DOI:
10.1039/D2RA01250B
(Review Article)
RSC Adv., 2022,
12, 20360-20378
Synchrotron radiation based X-ray techniques for analysis of cathodes in Li rechargeable batteries†
Received
24th February 2022
, Accepted 15th June 2022
First published on 13th July 2022
Abstract
Li-ion rechargeable batteries are promising systems for large-scale energy storage solutions. Understanding the electrochemical process in the cathodes of these batteries using suitable techniques is one of the crucial steps for developing them as next-generation energy storage devices. Due to the broad energy range, synchrotron X-ray techniques provide a better option for characterizing the cathodes compared to the conventional laboratory-scale characterization instruments. This work gives an overview of various synchrotron radiation techniques for analyzing cathodes of Li-rechargeable batteries by depicting instrumental details of X-ray diffraction, X-ray absorption spectroscopy, X-ray imaging, and X-ray near-edge fine structure-imaging. Analysis and simulation procedures to get appropriate information of structural order, local electronic/atomic structure, chemical phase mapping and pores in cathodes are discussed by taking examples of various cathode materials. Applications of these synchrotron techniques are also explored to investigate oxidation state, metal–oxygen hybridization, quantitative local atomic structure, Ni oxidation phase and pore distribution in Ni-rich layered oxide cathodes.
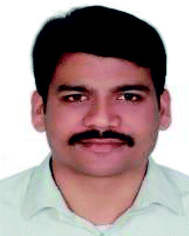 Jitendra Pal Singh | Jitendra Pal Singh is currently working at Manav Rachna University as a Ramanujan Fellow. He received Ph.D. from Govind Ballabh Pant University of Agriculture and Technology, Pantnagar, Uttarakhand in 2010. He worked as a Research Associate at Inter-University Accelerator Centre, New Delhi, during 2010–2011 and as a post-doc researcher at Taiwan SPIN Research Centre, National Chung Cheng University, Taiwan, during 2011–2012. He was an Assistant Professor at Krishna Engineering College, Ghaziabad, India, from 2012 to 2014. In 2014, he joined the Korea Institute of Science and Technology, Korea. He moved to Pohang Accelerator Laboratory, Pohang, South Korea and worked there from December 2018 to May 2022. His research interests are irradiation studies in nanoferrites, thin films, magnetic multilayers, biomaterials and cathode materials. He has expertise in X-ray absorption spectroscopy and hard X-ray imaging. Dr Singh is a member of Magnetic Society of India, Indian Physics Association, and Ion Beam Society of India. |
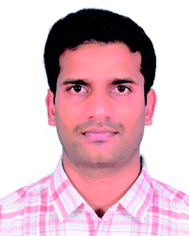 Anil Kumar Paidi | Anil K. Paidi earned his Ph.D. in Solid State Chemistry, Inorganic Chemistry Division under Prof. K. Vidyasagar from the Indian Institute of Technology Madras, India. Subsequently, he performed postdoctoral work under Prof. A. Mukhopadhyay's group (Indian Institute of Technology Bombay, India). Dr Paidi is currently a postdoctoral researcher, working with Dr Dochean Ahn and Dr Sangsul Lee at Pohang Accelerator Laboratory, South Korea. His research primarily focuses on understanding the chemistry that is relevant to energy storage materials. He is currently working on nickel-rich layered transition metal oxide compositions for high-capacity cathodes. |
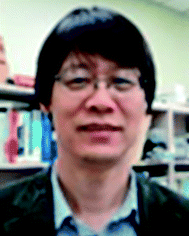 Keun Hwa Chae | Keun Hwa Chae received a B.Sc. in physics in 1986 and an M.Sc. in Physics in 1988 from Yonsei University, Seoul, Korea. In 1994, he received Ph.D. Degree in Physics from Yonsei University, Seoul, Korea. He was a postdoctoral fellow from 1995-97 at Rutgers-The State University of New Jersey, USA. His research fields are ion beam modification of materials and characterization of materials using synchrotron radiation. He has been working as a principal research scientist in Korea Institute of Science and Technology, Korea, since 2000. |
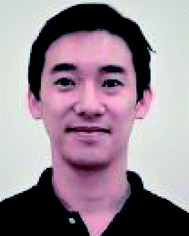 Sangsul Lee | Sangsul Lee is the head of the PAL-EUV metrology and inspection at Pohang Accelerator Laboratory, POSTECH. He is also the chief technology officer of Xavisoptics, an X-ray solution, and equipment company. He received Ph.D. in materials science and engineering from Hanyang University in 2012. His research interest is synchrotron-based nanoscale imaging such as EUV metrology, inspection, and nanoscale tomography. |
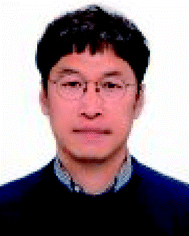 Docheon Ahn | Docheon Ahn is a senior scientist and manager of 9B high resolution powder diffraction beamline at Pohang Accelerator Laboratory (PAL). He received his Ph.D. in materials science & engineering from Pohang University of Science and Technology (POSTECH) in 2010. He was a visiting scientist at Advanced Photon Source (APS) of Argonne National Laboratory (ANL) in 2017. His primary research focuses on crystal structural analysis using X-ray powder diffraction and development of electrode materials for rechargeable batteries. He is the author of more than 100 peer-reviewed papers. |
Introduction
In recent years, the development of electrochemical devices with improved performance has received significant attention for fulfilling the increased energy demand of mankind.1,2 This necessity leaves researchers to optimize these devices in terms of cost-effectiveness and energy consumption.3–5 A battery is a fine example of an electrochemical energy device that stores chemical energy and converts it into electrical energy. The energy conversion takes place due to the occurrence of redox reactions at the anode and cathode.6 Thus, a typical battery consists of an anode and a cathode separated by an electrolyte (Fig. 1a and b).6,7
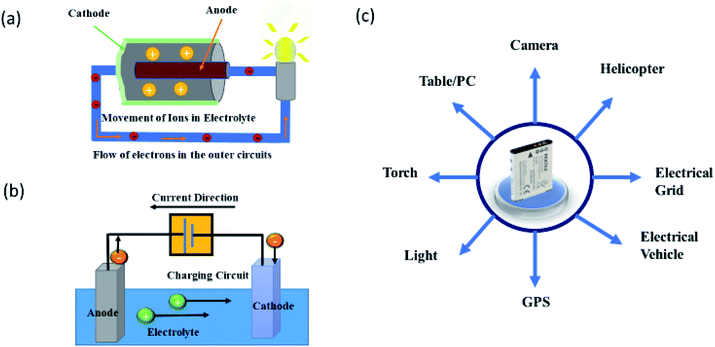 |
| Fig. 1 (a) Primary battery showing the electricity generation through the movement of electrons via electrolyte. Adapted from https://www.explainthatstuff.com/batteries.html. (b) Schematic of rechargeable batteries. Adapted from https://www.matsusada.com/column/battery_chaege-dischaege.html. (c) Applications of rechargeable battery. Adapted from https://www.lithiumbatterychina.com/blog/2019/03/21/how-to-find-the-right-custom-lithium-ion-battery-packs-manufacturers-from-china-for-electronic-devices/ | |
Fig. 1a shows a schematic of the primary battery which contains an immersed anode in the electrolyte surrounded by the cathode. In this case, electrical energy is produced by the movement of ions in the electrolyte. This kind of battery is also known as a primary battery as it is usually discarded after use.8,9 Thus, commercial use of this battery is limited to household appliances.10
Another battery that is based on the same phenomenon but can be charged again after being discharged is known as a rechargeable or secondary battery (Fig. 1b).11–13 The term “accumulator” is also used for this battery as it accumulates and stores energy through a reversible electrochemical reaction. This battery can be re-used after discharging which makes it suitable for long term use. Thus, it is utilized for numerous appliances – ranging from portable devices14,15 to electrical vehicles (EV)16–19 (Fig. 1c), thereby helping to fulfill the increased energy demands of mankind.
Rechargeable batteries are designed and named based on several combinations of electrodes and electrolytes. Some of these batteries are lead–acid,20 nickel–cadmium (NiCd),21 nickel–metal hydride (NiMH),22 lithium-ion (Li-ion),23 and lithium-ion polymer (Li-ion polymer).24 Li-ion rechargeable batteries (LIB)25,26 received significant attention from the scientific community in recent years owing to superior electrochemical performance.27–29
Li-ion rechargeable batteries (LIB)
LIB is a type of rechargeable battery in which lithium ions (Li+) move from the cathode to anode during charging and vice versa when discharging. As can be seen from the Ragone plot of energy devices, the performance of LIBs is just below the traditional combustion engine (Fig. 2a).30,31 This plot also informs that LIBs have better energy density and power density compared to not only to other rechargeable batteries but also to another sources of electricity storage such as capacitors. This makes these batteries prominent candidates to use them as fundamental devices for sustainable energy development.32–34
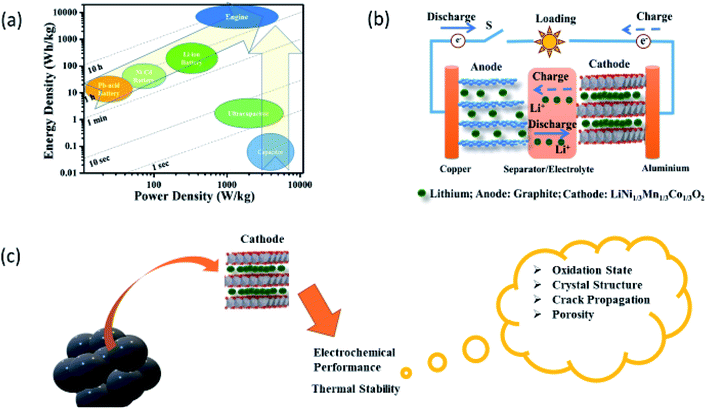 |
| Fig. 2 (a) Ragone plot for energy storage devices and traditional internal-combustion engine. Times shown are the time constants of the devices, obtained by dividing the energy density by the power density. Reproduced from ref. 30 with permission from [Springer Nature], copyright [2021]. (b) Schematic of a typical Li-rechargeable battery and it's various components. Reproduced from ref. 35 with permission from [Elsevier], copyright [2013]. (c) Issues that need attention in cathodes for the improved electrochemical performance of Li-rechargeable battery. | |
A typical LIB consists of a cathode composed of Li ions as constituents such as LiNi1/3Co1/3Mn1/3O2 (NCM333) and an anode (example, graphite) separated by an electrolyte along with a separator (Fig. 2b).35,36 Efforts to develop commercially successful LIBs for EVs37,38 and large-scale grid storages are under process.39,40 This reflects from the researchers' keen interest in understanding electrochemistry of LIBs by varying cathodes38,41–44 and anodes38,44,45 with the impression that electrochemistry plays a key role in the quest for better performance of electrochemical energy storage devices.46 Some of these anodes which are investigated so far are Li metal,47 Si nanoparticles,48 and NiFeOPO4/C anodes.49,50
The electrochemical behavior of Li-rechargeable batteries is extensively studied, focusing on the layered structured cathodes and their compatibility with anodes along with chemical intercalation process to produce high-energy power density.51–55 One condition for cathode is that they should provide sufficient lattice sites to store and release Li-ions, while maintaining stable cyclability and high specific capacity. Thus, numerous cathodes are developed owing to this concept. Crystal structure, specific capacity, volume capacity and average voltage of a few important cathodes are shown in Table 1.11,35,36,41 It can be seen clearly seen, that cathodes based on nickel cobalt manganese (NCM) oxide are more suitable56,57 because of high specific and volume capacity.11,42–44
Table 1 Characteristics of representative intercalation cathode compounds; crystal structure, theoretical/experimental/commercial gravimetric and volumetric capacities, average potentials and level of development. Reproduced from ref. 36 with permission from [Elsevier], copyright [2015]
Crystal structure |
Materials |
Theoretical/experimental |
Average voltage |
Ref. |
Specific capacity (mA h g−1) |
Volume capacity (mA h cm−3) |
Layered |
LiTiS2 |
225/210 |
697 |
1.9 |
58 |
LiCoO2 |
274/148 |
1363/550 |
3.8 |
59 |
LiNiO2 |
275/150 |
1280 |
3.8 |
60 |
LiMnO2 |
285/140 |
1148 |
3.3 |
61 |
LiNi0.33Mn0.33Co0.33O2 |
280/160 |
1333/600 |
3.7 |
62 |
LiNi0.8Mn0.15Al0.05O2 |
279/199 |
1284/700 |
3.7 |
63 |
Li2Mn2O3 |
458/180 |
1708 |
3.8 |
64 |
Spinel |
LiMn2O4 |
148/120 |
596 |
4.1 |
65 |
LiCo2O4 |
142/84 |
704 |
4.0 |
66 |
Olivine |
LiFePO4 |
170/165 |
589 |
3.4 |
67 |
LiMnPO4 |
171/168 |
567 |
3.8 |
68 |
LiCoPO4 |
167/125 |
510 |
4.2 |
69 |
Tavorite |
LiFeSO4 |
151/120 |
487 |
3.7 |
70 |
LiVPO4 |
156/129 |
484 |
4.2 |
71 |
NCM oxides exhibit layered structure, which is suitable for the de-lithiation and lithiation process.72,73 In the early days, these oxides having Ni, Co, and Mn ratio of 1
:
1
:
1 (i.e., NCM333) were extensively studied.74–76 Later, it is reported that excess of Ni significantly affects the electrochemical performance.77–79 Recent reports reveal that Ni-rich cathodes (LiNixMnyCo1−x−yO2; X ≥ 0.9)80–83 are better choice among other NCM compositions because of their high cumulative capacity. However, capacity retention is an issue in these cathodes which affects the overall electrochemical performance of LIBs. Besides the electrochemical performance, the thermal stability of cathode material (Fig. 2c) is another important parameter that needs to be taken care of while designing a cathode.84–86 These aspects in Ni-rich layered oxides are currently receiving significant attention and providing ample scope to the researchers of different communities for carrying out research activities in this direction. Synchrotron community is making a significant impact by solving various issues in cathodes and developing advanced techniques to achieve these objectives. Here, we summarize the recent progress by this community in the context of Ni-rich cathodes by giving an introduction to various synchrotron-assisted techniques.
