DOI:
10.1039/D2RA00753C
(Paper)
RSC Adv., 2022,
12, 12235-12241
Regioselective C-3-alkylation of quinoxalin-2(1H)-ones via C–N bond cleavage of amine derived Katritzky salts enabled by continuous-flow photoredox catalysis†
Received
4th February 2022
, Accepted 24th March 2022
First published on 29th April 2022
Abstract
An efficient, transition metal-free visible-light-driven continuous-flow C-3-alkylation of quinoxalin-2(1H)-ones has been demonstrated by employing Katritzky salts as alkylating agents in the presence of eosin-y as a photoredox catalyst and DIPEA as a base at room temperature. The present protocol was accomplished by utilizing abundant and inexpensive alkyl amine (both primary and secondary alkyl) and as well as this a few amino acid feedstocks were converted into their corresponding redox-active pyridinium salts and subsequently into alkyl radicals. A wide variety of C-3-alkylated quinoxalin-2(1H)-ones were synthesized in moderate to high yields. Further this environmentally benign protocol is carried out in a PFA (Perfluoroalkoxy alkane) capillary based micro reactor under blue LED irradiation, enabling excellent yields (72% to 91%) and shorter reaction times (0.81 min) as compared to a batch system (16 h).
Introduction
Quinoxalin-2(1H)-ones represent a structurally privileged class of heterocyclic skeleton and widely exist in various classes of bioactive natural products, pharmaceutical agents and material science.1 In particular, installation of functional groups at the C-3-position of quinoxalin-2(1H)-ones has attracted considerable attention from chemists because of its extensive utilization in agrochemicals, pharmaceutical and organic materials and because it exhibits significant bioactive properties including antiviral, antidiabetic, antimicrobial, antibacterial, anticancer, anti-inflammatory and protein kinase inhibitory activities.2 A few representative drugs with a quinoxalinone skeleton are depicted in Fig. S1.†
Due to the wide range of applications in several fields, much effective strategies targeting their synthesis have been established,3 such as introducing aryl, acyl, phosphonate, thioether, trifluoromethyl and amino groups into C-3 position of quinoxalin-2(1H)-ones to acquire valuably functional quinoxalin-2(1H)-ones. However, C–H alkylation of quinoxalin-2(1H)-ones is still under explored (Scheme 1). In this context, Qu and co-workers reported alkylation, oxyalkylation and hydroxyalkylation of quinoxalin-2(1H)-ones under micro-wave irradiation conditions.4 Yang and co-workers developed direct C–H cyanoalkylation of quinoxalin-2(1H)-ones.5 Wei6 and Suryavanshi7 developed visible light induced transformations to deliver functionalized quinoxalin-2(1H)-ones. Jin, He and Liu's group achieved the alkylation of quinoxalin-2(1H)-ones via a visible-light-driven decarboxylation process.8 Roy's group also explored the alkylation using di-tert-butyl peroxide (DTBP) as an alkoxyl radical mediator for hydrogen atom transfer (HAT).9 More recently, Xuan et al. explored a method for alkylation of quinoxalin-2(1H)-ones that utilizes 4-alkyl-1,4- dihydropyridines (R-DHPs) as alkyl radical precursors via visible light photoredox catalysis.10 Several research groups, including Zhang,11 Yu,12 Zhao,13 Li,14 Wang15 and Yang16 were developed different strategies for functionalizing quinoxalin-2(1H)-ones, which are well documented in the current literature.
