Seyyedi, Enhanced adsorption behavior of amended EDTA-graphene oxide for methylene blue and heavy metal ions, Int. J. Environ. Sci. Technol., 2019, 16(12), 8151–8160 CrossRef CAS.
- M. Rethinasabapathy, S.-M. Kang, I. Lee, G.-W. Lee, S. K. Hwang and C. Roh, et al. Layer-Structured POSS-Modified Fe-Aminoclay/Carboxymethyl Cellulose Composite as a Superior Adsorbent for the Removal of Radioactive Cesium and Cationic Dyes, Ind. Eng. Chem. Res., 2018, 57(41), 13731–13741 CrossRef CAS.
- T. Bohli, A. Ouederni and I. Villaescusa, Simultaneous adsorption behavior of heavy metals onto microporous olive stones activated carbon: analysis of metal interactions, Euro-Mediterr. j. environ. integr., 2017, 2(1), 19 CrossRef.
- S. Malamis and E. Katsou, A review on zinc and nickel adsorption on natural and modified zeolite, bentonite and vermiculite: examination of process parameters, kinetics and isotherms, J. Hazard. Mater., 2013, 252–253, 428–461 CrossRef CAS PubMed.
- H. Song, A. Kumar and Y. Zhang, A novel approach for the removal of Pb(2+) and Cd(2+) from wastewater by sulfur-ferromagnetic nanoparticles (SFMNs), Chemosphere, 2022, 287(Pt 2), 132156 CrossRef CAS PubMed.
- A. Kovtun, E. Campodoni, L. Favaretto, M. Zambianchi, A. Salatino and S. Amalfitano, et al. Multifunctional graphene oxide/biopolymer composite aerogels for microcontaminants removal from drinking water, Chemosphere, 2020, 259, 127501 CrossRef CAS.
- R. Gayathri, K. P. Gopinath and P. S. Kumar, Adsorptive separation of toxic metals from aquatic environment using agro waste biochar: Application in electroplating industrial wastewater, Chemosphere, 2021, 262, 128031 CrossRef CAS PubMed.
- Z. Du, H. Chen, X. Guo, L. Qin, D. Lin and L. Huo, et al. Mechanism and industrial application feasibility analysis on microwave-assisted rapid synthesis of amino-carboxyl functionalized cellulose for enhanced heavy metal removal, Chemosphere, 2021, 268, 128833 CrossRef CAS PubMed.
- F. Özmen, S. Korpayev, P. A. Kavaklı and C. Kavaklı, Activation of inert polyethylene/polypropylene nonwoven fiber (NWF) by plasma-initiated grafting and amine functionalization of the grafts for Cu(II), Co(II), Cr(III), Cd(II) and Pb(II) removal, React. Funct. Polym., 2022, 174 Search PubMed.
- N. Abdullah, F. E. C. Othman, N. Yusof, T. Matsuura, W. J. Lau and J. Jaafar, et al. Preparation of nanocomposite activated carbon nanofiber/manganese oxide and its adsorptive performance toward leads (II) from aqueous solution, J. Water Process. Eng., 2020, 37, 101430 CrossRef.
- A. Modi, B. Bhaduri and N. Verma, Facile One-Step Synthesis of Nitrogen-Doped Carbon Nanofibers for the Removal of Potentially Toxic Metals from Water, Ind. Eng. Chem. Res., 2015, 54(18), 5172–5178 CrossRef CAS.
- W. Czepa, D. Pakulski, S. Witomska, V. Patroniak, A. Ciesielski and P. Samori, Graphene oxide-mesoporous SiO2 hybrid composite for fast and efficient removal of organic cationic contaminants, Carbon, 2020, 158, 193–201 CrossRef CAS.
- F. Cao and J. Shen, PEI-Modified CMKGM/GO Porous Biocomposite for Superior Removal of Pb(II), J. Chem. Eng. Data, 2019, 64(12), 5622–5629 CrossRef CAS.
- H. Wang, X. Yuan, Y. Wu, X. Chen, L. Leng and H. Wang, et al. Facile synthesis of polypyrrole decorated reduced graphene oxide–Fe3O4 magnetic composites and its application for the Cr(VI) removal, Chem. Eng. J., 2015, 262, 597–606 CrossRef CAS.
- J. Kolarik, A. Bakandritsos, Z. Bad'ura, R. Lo, G. Zoppellaro and S. Kment, et al. Carboxylated Graphene for Radical-Assisted Ultra-Trace-Level Water Treatment and Noble Metal Recovery, ACS Nano, 2021, 15(2), 3349–3358 CrossRef CAS PubMed.
- Y. H. Liao, Y. Q. Wang, X. F. Zhu and G. D. Ji, Amide-functionalized graphene-assembled monoliths with high adsorption capacity of Cd2+, Environ. Technol. Innovation, 2021, 23, 00228-5 Search PubMed.
- T.-L. Ho, Hard soft acids bases (HSAB) principle and organic chemistry, Chem. Rev., 2002, 75(1), 1–20 CrossRef.
|
This journal is © The Royal Society of Chemistry 2022 |