Synchrotron radiation is an electromagnetic radiation emitted when relativistic charged particles are accelerated radially.87,88 This radiation is produced artificially in accelerators based on storage ring89,90 by using bending magnets,91 undulators and/or wigglers.92 Main characteristics of this radiation are polarization, and the presence of wavelengths over the entire electromagnetic spectrum.87–93 It also exhibits high brilliance, a high level of polarization (linear, elliptical, or circular), and high collimation.94,95 Low emittance, wide tunability in energy/wavelength by monochromatizating (sub-eV up to the MeV range), and pulsed light emission. These characteristics make this radiation more suitable for studying the material behavior compared to other radiations.96,97 A typical synchrotron radiation source consists of the following parts – (1) linear accelerator, (2) storage ring, and (3) beamline (Fig. 3). This radiation is allowed to travel towards the experimental end station by using beamlines.98
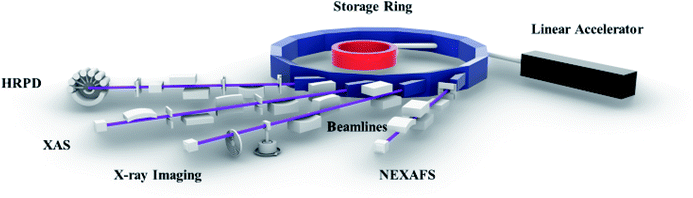 |
| Fig. 3 Various parts of synchrotron radiation source along with schematic of high-resolution powder diffraction (HRPD), X-ray absorption spectroscopy (XAS), X-ray imaging, and near edge X-ray absorption fine structure spectroscopy (NEXAFS) beamlines. | |
Several facilities are established worldwide to produce synchrotron radiation in a wide range of energy, i.e., 100 eV to 50 keV.99,100 These facilities consist of several characterization techniques such as X-ray photoelectron spectroscopy (XPS) and X-ray absorption spectroscopy (XAS).101 XPS gives chemical information of elements in the materials.102,103 XAS is able to probe the local atomic structure along with revealing the oxidation state of core atoms.104–107 High-resolution photoemission spectroscopy (HRPS) is efficient in examining the band structure.108 X-ray magnetic circular dichroism (XMCD) provides an opportunity to get insights into the local magnetic structure.109,110 X-ray reflectivity (XRR) and X-ray scattering (XRS) are used for elucidating dynamics of ordered and disordered materials.111 Even though depicting almost all aspects of materials, synchrotron techniques could not depict information of spatially resolved characteristics until the early years of this century.101,104,108,109 Thus, this drawback is overcome by utilizing spatially resolved accessories.112 XRD-imaging,113 XANES-imaging,114 and magnetic-imaging115 are the results of such efforts. These techniques can provide chemical and structural information of individual clusters of specific sizes decided by spatial resolution of particular facility.116,117 Thus, this review article gives an overview of XRD, XAS and XANES-imaging technique that are used for assessment of cathodes.118–123 Apart from this, X-ray imaging is too included in this study.124,125 A concise discussion of the phenomena investigated using these techniques is also elaborated.
Structural information
Synchrotron-based XRD provides a better way to understand the structural behavior of materials.126–130 The optical layout of high-resolution powder diffraction (HRPD) beamline is shown in Fig. 4a.128 The incident X-rays are vertically collimated by a mirror and monochromatized using a double-crystal Si(111) monochromator. The detector arm of the vertical scan diffractometer is composed of seven sets of Soller slits, flat Ge(111) crystal analyzers, anti-scatter baffles, and scintillation detectors, with each set separated by 20°. Fig. 4b shows the experimental procedure for in situ XRD of cathode. A coin-cell type battery with an electrode enabling measurement of XRD patterns at different stages of charging and discharging for the 1st cycle is designed. These patterns are recorded using a two-dimensional (2d) imaging plate and converted into a regular pattern by appropriate procedure.
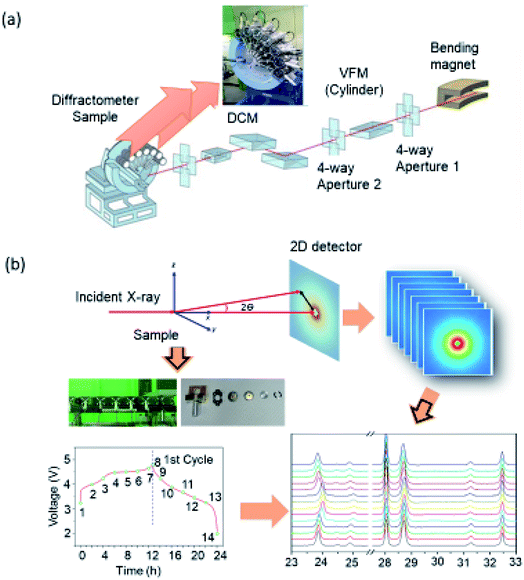 |
| Fig. 4 (a) Optical layout of a typical high-resolution powder diffraction beamline along with multistage target holder. Adapted from https://pal.postech.ac.kr/bl/9B/(PLS)II. (b) Experimental procedure for performing in situ XRD along with the sample holder, electrochemical curve and HRPD patterns at various states of charging and discharging. | |
The Rietveld refinement of the XRD pattern gives information of metal ion occupancy in the lattice of material under investigation.131,132 Several methods133,134 are developed to extract peak position, intensities, and width from XRD patterns for quantitative analysis of structural parameters. The most common powder XRD refinement technique is based on the method proposed in the 1960s by Hugo Rietveld.135
Fig. 5a shows the refined HRPD pattern of the LiNi0.87Co0.09Mn0.04O2 cathode using the Rietveld method. This pattern shows the presence of a layered structure. Estimated structural parameters are collated in Table 2. Due to better sensitivity, this technique is able to get insights into intermediate phases occurring during the growth of LiNi1/3Co1/3Mn1/3O2 cathode.136 In addition to this, these measurements are able to depict the surface nature of cathode. Patterns obtained from synchrotron X-ray reveal presence of NiO-like rock-salt phase by the reconstruction of surfaces in water washed LiNi0.88Co0.054Mn0.066O2.137
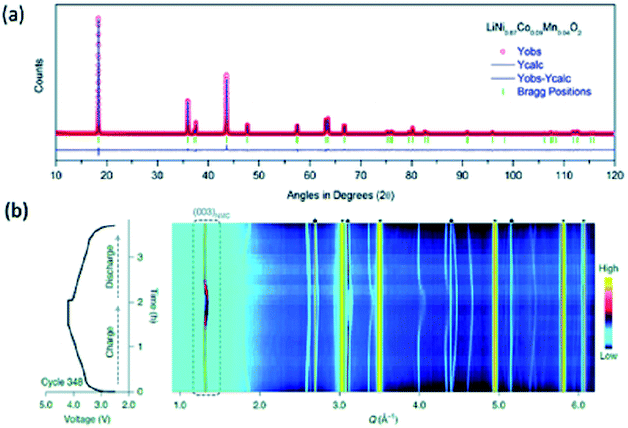 |
| Fig. 5 (a) Refined HRPD pattern for LiNi0.87Co0.09Mn0.04O2 cathode material of LIB battery. (b) Voltage profile during cycle 348 of LiNi0.8Mn0.1Co0.1O2 and the corresponding diffraction patterns. The colour scale indicates the intensity of the diffraction signals in arbitrary units. Reproduced from ref. 155 with permission from [Springer Nature], copyright [2021]. | |
Table 2 Structural and refined parameters from Rietveld refinement of HRPD pattern
The normalized site occupation numbers in % are: Li1 : Ni2 (97.52 : 2.48), Ni1 : Co : Mn (84.48 : 8.99 : 3.88), O (100). Fixed parameter. The occupancy were achieved by using the constraints as follows. Li3a + Ni3a = 0.08333, Mn3b + Co3b + Ni3b + Ni3a = 0.08333. |
Space group |
R m |
a (Å)/c (Å) |
2.87402(2)/14.2018(1) |
Atom |
Site |
Wyckoff positions |
Occupancya,c |
Li |
3a |
0 |
0 |
0 |
0.0812(2) |
Ni2 |
3a |
0 |
0 |
0 |
0.0021(2) |
Ni1 |
3b |
0 |
0 |
0.5 |
0.0703b |
Co |
3b |
0 |
0 |
0.5 |
0.0075b |
Mn |
3b |
0 |
0 |
0.5 |
0.0032b |
O |
6c |
0 |
0 |
0.2419(1) |
0.166b |
Reliability factors |
Rp = 6.67%, Rwp = 9.22%, Rexp = 5.99%, S = 1.54 |
This technique is used to establish the reversibility of crystallographic changes in NCM material during an electrochemical cycle.138–140 Numerous reports are available showing the abrupt lattice collapse in the material during charging of LIB having Ni-rich cathode.141–143 This phenomenon is ascribed to the Li utilization in the cathode material during charging and discharging by using in situ synchrotron X-ray diffraction.144
The use of synchrotron X-ray is not only limited to structural phase identification and quantitative estimation of lattice constants but it is also explored to investigate phase transition in Li-rich layered Li1.23Ni0.09Co0.12Mn0.56O2 during cycling,145 LiNi1/3Mn1/3Co1/3O2 at various C-rates146 and in LiNi0.85Co0.10Mn0.05O2.147 This technique also gives information of metal ion occupancy in the lattice,148,149 cation disorder150–152 by suitable refinement procedure. By employing in situ methodology, it can track cationic ordering/disordering in Ni-rich cathode during electrochemical cycle.153
Fatigue phase formation during a long cycle has also been established by synchrotron X-ray.154,155 In order to throw light on fatigue phase process, in situ X-ray study was reported by Xu et. al.155 Fig. 5c shows the phase segregation in polycrystalline LiNi0.8Mn0.1Co0.1O2 at a high state of charge (SoCs) at voltage profile during a particular cycle.155 This is associated with fatigue phase formation induced by surface reconstructions.156
In situ heating XRD, which is also termed as time-resolved (TR) XRD is a unique technique for investigation of real-time structural changes in the material during heating.157,158 Thus, it helps to understand the degradation and stability of cathode materials.159–163 These measurements show that thermal stability of charged LiNixMnyCozO2 (NMC, with x + y + z = 1, x
:
y
:
z = 4
:
3
:
3 (NMC433), 5
:
3
:
2 (NMC532), 6
:
2
:
2 (NMC622), and 8
:
1
:
1 (NMC811)) cathode decreases with increasing Ni content.159 Using this technique, Lipson et al., have shown that doping with Mg and Zr can impart substantial stabilization to Ni-rich cathode.164
Chemical/oxidation state investigation
X-rays have sufficient energy to eject a core electron from an atom. Each core–shell has distinct binding energy. When the X-ray energy is scanned through the binding energy of a core–shell, there is an abrupt increase in absorption cross-section (Fig. 6a).165 This gives rise to a so-called absorption edge, with each edge representing a different core–electron binding energy. These edges are named according to the principle quantum number, n, of the electron that is excited (Fig. 6b). For example, K-edge for n = 1, L-edge for n = 2, M-edge for n = 3, etc.
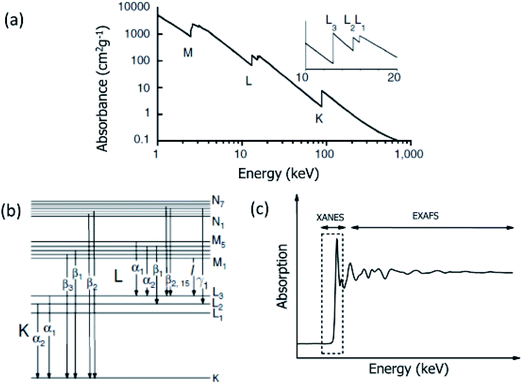 |
| Fig. 6 (a) Absorbance as a function of energy. There is abrupt change in the absorbance corresponding to K, L and M shells. Inset (a) shows similar changes observed for L1, L2 and L3 subshells. (b) Atomic energy levels showing electronic transitions corresponding to various shells. (c) A typical X-ray absorption spectrum showing the XANES and EXAFS regions. | |
The core-electron binding energy increases with increasing atomic number, ranging from 284 (C K-edge) to 115
606 eV (U K-edge).166 Thus, XAS is the measurement of the binding energy of elements by scanning material through X-ray.
XAS can probe the local electronic/atomic structure, thereby depicts information on oxidation states and atomic coordination. Thus, it is suitable to investigate a complete account of the surroundings of the element under investigation.167,168 These measurements, when performed for the X-ray energy up to 1000 eV from the main edge of element, are known as extended X-ray absorption fine structure (EXAFS) and are termed as X-ray absorption near edge fine structure (XANES) when performed in the energy range −20 to 60 eV from the main edge (Fig. 6c). The elements in the energy range of −20 to 60 eV from their edges can be probed either using hard X-ray or soft X-ray. However, measurement is often termed as XANES in the case of hard X-rays and near-edge X-ray absorption fine structure (NEXAFS) in the case of soft X-rays.
NEXAFS
Fig. 7a shows the optical layout of the soft-X-ray beamline having energy range of 200 to 1500 eV.169 NEXAFS spectrum for a given material can be measured in either total electronic yield (TEY) or total fluorescence yield (TFY) modes along with the angle dependence depending upon the requirement (Fig. 7b).170,171 TEY measurements probe the surface of materials (∼5 nm),172 however, depth is probed using TFY counterpart (50-100 nm).173 Fig. 7c shows the Ni L-edge NEXAFS spectra in TEY (i) and TFY mode (ii) for NCM attached with Li7La3Zr2O12 (LLZO) and Li2CoO2.174 These measurements reflect significant changes on the surface and bulk of this cathode.
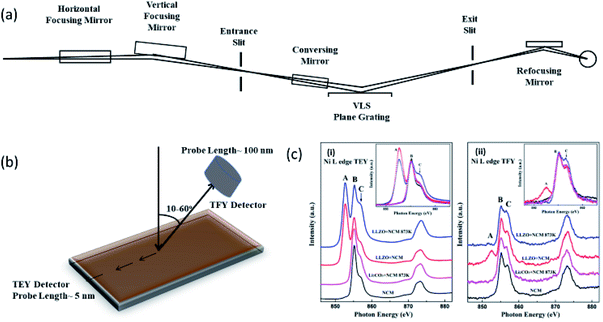 |
| Fig. 7 (a) Optical lay-out of soft X-ray beamline. Reproduced from ref. 169 with permission from [Elsevier], copyright [2007]. (b) Schematic representation of TEY and TFY mode; (c) NEXAFS spectra of NCM cathodes attached to LLZO and LiCoO2 cathodes. Reproduced from ref. 174 with permission from [ACS], copyright [2018]. | |
EXAFS
Fig. 8a depicts the schematic of beamline for measuring hard XAS.175 Experimental end station of this beamline is shown in Fig. 8b which exhibits the feasibility of performing measurements in both transmission and fluorescence mode. With a suitable experimental arrangement, coin cell can be studied during an electrochemical reaction (Fig. 8c).176 Thus, this beamline provides facility to probe cathodes either in situ176 or ex situ mode.177 Fig. 8d shows the Mn K-edge XAS spectra of Li2MnSO4 cathode at different charging and discharging states along with Mn oxide references.178
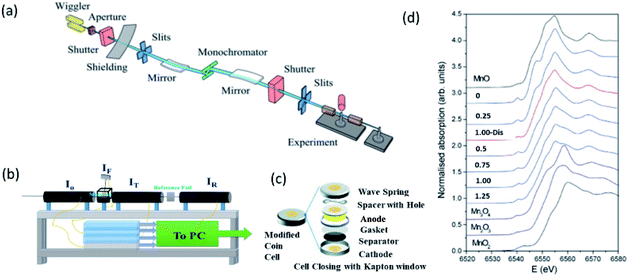 |
| Fig. 8 (a) Schematic of bending magnetic based XAS beamline. Reproduced from ref. 173 with permission from [AIP Publishing], copyright [2010]. (b) Representative end station for measuring XAS spectrum. (c) Schematic of coin cell used for in situ XAS measurements of cathode materials. Reproduced from ref. 176 with permission from [Springer Nature], copyright [2018]. (d) Mn K-edge XAS spectra of Li2−xMnSO4 cathode at different charging (x = 0.0–1.25) and discharging (x = 1.0-dis) states along with Mn oxides. Reproduced from ref. 178 with permission from [Elsevier], copyright [2008]. | |
Both the NEXAFS and XANES measurements can give information on the oxidation state of constituent ions of cathodes.179 The oxidation state of Mn ions in LiMn2O4 is determined by comparing it's XANES spectra with that of Mn2O3 and MnO2. Mn ions have a 3.5 valence state in this cathode (Fig. 9a).180 These measurements show that Mn3+ oxidation state is a mixture of the high spin (HS) and low spin (LS) states in LiMnO2 cathode.181 The exact information of Mn oxidation state in LiMn2O4 is carried out by plotting half-height energy values for the reference compound. The procedure of determining the oxidation state of unknown compound is depicted in Section S1.† This estimates the valence state of Mn at the fully discharged state to be approximately 3.6+.182
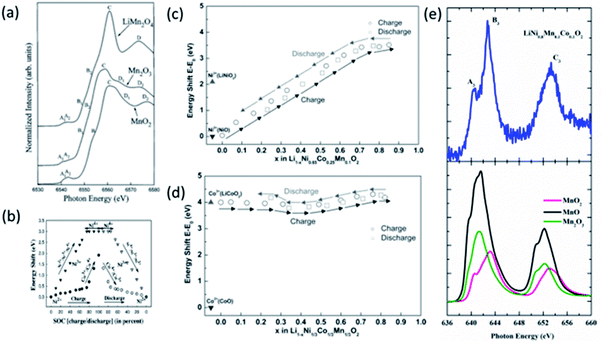 |
| Fig. 9 (a) Mn K-edge XANES spectra of LiMn2O4 cathode material along with reference oxides, MnO, Mn2O3 and MnO2. Reproduced from ref. 180 with permission from [Elsevier], copyright [1997]. (b) Plot of the white line energy shift vs. the state of charge (SOC) for Co and Ni K edge. The filled symbols represent the data during charging (i.e. during delithiation), while the empty symbols represent the data during discharging (i.e. during lithiation). Reproduced from ref. 183 with permission from [AIP publishing], copyright [2005]; energy shift E–E0 of the (c) Co K-edge and (d) Ni K-edge at the half-height of the edge step for Li1−xNi1/3Co1/3Mn1/3O2 at different lithium concentration during first charge and discharge cycle. Reproduced from ref. 189 with permission from [ACS], copyright [2005]. (e) Mn L-edge NEXAFS spectrum of LiNi0.8Mn0.1O0.1 cathode material along with Mn oxides. | |
XANES studies show that the oxidation state of Ni, Mn, and Co ions are 2+, 4+, and 3+ in the NCM333 cathode.183 These estimated oxidation states are in line with that determined from X-ray photoelectron spectroscopy.184,185 This material depicts the change of oxidation state of Ni and Co ions from the energy shift of the white line (Section S1†) during the charging and discharging of cathode (Fig. 9b).183 Similar observations were carried out by Tian et al. for LiNi0.6Mn0.2Co0.2O2 using XAS186 and from soft X-ray spectroscopy.187 In situ hard XAS exhibits no change of Mn oxidation state during the de-lithiation and lithiation for LiNi0.6Mn0.2Co0.2O2 cathode. These measurements show systematic changes are observed for Ni and Co ions.188 This effect is associated with migration and insertion of Li ions into the NCM cathode as Ni oxidation state is sensitive to the Li content (Fig. 9c), however, it's influence is almost non-significant on the oxidation state of Co ions (Fig. 9d).189
Apart from the XANES measurements, NEXAFS is also helpful to get quantitative information on mixed oxidation states.190 Authors have reported the presence of mixed Fe2+ and Fe3+ oxidation states of Fe ions in LixFePO4 cathode material;191 Mn3+, and Mn4+ in Na0.44MnO2 electrode192 from these measurements. Mn K-edge NEXAFS spectrum of NCM811 along with MnO, Mn2O3, and MnO2 is shown in Fig. 9e. The spectral features A3, B3, and C3 are analogs to that observed for MnO2, revealing the 4+ oxidation.
In addition to depict the information of oxidation state via metal L-edge, NEXAFS also give information of M–O hybridization process from the O K-edge measurements. Information on this aspect can be revealed by analyzing the pre-edge region of the O K-edge spectrum of cathode.193–195 Our group has investigated this effect in NCM811 cathode using O K-edge NEXAFS during the charging and discharging.188 Since, in layered oxides, metal–oxygen bond is important for oxygen-redox activity,189,190 hence, information specific to M–O hybridization is very effective to gather information of this activity.196 Koo et al. explain the redox mechanism of both cationic and anionic activity across the full cycling range of NCM333 materials by determining M–O hybridization from the pre-edge region of O K-edge.197 Thus, these measurements persist a way to throw light on the redox mechanism in these cathodes.198,199
Local atomic structure investigation
EXAFS spectrum of material gives quantitative information of coordination number (N) and bond-lengths (R) using proper simulation process.200–202 VIPER,203 LARCH,204 EVAX205 and Demeter206 are the data processing package used for simulation purposes. A typical equation for the EXAFS, which is basis for simulation is given below |
 | (1) |
where Nz is the total number of atoms in the zth shell, λ is the mean free path of photoelectron, and fz is the backscattering amplitude. The term before sin function represents the amplitude of the wave, which can be explained using the following equation, |
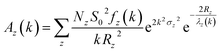 | (2) |
In eqn (2), σ represents a fluctuation in Rz due to structural disorder and temperature. The part sin [2kRz + δz(k)] of the EXAFS equation represents the oscillation. The term δz(k) is the phase shift. The EXAFS equation represents it's dependence on the number of atoms in a particular shell, bond distance Rz, scattering amplitude. Thus, proper adjustment of scattering amplitude and disorder term, S, enables one to determine the number of atoms and bond distance, which is done by simulating the EXAFS spectrum using a particular program.207,208 The parameters given in Table 3 need specific consideration during simulation.209,210 Thus, criteria for simulation of EXAFS spectrum210–212 can provide precise bond-length160,161 and coordination number of shells.213
Table 3 Parameters and their criteria for EXAFS simulation
Parameters |
Representation |
Origin |
Fitting criteria |
Characteristics |
Determination |
S02 |
Amplitude reduction factor |
Intrinsic losses due to inelastic effects in the EXAFS equation |
0.7 < S02 < 1.0 |
Remains same for similar structure |
From the standard |
N |
Degeneracy |
Coordination number of known atomic species |
|
Depends on the structure |
|
ΔR |
Change in bond-length |
Bond-length among atomic species |
≤0.05 |
|
Determined independently for each path |
ΔE0 |
Correction to main edge |
Uncertainty in determination of energy E0 |
≤2 |
|
One value for the energy shift (E0) can be applied to all paths |
σ2 |
Mean-squared displacement of the half path length |
The thermal and static disorders are similar in the coordination shells |
≤0.003 |
|
|
Fig. 10 shows the results obtained from XAS study of 0.5Li2MnO3·0.5LiCoO2, Li[Ni0.17Li0.2Co0.07Mn0.56]O2 and NCM333 cathodes.214,215 XANES spectra and k2-weighted Fourier-transformed EXAFS signals at the Mn and Co K-edges of the 0.5Li2MnO3·0.5LiCoO2 synthesized using ball-milling and sol–gel method are shown in Fig. 10a and b. The XANES spectra at Mn and Co edges almost overlap for both synthesis methods showing presence of these atoms in similar environments with quite similar local structures.
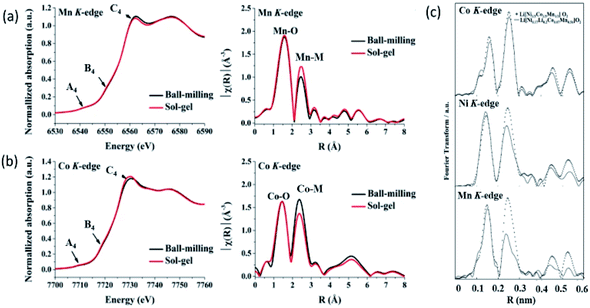 |
| Fig. 10 XANES spectra and k2-weighted Fourier-transformed EXAFS signals at the (a) Mn and (b) Co K-edges of the 0.5Li2MnO3·0.5LiCoO2 materials. Reproduced from ref. 214 with permission from [Springer Nature], copyright [2019]. (c) k3-Weighted Fourier transforms for Ni, Co, and Mn absorbers for Li[Ni0.17Li0.2Co0.07Mn0.56]O2 and Li[Ni1/3Co1/3Mn1/3]O2. Reproduced from ref. 215 with permission from [The Electrochemical Society of Japan], copyright [2010]. | |
To get deeper insights of local atomic structure, these authors have also compared the Fourier transform of EXAFS spectra and observed significant changes in the peak corresponding to Mn/Co–M bonds. These differences in EXAFS spectra due to the different Li2MnO3 and LiCoO2 domain sizes, indicating different phase separation behaviors. In another experiment carried out for Li[Ni0.17Li0.2Co0.07Mn0.56]O2 and NCM333, authors observed differences in the peak corresponding to M–O shell with dominant effect corresponding to Mn–O shell. This is associated with the difference in surrounding of Mn sites in both oxides. Quantitative information of local atomic structure revealed by simulation of EXAFS spectra is collated in Table 4.
Table 4 Co-ordination number (N). Bond-distance (R), Debye–Waller factor (σ2) for different materials estimated from Ni K-edge spectra. Reproduced from ref. 215 with permission from [The Electrochemical Society of Japan], copyright [2010]
Materials |
Shell |
N |
R (Å) |
σ2 (Å2) (10−5 nm2) |
N |
Fitting range (nm−1) |
Ni–O |
Co–O |
Mn–O |
Li[Ni0.17Li0.2Co0.07Mn0.56]O2 |
0.02023 ± 0.0011 |
|
|
5.1 |
6 |
20 < k < 120 |
|
0.1918 ± 0.0012 |
|
3.7 |
6 |
26 < k < 107 |
|
|
0.1913 ± 0.0010 |
2.6 |
6 |
27.5 < k < 119 |
Li[Ni1/3Co1/3Mn1/3]O2 |
0.02020 ± 0.0010 |
|
|
3.8 |
6 |
20 < k < 120 |
|
0.1910 ± 0.0012 |
|
2.2 |
6 |
26 < k < 107 |
|
|
0.1922 ± 0.0011 |
3.1 |
6 |
27.5 < k < 119 |
LiNiO2 |
0.197 |
|
|
12.1 |
6 |
27 < k < 118 |
LiCoO2 |
|
0.193 |
|
3.0 |
6 |
27 < k < 121 |
LiMnO2 |
|
|
0.192 |
2.3 |
6 |
27.5 < k < 120 |
Tsai et al. quantitatively investigated the M (Ni, Mn, & Co)–O and M–M bond lengths in NCM333 cathode using EXAFS studies during cycling. Reduction of bond-lengths with the increase of Li-ion concentration is reported by this group.189 M–O bond-lengths in Li[Ni0.17Li0.2Co0.07Mn0.56]O2 cathode material decrease upon charging and retains values equivalent to original after discharging.182 These studies are carried out to investigate the modulation of both CNs and bond-length of Ni–O and Ni-metal during charging, discharging for NCM333 cathode during various stages of charging and discharging.183
XAS also gives information of vacancies in cathode materials of LIB.216,217 These kinds of investigations give understanding of the degradation mechanisms in NCM cathode materials.218,219 Using this technique, Lee et al. reported that the formation of oxygen vacancies around Ni could be inferred to be more pronounced in a cathode material with a higher Ni content.220
Chemical phase mapping
Chemical phase mapping is the process of visualizing the regions of different oxidation states in a particular material by measuring XANES spectra (Fig. 11a). Technique to obtain this mapping is known as XANES-imaging. In this technique, multiple 2d images of material are produced to obtain spatially resolved information.221–223 Fig. 11b illustrates the change of intensity distribution for 2d images with a change of X-ray energy (Fig. 11b). Thus, pixel counts as a function of X-ray energy forms the XANES spectrum of the element in the material.
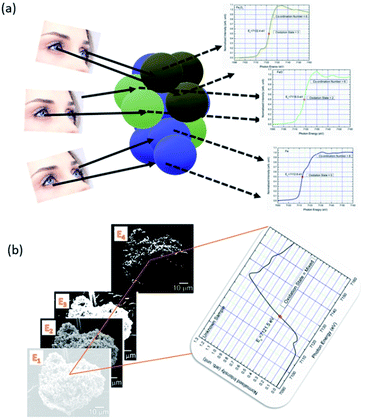 |
| Fig. 11 (a) Schematic representation of visualization of regions of different oxidation states in materials. (b) Principle of obtaining of XANES spectrum from X-ray imaging. | |
In this experiment, a set of raw data obtained from 2d imaging XAS measurement consists of a 3d array of the transmitted X-ray intensities, I (E′(x,y)), where, E′ is the apparent X-ray energy and (x,y) is the measurement position on the sample.224
The X-ray absorbance, μt at each position (x,y) is expressed by the following equation,
|
μt(E′,x,y) = ln[I0(E′,x,y)/I(E′,x,y)]
| (3) |
where, the ionization chamber measures the incident X-ray intensities, the
I0(
E′,
x,
y) term is replaced by the detected value at
E′. The spectrum for a specific area can be obtained by integrating
μt(
E′,
x,
y).
To get the XANES-imaging spectrum, the samples are mounted on a platform placed between the incident X-ray and charged coupled device (CCD) detector, as shown in Fig. 12a. Sample images (Fig. 12b) along with background images (Fig. 12c) at different X-ray energies are recorded using a CCD.225 After obtaining various images, each image at specific X-ray energy is corrected with respect to the background. These images are aligned and converted into the XANES spectrum. After obtaining, spectrum, chemical speciation of the material is performed using linear combination fitting (LCF).
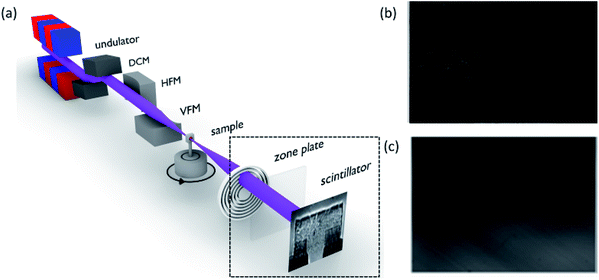 |
| Fig. 12 (a) Schematic of a typical X-ray-imaging beamline for XANES measurements. DCM-double crystal monitor, HFM-horizontal focusing mirror, VFM-vertical focusing mirror. Reproduced from ref. 225 with permission from [IUCr], copyright [2020]. (b) X-ray image measured for NCM111 cathode material and (c) background image. | |
Thus, XANES-imaging experiments are performed along with appropriate references to get chemical phase mapping of material. Schematic of obtaining chemical phase mapping is given as ESI (Section S2†).
This technique is considered suitable for determining chemical phase mapping at various states of charging and discharging. 2d-XANES imaging applied to LiFePO4 cathode material of Li-battery shows that both the LiFePO4 and FePO4 phase co-exists during charging.226 In another work, this technique is applied to NCM622 cathode (Fig. 13a)227 and to visualize the Ni oxidation state distribution in radially aligned grains and randomly oriented grains-NCM.228 Fig. 13(a) shows that a near-complete phase transition occurs at the final charge and discharge stage, indicating highly electrochemical reversibility in case of NCM622. Nevertheless, high reversibility cannot survive after 200 cycles, which is unambiguously revealed through operando transmission X-ray microscopy (TXM) chemical phase mapping. During the 201st charge cycle a highly heterogeneous chemical phase distribution appears in single-crystal NCM (Fig. 13a).228
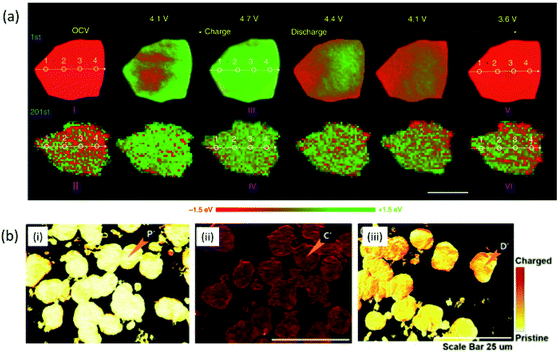 |
| Fig. 13 (a) Operando 2D chemical phase mappings at the Ni K-edge of NCM particles during the first and 201st cycles. Scale bars 2 μm. Reproduced from ref. 227 with permission from [Springer Nature], copyright [2020]. (b) XANES-mapping of pristine, charged and discharged cathode materials. Reproduced from ref. 229 with permission from [Elsevier], copyright [2020]. | |
In a recent study from our group,229 this technique is effectively applied to visualize the chemical distribution map of the discharged cathode at a charging rate of 3C. The results are shown in Fig. 13b. The results also exhibit the reversibility of chemical phase after comparison of pristine (i), charged (ii) and discharged NCM333 cathode (ii).
Tomography
X-ray imaging is well established to quantify the deformation of a single secondary particle, and its detailed investigation is discussed by Liu et al. for NCM cathode.230,231 Experimental procedure and tomographic images obtained for LiNi0.8Co0.1Mn0.1O2 cathode are shown in Fig. 14a (Section S3†). These measurements revealed almost 1.85% porosity of the secondary particle (Fig. 14b). Sub-micron focused operando synchrotron XRD and in situ ptycho-tomographic nano-scale imaging of a single nano-structured LiNi0.8Co0.1Mn0.1O2 core–shell particle during charge gives a thorough understanding of the anisotropic deformation and damage phenomena at a particle level (Fig. 14c).232
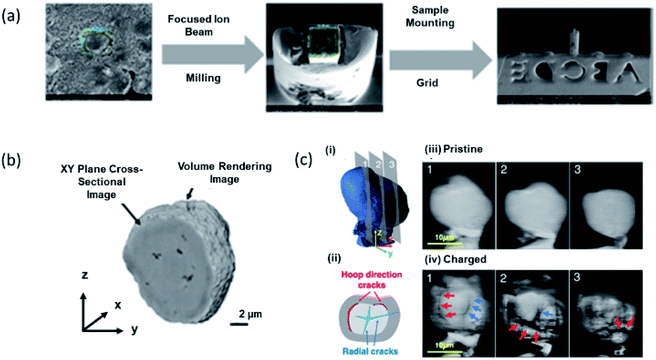 |
| Fig. 14 (a) Sample preparation for X-ray tomographic measurements. (b) X-ray tomographic image of LiNi0.8Co0.1Mn0.1O2 cathode. (c) (i) 3D ptycho-tomography reconstruction of a single particle in a pristine state with an indication of cross-sectional planes 1–3 shown on the right. (ii) Reconstructed ptychography slices of pristine single particle. (iii) Crack types observed after single charge: cracks in radial direction initiated in the core (blue) and cracks in hoop direction along core–shell interface (red). (iv) Reconstructed ptychography slices after a single charge up to 17.8% SoC, revealing significant microstructural degradation in radial direction (blue arrows) and along the core–shell interface (red). Reproduced from ref. 232 with permission from [RSC], copyright [2020]. | |
Thus, these techniques are very effective in probing the cathode materials of LIB, and numerous researchers carefully review their effectiveness with a combined approach to understand the degradation mechanism by looking into structure, local atomic structure, chemical phase mapping and tomography of the cathode.233–235 However, there is a rare use of these techniques in combined way. Our group recently investigated by combining these techniques that first cycle irreversibility is associated with the irreversibility of Ni oxidation states. Chemical state irreversibility of Ni ions is affected by the pore distribution in the cathode. During the first cycle, both the local structure and long-range structure remain unaltered.236 Thus, we believe that cumulative applications of these characterization techniques will be able to solve various issues of LIB. Since, still there are numerous challenges to exactly identifying the structural behaviour of NCM cathode surface, hence, the techniques such as single-shot coherent X-ray imaging237 and transient X-ray absorption spectroscopy238,239 developed at 4th generation synchrotron facility may be effective.240 However, the use of these techniques for battery research are in infant stages till date.
Conclusions
This review paper briefly describes Li-ion rechargeable batteries and related synchrotron techniques. A description of the phenomena that can be investigated using these techniques is also discussed in a concise way. XRD that determines long-range structure order is also helpful to throw light on the thermal stability of cathodes by temperature dependent measurements. This technique depicts the fatigue process in cathodes. XAS gives information on oxidation state and local atomic structure of cathode materials. It is also suitable to investigate the metal–oxygen hybridization process as well as oxygen vacancies in the cathodes. XANES-imaging visualizes the distribution of the chemical state on the particle surface. X-ray imaging provides tomographic information of particles of cathodes. It can also analyze cracks and pores quantitatively. Thus, synchrotron radiation analytical tools are effective to give a complete overview of the NCM cathodes during charging and discharging of LIB by their structure, local electronic/atomic structure, chemical phase mapping and tomography.
Author contributions
JPS, and SL conceptualise the idea. JPS wrote the manuscript in consultation with KHC, DA and SL. AKP revised the manuscript. SL and DA supervise the work. All authors have approved the manuscript.
Conflicts of interest
There are no conflicts to declare.
Acknowledgements
This research was supported by Commercializations Promotion Agency for R&D Outcomes (COMPA) funded by the Ministry of Science and ICT (1711149965). This work was also supported by Korea Institute of Science and Technology (KIST Project No. 2V09190). D. Ahn acknowledges financial supporting of the National Research Foundation of Korea (NRF) funded by the MSIT (2021M3H4A1A02049904) and Technology Innovation Program (Alchemist Project, 20012196, Al based supercritical materials discovery) funded by the Ministry of Trade, Industry & Energy, Korea. JPS acknowledges Science and Engineering Research Board, Department of Science and Technology, New Delhi, India for providing research grant through Ramanujan Fellowship (RJF2021/000115).
Notes and references
- H. Sun, Y. Zhang, J. Zhang, X. Sun and H. Peng, Nat. Rev. Mater., 2017, 2, 17023 CrossRef CAS.
- S. Staffell, D. Scamman, A. V. Abad, P. Balcombe, P. E. Dodds, P. Ekins, N. Shah and K. R. Ward, Energy Environ. Sci., 2019, 12, 463 RSC.
- T. Liu, Y. Zhang, Z. Jiang, X. Zeng, J. Ji, Z. Li, X. Gao, M. Sun, Z. Lin, M. Ling, J. Zheng and C. Liang, Energy Environ. Sci., 2019, 12, 1512 RSC.
- Q. Liu, Z. Hu, W. Li, C. Zhou, H. Jin, S. Wang, S. Chou and S. X. Dou, Energy Environ. Sci., 2021, 14, 158 RSC.
- A. Noori, M. F. El-Kady, M. S. Rahmanifar, R. B. Kaner and M. F. Mousavi, Chem. Soc. Rev., 2019, 48, 1272 RSC.
- K. Schmidt-Rohr, J. Chem. Educ., 2018, 95, 1801 CrossRef CAS.
- G. J. May, A. Davidson and B. Monahov, J. Energy Storage, 2018, 15, 145 CrossRef.
- M. G. Choi, K. M. Kim and Y.-Gi Lee, Curr. Appl. Phys., 2010, 10, e92 CrossRef.
- X. Chen, X. Liu, Q. Le, M. Zhang, M. Liu and A. Atrens, J. Mater. Chem. A, 2021, 9, 12367 RSC.
- G. Blomgren, Primary Batteries, Selection and Application, in Encyclopedia of Applied Electrochemistry, ed. G. Kreysa, K. Ota and R. F. Savinell, Springer, New York, NY, 2014, DOI:10.1007/978-1-4419-6996-5_387.
- H. Wang, X. Liang, J. Wang, S. Jiao and D. Xue, Nanoscale, 2020, 12, 14 RSC.
- M. Winter, B. Barnett and K. Xu, Chem. Rev., 2018, 118, 11433 CrossRef CAS PubMed.
- W. Chen, J. Liang, Z. Yang and G. Li, Energy Procedia, 2019, 158, 4363 CrossRef.
- Y. Liang, C.-Z. Zhao, H. Yuan, Y. Chen, W. Zhang, J.-Q. Huang, D. Yu, Y. Liu, M.-M. Titirici, Yu-L. Chueh, H. Yu and Q. Zhang, InfoMat, 2019, 1, 6 CrossRef CAS.
- C. P. Grey and D. S. Hall, Nat. Commun., 2020, 11, 6279 CrossRef CAS PubMed.
- F. Wu, J. Maier and Y. Yu, Chem. Soc. Rev., 2020, 49, 1569 RSC.
- M. Rosa Palacin, Acc. Mater. Res., 2021, 2, 319 CrossRef.
- D. E. Eapen, R. Suresh, S. Patil and R. Rengaswamy, Renewable Sustainable Energy Rev., 2021, 147, 111165 CrossRef CAS.
- T. Chen, Y. Jin, H. Lv, A. Yang, M. Liu, B. Chen, Y. Xie and Q. Chen, Trans. Tianjin Univ., 2020, 26, 208 CrossRef.
- M. Kwiecien, P. Schröer, M. Kuipers and D. U. Sauer, Current research topics for lead–acid batteries, in Lead-acid batteries for future automobiles, ed. J. Garche, E. Karden and D. A. J. Rand, 2017, p. 133 Search PubMed.
- K. Huang, J. Li and Z. Xu, Waste Manag., 2010, 30, 2292 CrossRef CAS PubMed.
- S. K. Dhar, S. R. Ovshínsky, P. R. Gifford, D. A. Corrigan, M. A. Fetcenko and S. Venkatesan, J. Power Sources, 1997, 65, 1 CrossRef CAS.
- H.-L. Chen, P.-S. Wu and J.-J. Wu, ACS Sustainable Chem. Eng., 2019, 20, 17100 CrossRef.
- B. Ji-Hoon, K. Sangwan, G. H. Dong and J.-C. Lee, ACS Appl. Mater. Interfaces, 2019, 11, 29718 CrossRef PubMed.
- A. Masias, J. Marcicki and W. A. Paxton, ACS Energy Lett., 2021, 6, 621 CrossRef CAS.
- J. Chidiac, L. Timperman and M. Anouti, J. Energy Chem., 2022, 65, 352 CrossRef.
- J. Jiang, G. Nie and P. Nie, Nano-Micro Lett., 2020, 12, 183 CrossRef CAS PubMed.
- G. Zhu, X. Tian, H.-C. Tai, Y.-Y. Li, J. Li, H. Sun, P. Liang, M. Angell, C.-L. Huang, C.-S. Ku, W.-H. Hung, S.-K. Jiang, Y. Meng, H. Chen, M.-C. Lin, B.-. Hwang and H. Dai, Nature, 2021, 596, 525 CrossRef CAS PubMed.
- J. W. Choi and D. Aurbach, Nat. Rev. Mater., 2016, 1, 16013 CrossRef CAS.
- R. Zhou, S. Wei, Y. Liu, N. Gao, G. Wang, J. Lian and Q. Jiang, Sci. Rep., 2019, 9, 3980 CrossRef PubMed.
- B. D. McCloskey, J. Phys. Chem. Lett., 2015, 6, 18 Search PubMed.
- J.-M. Tarascon and M. Armand, Nature, 2001, 414, 359 CrossRef CAS PubMed.
- R. Schmuch, R. Wagner, G. Hörpel, T. Placke and M. Winter, Nat. Energy, 2018, 3, 267 CrossRef CAS.
- W. Zuo, M. Luo, X. Liu, J. Wu, H. Liu, J. Li, M. Winter, R. Fu, W. Yang and Y. Yang, Energy Environ. Sci., 2020, 13, 4450 RSC.
- J. Xu, S. Dou, H. Liu and L. Dai, Nanoenergy, 2013, 2, 439 CAS.
- N. Nitta, F. Wu, J. T. Lee and G. Yushin, Mater. Today, 2015, 18, 252 CrossRef CAS.
- N. Yabuuchia, M. Takeuchi, M. Nakayama, H. Shiiba, M. Ogawa, K. Nakayama, T. Ohta, D. Endo, T. Ozaki, T. Inamasu, K. Sato and S. Komab, Proc. Natl. Acad. Sci. U. S. A., 2015, 112, 7650 CrossRef PubMed.
- T. Kim, W. Song, D.-Y. Son, L. K. Ono and Y. Qi, J. Mater. Chem. A, 2019, 7, 2942 RSC.
- T. M. Güra, Energy Environ. Sci., 2018, 11, 2696 RSC.
- X. Fan, B. Liu, J. Liu, J. Ding, X. Han, Y. Deng, X. Lv, Y. Xie, B. Chen, W. Hu and C. Zhong, Trans. Tianjin Univ., 2020, 26, 92 CrossRef.
- M. Li, J. Lu, Z. Chen and K. Amine, Adv. Mater., 2018, 30, 1800561 CrossRef PubMed.
- B. L. Ellis, K. T. Lee and L. F. Nazar, Chem. Mater., 2010, 22, 691 CrossRef CAS.
- U.-H. Kim, D.-W. Jun, K.-J. Park, Q. Zhang, P. Kaghazchi, D. Aurbach, D. T. Major, G. Goobes, M. Dixit, N. Leifer, C. M. Wang, P. Yan, D. Ahn, K.-H. Kim, C. S. Yoon and Y.-K. Sun, Energy Environ. Sci., 2018, 11, 1271 RSC.
- A. Mishra, A. Mehta, S. B. Shweta, J. Malode, N. P. Shetti, S. S. Shukla, M. N. Nadagouda and T. M. Aminabhavi, Mater. Sci. Energy Technol., 2018, 1, 182 Search PubMed.
- P. Roy and S. K. Srivastava, J. Mater. Chem. A, 2015, 3, 2454 RSC.
- Q. Zhang, Q.-F. Dongz, M.-S. Zheng and Z.-W. Tian, J. Electrochem. Soc., 2011, 158, A443 CrossRef CAS.
- W. Xu, J. Wang, F. Ding, X. Chen, E. Nasybulin, Y. Zhang and J.-G. Zhang, Energy Environ. Sci., 2014, 7, 513 RSC.
- S. Tardif, E. Pavlenko, L. Quazuguel, M. Boniface, M. Maréchal, J.-S. Micha, L. Gonon, V. Mareau, G. Gebel, P. B. Guillemaud, F. Rieutord and S. Lyonnard, ACS Nano, 2017, 11, 11306 CrossRef CAS PubMed.
- H. Aziam, G. Garhi, Y. Tamraoui, L. Ma, T. Wu, G. L. Xu, B. Manoun, J. Alami, K. Amine and I. Saadoune, Electrochim. Acta, 2018, 283, 1238 CrossRef CAS.
- H. Aziam, S. Indris, H. B. Youcef, R. Witte, A. Sarapulova, H. Ehrenberg and I. Saadoune, J. Alloys Compd., 2022, 906, 164373 CrossRef CAS.
- C. Liu, Z. G. Neale and G. Cao, Mater. Today, 2016, 19, 109 CrossRef CAS.
- W. Tang, G. Zhou, J. Cao, Z. Chen, Z. Yang, H. Huang, Y. Qu, C. Li, W. Zhang and H. Liu, ACS Appl. Energy Mater., 2021, 4, 2962 CrossRef CAS.
- F. Wu and G. Yusin, Energy Environ. Sci., 2017, 10, 435 RSC.
- R. Chen, T. Zhao, X. Zhang, L. Li and F. Wu, Nanoscale Horiz., 2016, 1, 423 RSC.
- H. Sun, J. Zhu, D. Baumann, L. Peng, Y. Xu, I. Shakir, Y. Huang and X. Duan, Nat. Rev. Mater., 2019, 4, 45 CrossRef.
- F. Yang, D. Wang, Y. Xing and K.-L. Tsui, Microelectron. Reliab., 2017, 70, 70 CrossRef CAS.
- S.-T. Myung, F. Maglia, K.-J. Park, C. S. Yoon, P. Lamp, S.-J. Kim and Y.-K. Sun, ACS Energy Lett., 2017, 2, 196 CrossRef CAS.
- G. Che, K. B. Jirage, E. R. Fisher, C. R. Martin and H. Yoneyama, J. Electrochem. Soc., 1997, 144, 4296 CrossRef CAS.
- J. Cho, Y. W. Kim, B. Kim, J. G. Lee and B. Park, Angew. Chem., Int. Ed., 2003, 42, 1618 CrossRef CAS PubMed.
- T. Ohzuku, A. Ueda and M. Nagayama, J. Electrochem. Soc., 1993, 140, 1862 CrossRef CAS.
- P. Bruce, A. R. Armstrong and R. L. Gitzendanner, J. Mater. Chem., 1999, 9, 193 RSC.
- F. Lin, I. M. Markus, D. Nordlund, T.-C. Weng, M. D. Asta, H. L. Xin and M. M. Doeff, Nat. Commun., 2014, 5, 3529 CrossRef PubMed.
- S. K. Martha, O. Haik, E. Zinigrad, I. Exnar, T. Drezen, J. H. Miners and D. Aurbach, J. Electrochem. Soc., 2011, 158, A1115 CrossRef CAS.
- R. Wang, X. He, L. He, F. Wang, R. Xiao, L. Gu, H. Li and L. Chen, Adv. Energy Mater., 2013, 3, 1358 CrossRef CAS.
- M.-J. Lee, S. Lee, P. Oh, Y. Kim and J. Cho, Nano Lett., 2014, 14, 993 CrossRef CAS PubMed.
- S. Choi and A. Manthiram, J. Electrochem. Soc., 2002, 149, A162 CrossRef CAS.
- A. Yamada, S. C. Chung and K. Hinokuma, J. Electrochem. Soc., 2001, 148, A224 CrossRef CAS.
- D. Choi, D. Wang, I. T. Bae, J. Xiao, Z. Nie, W. Wang, V. V. Viswanathan, J. L. Yun, J. G. Zhang, G. L. Graff, Z. Yang and J. Liu, Nano Lett., 2010, 10, 2799 CrossRef CAS PubMed.
- J. Lloris, C. Pérez Vicente and J. L. Tirado, Electrochem. Solid-State Lett., 2002, 5, A234 CrossRef CAS.
- A. Sobkowiak, M. R. Roberts, R. Younesi, T. Ericsson, L. Häggström, C. W. Tai, A. M. Andersson, K. Edström, T. Gustafsson and F. Björefors, Chem. Mater., 2013, 25, 3020 CrossRef CAS.
- J. Barker, R. K. B. Gover, P. Burns, A. Bryan, M. Y. Saidi and J. L. Swoyer, J. Power Sources, 2005, 146, 516 CrossRef CAS.
- W.-G. Ryu, H. S. Shin, M. S. Park, H. Kim, K. N. Jung and J. W. Lee, Ceram. Int., 2019, 45, 13942 CrossRef CAS.
- W. Hua, B. Schwarz, M. Knapp, A. Senyshyn, A. Missiul, X. Mu, S. Wang, C. Kübel, J. R. Binder and S. Indris, J. Electrochem. Soc., 2019, 166, A5025 CrossRef CAS.
- M. Stein IV, C. F. Chen, M. Mullings, D. Jaime, A. Zaleski, P. P. Mukherjee and C. P. Rhodes, J. Electrochem. Energy Convers. Storage, 2016, 13, 031001 CrossRef.
- A. Chakraborty, S. Kunnikuruvan, M. Dixit and D. T. Major, Isr. J. Chem., 2020, 60, 850 CrossRef CAS.
- L. Zhu, C. Bao, L. Xie, X. Yang and X. Cao, J. Alloys Compd., 2020, 831, 154864 CrossRef CAS.
- Q. Wang, B. Mao, S. I. Stoliarov and J. Sun, Prog. Energy Combust. Sci., 2019, 73, 95 CrossRef.
- A. Manthiram, B. Song and W. Li, Energy Storage Mater., 2017, 6, 125 CrossRef.
- X. Cheng, J. Zheng, J. Lu, Y. Li, P. Yan and Y. Zhang, Nano Energy, 2019, 62, 30 CrossRef CAS.
- H. Sun and K. Zhao, J. Phys. Chem. C, 2017, 121, 6002 CrossRef CAS.
- Q. Tao, L. Wang, C. Shi, J. Li, G. Chen, Z. Xue, J. Wang, S. Wang and H. Jin, Mater. Chem. Front., 2021, 5, 2607 RSC.
- L. Mu, Z. Yang, L. Tao, C. K. Waters, Z. Xu, L. Li, S. Sainio, Y. Du, H. L. Xin, D. Nordlund and F. Jin, J. Mater. Chem. A, 2020, 8, 17487 RSC.
- Y. Lu, Y. Zhang, Q. Zhang, F. Cheng and J. Chen, Particuology, 2020, 53, 1 CrossRef CAS.
- Y. Ruana, X. Song, Ya. Fu, C. Song and V. Battaglia, J. Power Sources, 2018, 400, 539 CrossRef.
- L. Wu, K.-W. Nam, X. Wang, Y. Zhou, J.-C. Zheng, X.-Q. Yang and Y. Zhu, Chem. Mater., 2011, 23, 3953 CrossRef CAS.
- B. Liu, Y. Jia, C. Yuan, L. Wang, X. Gao, S. Yin and J. Xu, Energy Storage Mater., 2020, 24, 85 CrossRef.
- V. G. Bagrova, J. Synchrotron Radiat., 2018, 25, 1619 CrossRef PubMed.
- D. Rouan, Synchrotron Radiation, in Encyclopedia of Astrobiology, ed. M. Gargaud, et al., Springer, Berlin, Heidelberg, 2015 Search PubMed.
- P. F. Tavares, S. C. Leemann, M. Sjöström and Å. Andersson, J. Synchrotron Radiat., 2014, 21, 862 CrossRef CAS PubMed.
- S. Moniri and P. Taherparvar, J. Instrum., 2019, 14, T02001 CrossRef CAS.
- L. Filler, Design of three bending magnets for use with beam from target to eight-inch pipe, United States, 1968, DOI:10.2172/1157343.
- G. Geloni, V. Kocharyan and E. Saldin, J. Synchrotron Radiat., 2015, 22, 288 CrossRef CAS PubMed.
- K. J. Kim, AIP Conf. Proc., 1989, 184, 565 CrossRef.
- J. L. Laclare, Nucl. Instrum. Methods Phys. Res., Sect. A, 2001, 467–468, 1 CrossRef CAS.
- C. A. Smith, G. L. Card, A. E. Cohen, T. I. Doukov, T. Eriksson, A. M. Gonzalez, S. E. McPhillips, P. W. Dunten, I. I. Mathews, J. Song and S. M. Soltis, J. Appl. Crystallogr., 2010, 43, 1261 CrossRef CAS PubMed.
- Y. Hirose, R&D Rev. Toyota CRDL, 2003, 38, 1 Search PubMed.
- E. Mitchell, P. Kuhn and E. Garman, Structure, 1999, 7, R111 CrossRef CAS PubMed.
- Y. U. Sohn, J.-Y. Choi, S. N. Kim, K. R. Kim, J. S. Bak and Y. M. Koo, Nucl. Instrum. Methods Phys. Res., Sect. A, 2001, 467–468, 707 CrossRef CAS.
- H. Winick and G. P. Williams, Synchrotron Radiat. News, 1991, 4, 23 CrossRef.
- H. H. Lee, M. Kumar and H.-J. Shin, Phys. Adv. Technol., 2017, 26, 7 CrossRef.
- D. Céolin, J. M. Ablett, D. Prieur, T. Moreno, M. J.-P. Rueff, T. Marchenko, L. Journel, R. Guillemin, T. Marin and M. Simon, J. Electron Spectrosc. Relat. Phenom., 2013, 190, 188 CrossRef.
- P. S. Bagus, E. Ilton and C. J. Nelin, Catal. Lett., 2018, 148, 1785 CrossRef CAS.
- Y. Yang, S. Harmer and M. Chen, Hydrometallurgy, 2015, 156, 89 CrossRef CAS.
- J. Yano and V. K. Yachandra, Photosynth. Res., 2009, 102, 241 CrossRef CAS PubMed.
- W. Li, M. Li, Y. Hu, J. Lu, A. Lushington, R. Li, T. Wu, T.-K. Sham and X. Sun, Small Methods, 2018, 2, 1700341 CrossRef.
- M. Fehse, A. Iadecola, L. Simonelli, A. Longo and L. Stievano, Phys. Chem. Chem. Phys., 2021, 23, 23445 RSC.
- T. Alemu and F.-M. Wang, J. Synchrotron Radiat., 2018, 25, 151 CrossRef CAS PubMed.
- Y. Takata, K. Tamasaku, T. Tokushima, D. Miwa, S. Shin, T. Ishikawa, M. Yabashi, K. Kobayashi, J. J. Kim, T. Yao, T. Yamamoto, M. Arita, H. Namatame and M. Taniguchi, Appl. Phys. Lett., 2001, 84, 4310 CrossRef.
- G. der Laan and A. I. Figueroa, Coord. Chem. Rev., 2014, 277–278, 95 CrossRef.
- A. Q. R. Baron, Y. Tanaka, S. Goto, K. Takeshita, T. Matsushita and T. Ishikawa, J. Phys. Chem. Solids, 200, 61, 461 CrossRef.
- J. McBreen, J. Solid State Electrochem., 2009, 13, 1051 CrossRef CAS.
- H. Ade, X. Zhang, S. Cameron, C. Costello, J. Kirz and S. Williams, Science, 1992, 258, 972 CrossRef CAS PubMed.
- J. Hilhorst, F. Marschall, T. N. Tran Thi, A. Last and T. U. Schülli, J. Appl. Crystallogr., 2014, 47, 1882 CrossRef CAS.
- F. Meirer, Y. Liu, E. Pouyet, B. Fayard, M. Cotte, C. Sanchez, J. Andrews, A. Mehta and P. Sciau, J. Anal. At. Spectrom., 2013, 28, 1870 RSC.
- P. Fischer, D.-H. Kim, B. L. Mesler, W. Chao and E. H. Anderson, J. Magn. Magn. Mater., 2007, 310, 2689 CrossRef CAS.
- P. Tack, J. Garrevoet, S. Bauters, B. Vekemans, B. Laforce, E. V. Ranst, D. Banerjee, A. Longo, W. Bras and L. Vincze, Anal. Chem., 2014, 86, 8791 CrossRef CAS PubMed.
- K. J. Gaffney and H. N. Chapman, Science, 2007, 316, 1444 CrossRef CAS PubMed.
- J. McBreen, J. Solid State Electrochem., 2009, 13, 1051 CrossRef CAS.
- M. Giorgetti, ISRN Mater. Sci., 2013, 2013, 938625 Search PubMed.
- P. P. R. M. L. Harks, F. M. Mulder and P. H. L. Notten, J. Power Sources, 2018, 288, 92 CrossRef.
- E. Talaie, P. Bonnick, X. Sun, Q. Pang, X. Liang and L. F. Nazar, Chem. Mater., 2017, 29, 90 CrossRef CAS.
- F. Lin, Y. Liu, X. Yu, L. Cheng, A. Singer, O. G. Shpyrko, H. L. Xin, N. Tamura, C. Tian, T. C. Weng, X.-Q. Yang, Y. S. Meng, D. Nordlund, W. Yang and M. M. Doeff, Chem. Rev., 2017, 117, 13123 CrossRef CAS PubMed.
- B. Bozzini and A. Goldoni, J. Phys. D: Appl. Phys., 2018, 51, 050201 CrossRef.
- T. M. M. Heenan, C. Tan, J. Hack, D. J. L. Brett and P. R. Shearing, Mater. Today, 2019, 31, 69 CrossRef CAS.
- S. K. Babu, A. I. Mohamed, J. F. Whitacre and S. Litster, J. Power Sources, 2015, 283, 314 CrossRef.
- A. V. Llewellyn, A. Matruglio, D. J. L. Brett, R. Jervis and P. R. Shearing, Condens. Matter, 2020, 5, 75 CrossRef CAS.
- S. Wang, W. Hua, S. Zhou, X. He and L. Liu, Chem. Eng. J., 2020, 400, 125998 CrossRef CAS.
- H. J. Kim, J. H. Jo, J. U. Choi, N. Voronina, D. Ahn, T.-Y. Jeon, H. Yashiro, Y. Aniskevich, G. Ragoisha, E. Streltsov and S.-T. Myung, Energy Storage Mater., 2021, 40, 197 CrossRef.
- J. Kim, D. Ahn, C. Kulshreshtha, K.-S. Sohn and N. Shin, Acta Crystallogr., Sect. C: Cryst. Struct. Commun., 2009, 65, i14 CrossRef CAS PubMed.
- J. I. Sohn, H. J. Joo, K. S. Kim, H. W. Yang, A.-R. Jang, D. Ahn, H. H. Lee, S. N. Cha, D. J. Kang, J. M. Kim and M. E. Welland, Nanotechnology, 2012, 23, 205707 CrossRef PubMed.
- S. Senbhagaraman, T. N. Guru Row and A. M. Umarji, J. Mater. Chem., 1993, 3, 309 RSC.
- J. P. Singh, S. O. Won, W. C. Lim, I. J. Lee and K. H. Chae, J. Mol. Struct., 2016, 1108, 444 CrossRef CAS.
- S. Weber, J. Appl. Crystallogr., 1997, 30, 565 CrossRef CAS.
- G. Rodriguez and S. Rodriguez, J. Chem. Educ., 1989, 66, 648 CrossRef CAS.
- D. L. Bish and S. A. Howard, J. Appl. Crystallogr., 1988, 21, 86 CrossRef CAS.
- W. Hua, K. Wang, M. Knapp, B. Schwarz, S. Wang, H. Liu, J. Lai, M. Müller, A. Schökel, A. Missyul, D. F. Sanchez, X. Guo, J. R. Binder, J. Xiong, S. Indris and H. Ehrenberg, Chem. Mater., 2020, 32, 4984 CrossRef CAS.
- W. Lee, S. Lee, E. Lee, M. Choi, R. Thangavel, Y. Lee and W. S. Yoon, Energy Storage Mater., 2022, 44, 441–451 CrossRef.
- S. Hwang, S. M. Kim, S.-M. Bak, K. Y. Chung and W. Chang, Chem. Mater., 2015, 27, 6044 CrossRef CAS.
- Z. Feng, R. Rajagopalan, S. Zhang, D. Sun, Y. Tang, Y. Ren and H. Wang, Adv. Sci., 2021, 8, 2001809 CrossRef CAS PubMed.
- P. Wang, P. Li, T.-F. Yi, H. Yu, X. Lin, S. Qian, Y. R. Zhu, M. Shui and J. Shu, Electrochim. Acta, 2016, 190, 248 CrossRef CAS.
- M. Jeong, H. Kim, W. Lee, S.-J. Ahn, E. Lee and W.-S. Yoon, J. Power Sources, 2020, 474, 228592 CrossRef CAS.
- N. Li, S. Sallis, J. K. Papp, B. D. McCloskey, W. Yang and W. Tong, Nano Energy, 2020, 78, 105365 CrossRef CAS.
- A. O. Kondrakov, H. Geßwein, K. Galdina, L. de Biasi, V. Meded, E. O. Filatova, G. Schumacher, W. Wenzel, P. Hartmann, T. Brezesinski and J. Janek, J. Phys. Chem. C, 2017, 121, 24381 CrossRef CAS.
- W. Li, H. Y. As, Q. Xie and A. Manthiram, J. Am. Chem. Soc., 2019, 141, 5097 CrossRef CAS PubMed.
- C. H Shen, Q. Wang, F. Fu, L. Huang, Z. Lin, S.-Y. Shen, H. Su, X.-M. Zheng, B.-B. Xu, J.-T. Li and S.-G. Sun, ACS Appl. Mater. Interfaces, 2014, 6, 5516 CrossRef PubMed.
- Y. N. Zhou, J. L. Yue, E. Hu, H. Li, L. Gu, K. W. Nam, S. M. Bak, X. Yu, J. Liu, J. Bai, E. Dooryhee, Z.-W. Fu and X.-Q. Yang, Adv. Energy Mater., 2016, 6, 1600597 CrossRef.
- J. Lai, J. Zhang, Z. Li, Y. Xiao, W. Hua, Z. Wu, Y. Chen, Y. Zhong, W. Xiang and X. Guo, Chem. Commun., 2020, 56, 4886 RSC.
- S. Gao, Y.-T. Cheng and M. Shirpour, ACS Appl. Mater. Interfaces, 2019, 11, 982 CrossRef CAS PubMed.
- J. Zheng, Y. Ye, T. Liu, Y. Xiao, C. Wang, F. Wang and F. Pan, Acc. Chem. Res., 2019, 52, 2201 CrossRef CAS PubMed.
- M. Jiang, Q. Zhang, X. Wu, Z. Chen, D. L. Danilov, R.-A. Eichel and P. H. L. Notten, ACS Appl. Energy Mater., 2020, 3, 6583 CrossRef CAS.
- T. Wang, K. Ren, W. Xiao, W. Dong, H. Qiao, A. Duan, H. Pan, Y. Yang and H. Wang, J. Phys. Chem. C, 2020, 124, 5600 CrossRef CAS.
- S. Wang, W. Hua, A. Missyul, M. S. D. Darma, A. Tayal, S. Indris, H. Ehrenberg, L. Liu and M. Knapp, Adv. Funct. Mater., 2021, 31, 2009949 CrossRef CAS.
- D. Wang, C. Xin, M. Zhang, J. Bai, J. Zheng, R. Kou, J. Y. P. Ko, A. Huq, G. Zhong, C. J. Sun, Y. Yang, Z. Chen, Y. Xiao, K. Amanine, F. Pan and F. Wang, Chem. Mater., 2019, 31, 2731 CrossRef CAS.
- S. Schweidler, L. de Biasi, G. Garcia, A. Mazilkin, P. Hartmann, T. Brezesinski and J. Janek, ACS Appl. Energy Mater., 2019, 2, 7375 CrossRef CAS.
- C. Xu, K. Märker, J. Lee, A. Mahadevegowda, P. J. Reeves, S. J. Day, M. F. Groh, S. P. Emge, C. Ducati, B. L. Mehdi, C. C. Tang and C. P. Grey, Nat. Mater., 2021, 20, 84 CrossRef CAS PubMed.
- Y. Kim, H. Park, K. Shin, G. Henkelman, J. H. Warner and A. Manthiram, Adv. Energy Mater., 2021, 11, 2101112 CrossRef CAS.
- N. E. Henriksen and K. B. Møller, J. Phys. Chem. B, 2008, 112, 558 CrossRef CAS PubMed.
- E. Hu, S. MinBak, S. D. Senanayake, X.-Q. Yang, K.-W. Nam, L. Zhang and M. Shao, J. Power Sources, 2015, 277, 193 CrossRef CAS.
- L. Wu, K.-W. Nam, X. Wang, Y. Zhou, J.-C. Zheng, X. Q. Yang and Y. Zhu, Chem. Mater., 2011, 23, 3953 CrossRef CAS.
- S. M. Bak, E. Hu, Y. Zhou, X. Yu, S. D. Senanayake, S.-J. Cho, K. B. Kim, K. Yoon Chung, X. Q. Yang and K. W. Nam, ACS Appl. Mater. Interfaces, 2014, 6, 22594 CrossRef CAS PubMed.
- X. Q. Yang, X. Sun, S. J. Lee, J. McBreen, S. Mukerjee, M. L. Daroux and X. K. Xing, Electrochem. Solid-State Lett., 1999, 2, 157 CrossRef CAS.
- D. Mohanty, J. Li, S. C. Nagpure, D. L. Wood III and C. Daniel, MRS Energy Sustain., 2015, 2, e15 CrossRef.
- K. W. Nam, S. M. Bak, E. Hu, X. Yu, Y. Zhou, X. Wang, L. Wu, Y. Zhu, K. Y. Chung and X. Q. Yang, Adv. Funct. Mater., 2013, 23, 1047 CrossRef CAS.
- A. L. Lipson, J. L. Durham, M. LeResche, I. A. Baker, M. J. Murphy, T. T. Fister, L. Wang, F. Zhou, L. Liu, K. Kim and D. Johnson, ACS Appl. Mater. Interfaces, 2020, 12, 18512 CrossRef CAS PubMed.
- E. Penner-Hahn, in X-ray Absorption Spectroscopy, eLS, 2005, DOI:10.1038/npg.els.0002984.
- S. Brennan and P. L. Cowan, Rev. Sci. Instrum., 1992, 63, 850 CrossRef.
- A. Sharma, J. P. Singh, S. O. Won, K. H. Chae, S. K. Sharma and S. K. Gautam, Introduction to X-ray absorption spectroscopy and its applications in material science, in Handbook of Materials Characterization, ed. S. Sharma, Springer, Cham 2018, pp. 497–548 Search PubMed.
- A. Sharma, M. Varshney, W. C. Lim, H. J. Shin, J. P. Singh, S. O. Won and K. H. Chae, Phys. Chem. Chem. Phys., 2017, 19, 6397 RSC.
- H.-N. Hwang, H.-S. Kim, B. Kim, C. C. Hwang, S. W. Moon, S. M. Chung, C. Jeon, C.-Y. Park, K. H. Chae and W. K. Choi, Nucl. Instrum. Methods Phys. Res., Sect. A, 2007, 581, 850 CrossRef CAS.
- J. P. Singh, S. H. Kim, S. O. Won, I. J. Lee and K. H. Chae, RSC Adv., 2018, 8, 31275 RSC.
- J. P. Singh and K. H. Chae, ACS Omega, 2019, 4, 7140 CrossRef CAS PubMed.
- G. Ali, J.-H. Lee, B. W. Cho, K.-W. Nam, D. Ahn, W. Chang, S. H. Oh and K. Y. Chung, Electrochim. Acta, 2016, 191, 307 CrossRef CAS.
- P. Thakur, V. Bisogni, J. C. Cezar, N. B. Brookes, G. Ghiringhelli, S. Gautam, K. H. Chae, M. Subramanian, R. Jayavel and K. Asokan, J. Appl. Phys., 2010, 107, 103915 CrossRef.
- N. Zhang, X. Long, Z. Wang, P. Yu, F. Han, J. Fu, G. X. Ren, Y. Wu, S. Zheng, W. Huang, C. Wang, H. Li and X. Liu, ACS Appl. Energy Mater., 2018, 1, 5968 CrossRef.
- I.-J. Lee, C.-J. Yu, Y.-D. Yun, C.-S. Lee, I. D. Seo, H.-Y. Kim, W.-W. Lee and K. H. Chae, Rev. Sci. Instrum., 2010, 81, 026103 CrossRef PubMed.
- S.-M. Bak, Z. Shadike, R. Lin, X. Yu and X.-Q. Yang, NPG Asia Mater., 2018, 10, 563 CrossRef.
- C.-J. Chang, Y. Zhu, J. Wang, H.-C. Chen, C.-W. Tung, Y.-C. Chu and H. M. Chen, J. Mater. Chem. A, 2020, 8, 19079 RSC.
- R. Dominko, I. Arčon, A. Kodre, D. Hanžel and M. Gaberšček, J. Power Sources, 2009, 189, 51 CrossRef CAS.
- G. Aquilanti, M. Giorgetti, R. Dominko, L. Stievano, I. Arcon, N. Novelo and L. Olivi, J. Phys. D: Appl. Phys., 2017, 50, 074001 CrossRef.
- R. S. Liu, L. Y. Jang, J. M. Chen, Y. C. Tsai, Y. D. Hwang and R. G. Liu, J. Solid State Chem., 1997, 128, 326 CrossRef CAS.
- H. Wadati, D. G. Hawthorn, T. Z. Regier, G. Chen, T. Hitosugi, T. Mizokawa, A. Tanaka and G. A. Sawatzky, Appl. Phys. Lett., 2010, 97, 022106 CrossRef.
- A. Ito, Y. Sato, T. Sanada, M. Hatano, H. Horie and Ya. Ohsawa, J. Power Sources, 2011, 196, 6828 CrossRef CAS.
- A. Deb, U. Bergmann, S. P. Cramer and E. J. Cairns, J. Appl. Phys., 2005, 97, 113523 CrossRef.
- K. M. Shaju, G. V. S. Rao and B. V. R. Chowdari, Electrochim. Acta, 2002, 48, 145 CrossRef CAS.
- Z. Chen, J. Wang, B. Tom, L. Bai, S. Chen, Y. Zhao, T. Sum, J. Lin and Z. Shen, Sci. Rep., 2016, 6, 25771 CrossRef CAS PubMed.
- K. R. Tallman, G. P. Wheeler, C. J. Kern, E. Stavitski, X. Tong, K. J. Takeuchi, A. C. Marschilok, D. C. Bock and E. S. Takeuchi, J. Phys. Chem. C, 2021, 125, 58 CrossRef CAS.
- C. Tian, Y. Xu, D. Nordlund, F. Lin, J. Liu, Z. Sun, Y. Liu and M. Doeff, Joule, 2018, 2, 464 CrossRef CAS.
- Xi. Liu, D. Wang, G. Liu, V. Srinivasan, Z. Liu, Z. Hussain and W. Yang, Nat. Commun., 2013, 4, 2568 CrossRef PubMed.
- Y. W. Tsai, B. J. Hwang, G. Ceder, H. S. Sheu, D. G. Liu and J. F. Lee, Chem. Mater., 2005, 17, 3191 CrossRef CAS.
- K. Kubobuchi, M. Mogi, M. Matsumoto, T. Baba, C. Yogi, C. Sato, T. Yamamoto, T. Mizoguchi and H. Imai, J. Appl. Phys., 2016, 120, 142125 CrossRef.
- A. Iadecola, A. Perea, L. Aldon, Gi. Aquilanti and L. Stievano, J. Phys. D: Appl. Phys., 2017, 50, 144004 CrossRef.
- Qi. Li, R. Qiao, L. A. Wray, J. Chen, Z. Zhuo, Y. Chen, S. Yan, F. Pan, Z. Hussain and W. Yang, J. Phys. D: Appl. Phys., 2016, 49, 413003 CrossRef.
- T. Liu, L. Yu, J. Liu, J. Lu, X. Bi, A. Dai, M. Li, M. Li, Z. Hu, L. Ma, D. Luo, J. Zheng, T. Wu, Y. Ren, J. Wen, F. Pan and K. Amine, Nat. Energy, 2021, 6, 277 CrossRef CAS.
- F. Frati, M. O. J. Y. Hunault and F. M. F. de Grooet, Chem. Rev., 2020, 120, 4056 CrossRef CAS PubMed.
- C. Liang, W. Zhang, Z. Wei, Z. Wang, Q. Wang and J. Sun, J. Energy Chem., 2021, 59, 446 CrossRef.
- J. P. Singh, J. Y. Park, K. Hwa Chae, D. Ahn and S. Lee, Nanomaterials, 2020, 10, 459 CrossRef PubMed.
- S. Koo, J. Lee, J. Lee, S. Yoon and D. Kim, Energy Storage Mater., 2021, 42, 764 CrossRef.
- J. Hong, W. E. Gent, P. Xiao, K. Lim, D.-H. Seo, J. Wu, P. M. Csernica, C. J. Takacs, D. Nordlund, C.-J. Sun, K. H. Stone, D. Passarello, W. Yang, D. Prendergast, G. Ceder, M. F. Toney and W. C. Chueh, Nat. Mater., 2019, 18, 256 CrossRef CAS PubMed.
- G. H. Lee, J. Wu, D. Kim, K. Cho, M. Cho, W. Yang and Y.-M. Kang, Angew. Chem., 2020, 132, 8759 CrossRef.
- A. D. Dwivedi, N. D. Sanadiya, J. P. Singh, S. M. Husnain, K. H. Chae, D. S. Hwang and Y. S. Chang, ACS Sustainable Chem. Eng., 2017, 5, 518 CrossRef CAS.
- V. Singh, A. K. Paidi, C.-H. Shim, S. H. Kim, S. O. Won, J. P. Singh, S. Lee and K. H. Chae, Crystals, 2021, 11, 490 CrossRef CAS.
- J. P. Singh, W. C. Lim, S. O. Won, J. Song and K. H. Chae, J. Korean Phys. Soc., 2018, 72, 890 CrossRef CAS.
- K. V. Klementiev, VIPER, freeware, J. Phys. D: Appl. Phys., 2001, 34, 209 CrossRef.
- M. Newville, J. Phys.: Conf. Ser., 2013, 430, 012007 CrossRef CAS.
- J. Timoshenko, A. Kuzmin and J. Purans, Comput. Phys. Commun., 2012, 183, 1237 CrossRef CAS.
- B. Ravel and M. Newville, J. Synchrotron Radiat., 2005, 12, 537 CrossRef CAS PubMed.
- M. Newville, Rev. Mineral. Geochem., 2014, 78, 33 CrossRef CAS.
- Z. Sun, W. Yan, T. Yao, Q. Liu, Y. Xie and S. We, Dalton Trans., 2013, 42, 13779 RSC.
- S. D. Kelly, D. Hesterberg and B. Ravel, Methods of soil analysis part 5 mineralogical methods, Soil Science Society of America, Madison, 2008, ch. 14 Search PubMed.
- D. C. Koningsberger, B. L. Mojet, G. E. Van Dorssen and D. E. Ramaker, Top. Catal., 2000, 10, 143 CrossRef CAS.
- E. Curis, J. Osan, G. Falkenberg, S. Benazeth and S. Torok, Spectrochim. Acta, 2015, 60, 841 CrossRef.
- E. Curis and S. Bénazeth, J. Synchrotron Radiat., 2015, 12, 361 CrossRef PubMed.
- C. Yin, Z. Wei, M. Zhang, B. Qiu, Y. Zhou, Y. Xiao, D. Zhou, L. Yun, C. Li, Q. Gu, W. Wen, X. Li, X. Wen, Z. Shi, L. He, Y. S. Meng and Z. Liu, Mater. Today, 2021, 51, 15–26 CrossRef CAS.
- S. Kaewmala, W. Limphirat, V. Yordsri, H. Kim, S. Muhammad, W.-S. Yoon, S. Srilomsak, P. Limthongkul and N. Meethong, Sci. Rep., 2019, 9, 427 CrossRef PubMed.
- A. Ito, Y. Sato, T. Sanada, T. Ohwaki, M. Hatano, H. Horie and Y. Ohawa, Electrochemistry, 2010, 78, 380 CrossRef CAS.
- S. Tao, W. Huang, S. Chu, B. Qian, L. Liu and W. Xu, Mater. Today Phys., 2021, 18, 100403 CrossRef CAS.
- P. Yan, J. Zheng, Z.-K. Tang, A. Devaraj, G. Chen, K. Amine, J.-G. Zhang, L.-M. Liu and C. Wang, Nat. Nanotechnol., 2019, 14, 602 CrossRef CAS PubMed.
- S.-K. Jung, H. Gwon, J. Hong, K.-Y. Park, D.-H. Seo, H. Kim, J. Hyun, W. Yang and K. Kang, Adv. Energy Mater., 2014, 4, 1300787 CrossRef.
- Y. Su, Q. Zhang, L. Chen, L. Bao, Y. Lu, Q. Shi, J. Wang, S. Chen and F. Wu, ACS Appl. Mater. Interfaces, 2020, 12, 37208 CrossRef CAS PubMed.
- L. Li, J. Chen, H. Huang, L. Tan, L. Song, H.-H. Wu, C. Wang, Z. Zhao, H. Yi, J. Duan, T. Dong, E. Lee, S. Muhammad, T. Kim, H. Kim, W. Lee and W.-S. Yoon, Adv. Sci., 2020, 7, 1902413 CrossRef PubMed.
- J. Lim, S. Y. Park, J. Y. Huang, S. M. Han and H. T. Kim, Rev. Sci. Instrum., 2013, 84, 013707 CrossRef PubMed.
- J. Lim, H. Kim and S. Y. Park, J. Synchrotron Radiat., 2014, 21, 827 CrossRef CAS PubMed.
- B. E. Etschmann, E. Donner, J. Brugger, D. L. Howard, M. D. de Jonge, D. Paterson, R. Naidu, K. G. Scheckel, C. G. Ryan and E. Lombi, Environ. Chem., 2014, 11, 341 CrossRef CAS.
- M. Katayama, K. Sumiwaka, K. Hayashi, K. Ozutsumi, T. Ohta and Y. Inada, J. Synchrotron Radiat., 2012, 19, 717 CrossRef CAS PubMed.
- J. Y. Park, J. P. Singh, J. Lim and S. Lee, J. Synchrotron Radiat., 2020, 27, 545 CrossRef CAS PubMed.
- J. Wang, Y. C. Karen Chen-Wiegert and J. Wang, Nat. Commun., 2014, 5, 4570 CrossRef CAS PubMed.
- F. Wang, S. Lou, S. Li, Z. Yu, Q. Liu, A. Dai, C. Cao, M. F. Toney, M. Ge, X. Xiao, W. K. Lee, Y. Yao, J. Deng, T. Liu, Y. Tang, G. Yin, J. Lu, D. Su and J. Wang, Nat. Commun., 2020, 11, 3050 CrossRef PubMed.
- Z. Xu, Z. Jiang, C. Kai, R. Xu, C. Qin, Y. Zhang, M. M. Rehman, C. Wei, D. Nordlund, C. J. Sun, X. Xiao, X. W. Du, K. Zhao, P. Yan, Y. Liu and F. Lin, Nat. Commun., 2020, 11, 83 CrossRef CAS PubMed.
- J. Y. Park, J. P. Singh, J. Lim, K. H. Chae and S. Lee, Mater. Lett., 2020, 261, 126983 CrossRef CAS.
- J. Le Houx and D. Kramer, Energy Reports, 2021, 7, 9 CrossRef.
- J. Y. Park, Y. Kim, S. Lee and J. Lim, J. Synchrotron Radiat., 2020, 27, 1696 CrossRef PubMed.
- L. R. Brandt, J.-J. Marie, T. Moxham, D. P. Förstermann, E. Salvati, C. Besnard, C. Papadaki, Z. Wang, P. G. Bruce and A. M. Korsunsky, Energy Environ. Sci., 2020, 13, 3556 RSC.
- M. Jiang, D. L. Danilov, R.-A. Eichel and P. H. L. Notten, Adv. Energy Mater., 2021, 11, 2103005 CrossRef CAS.
- J. Chen, W. Deng, X. Gao, S. Yin, L. Yang, H. Liu, G. Zou, H. Hou and X. Ji, ACS Nano, 2021, 15, 6061 CrossRef CAS PubMed.
- W. Hua, B. Schwarz, R. Azmi, M. Müller, M. S. D. Darma, M. Knapp, A. Senyshyn, M. Heere, A. Missyul, L. Simonelli, J. R. Binder, S. Indris and H. Ehrenberg, Nano Energy, 2020, 78, 105231 CrossRef CAS.
- J. P. Singh, J. Y. Park, A. K. Paidi, Y. Lee, J. Lim, K. H. Chae, D. Ahn and S. Lee, Probing Origin of First Cycle Irreversibility in the Ni-Rich Layered Oxide Cathode Materials: Role of Porosity and Chemical State Heterogeneity, communication.
- D. Sung, D. Nam, M.-j. Kim, S. Kim, K. S. Kim, S.-Y. Park, S. M. Hwang, C. Jung, H. Lee, D. H. Cho, M. Kim, I. Eom, S. Yong Lee, C. Song and S. Kim, Appl. Sci., 2021, 11, 5082 CrossRef CAS.
- J.-W. Lee, M. Kim, G. Kang, S. M. Vinko, L. Bae, M. S. Cho, H.-K. Chung, M. Kim, S. Kwon, G. Lee, C. H. Nam, S. H. Park, J. H. Sohn, S. H. Yang, U. Zastrau and B. I. Cho, Phys. Rev. Lett., 2021, 127, 175003 CrossRef CAS PubMed.
- I. Eom, S. H. Chun, J. H. Lee, D. Nam, R. Ma, J. Park, S. Park, S. H. Park, H. Yang, I. Nam, M. H. Cho, C. H. Shim, G. Kim, C.-K. Min, H. Heo, H.-S. Kang and C. Kim, Appl. Sci., 2022, 12, 1010 CrossRef CAS.
- I. Nam, C.-K. Min, B. Oh, G. Kim, D. Na, Y. J. Suh, H. Yang, M. H. Cho, C. Kim, M.-J. Kim, C. H. Shim, J. H. Ko, H. Heo, J. Park, J. Kim, S. Park, G. Park, S. Kim, S. H. Chun, H. J. Hyun, J. H. Lee, K. S. Kim, I. Eom, S. Rah, D. Shu, K.-J. Kim, S. Terentyev, V. Blank, Y. Shvyd'ko, S. J. Lee and H.-S. Kang, Nat. Photonics, 2021, 15, 435 CrossRef CAS.
|
This journal is © The Royal Society of Chemistry 2022 |
Click here to see how this site uses Cookies. View our privacy policy here.