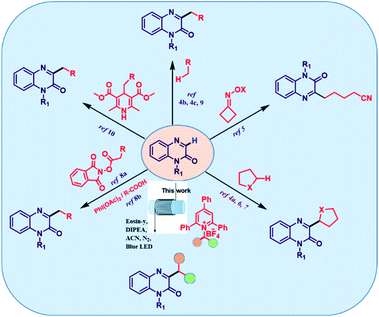 |
| Scheme 1 C-3-alkylation of quinoxalin-2(1H)-one derivatives in the reported works and this work. | |
Despite these elegant achievements, several drawbacks still remain in the previous reports including expensive starting materials, longer reaction times, limited substrate scope and use of transition metal catalysts. The ester alkyl groups cannot be introduced into quinoxalin-2(1H)-one framework through these methods. Thus it is also highly desirable to develop a novel, environmentally benign, more sustainable and metal free pathway to access C-3-functionalized quinoxalin-2(1H)-one frameworks to further promote its application in drug discovery and development. On the other hand, the generation of alkyl radical precursors from naturally and widely abundant, inexpensive alkyl amine derivatives17 via cleavage of C–N bond are less far investigated because of high bond dissociation energy and high stability of inactivated C–N bonds.18 In 1980's, the bench-stable Katritzky salts19 discovered by Katritzky have become an alternative and effective tool for the generation of alkyl radical precursors through a single-electron reduction and fragmentation via de-aminative pathway. Watson20 and other research groups have demonstrated the utility of these redox-active amines as sources of alkyl radical precursors in C–H arylation,21 borylation,22 alkynylation,23 allylation24 and dicarbofunctionalization.25
Photoredox catalysis has emerged as a flexible and powerful platform for the manufacture of radical reactions via single-electron-transfer (SET) process under mild reaction conditions.26 To promote the organic transformations in a clean, inexpensive, renewable pathway and as a part of our continuing investigations with the visible-light photoredox organic reactions,27 we herein disclose a novel, environmentally benign, metal-free and continuous-flow photoredox protocol to access C-3-alkylated quinoxalin-2(1H)-ones via C–N bond cleavage of amine/amino acid derived Katritzky salts with low catalyst loading under mild reaction conditions.
Accordingly, our initial investigation commenced with quinoxalin-2(1H)-ones (1) and alkyl amine derived Katritzky salt (2a) as the model reaction substrates by using rosebengal (2 mol%) as a photocatalyst (Table 1, entry 1). The reaction was conducted in CH3CN solvent, N2 atmosphere with 3 W blue LED lamp irradiation at room temperature. To our delight, the desired product 3a was obtained in 28% yield after 16 h (Table 1, entry 1). There was no further increase in the yield of the product when the reaction time was further prolonged. The structure of 3a was characterized by 1H and 13C NMR and mass spectroscopic studies. Encouraged by this result, a series of photocatalysts such as eosin-y, Na2-eosin-y, rhodamine B, fluorescein, TiO2, Ir(ppy)3 and acridine red were examined to enhance the yield of the reaction (Table 1, entries 2–8). Among the above photocatalysts examined, eosin-y was found to demonstrate the highest catalytic activity leading to the desired alkylated product 3a in 43% yield (Table 1, entry 8), which may be accredited to stronger absorption capability of eosin-y in the range of blue light. An improved yield of 3a was observed with an additional base i.e., Na2CO3 (Table 1, entry 9). This prompted us to investigate the effect of various bases such as Cs2CO3 in dimethylformamide, 2,6-lutidine, K2CO3 and DBU were in dimethylsulfoxide, DIPEA in acetonitrile and it was found that 3.0 equiv. of DIPEA gave the best result (Table 1, entries 9–14).
Table 1 Optimization of reaction conditions in batch for alkylation of quinoxalin-2(1H)-ones via C–N bond scission of amine/amino acid derived Katritzky saltsa
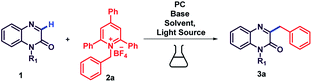
|
Entry |
Photocatalyst |
Base (equiv.) |
Solvent |
Yield (%)b |
Standard reaction conditions: 1 (1 mmol), 2a (1 mmol), catalyst 2 mol%, base (3.0 equiv), solvent (3 mL), stirred for 16 h. 3 W Blue LED light kept at a distance of 3 cm from the reaction flask. Isolated yields. catalyst 3 mol%. Catalyst 1 mol%. Catalyst was not used. The reaction was carried out with white LED light source. The reaction was carried out with green LED light source. No light. 2 mL of ACN was used. 4 mL of ACN was used. The reaction was carried out in an open atmosphere. N.D. = product 3a was not detected on TLC. DBU = 1, 8-diazabicyclo [5.4.0] undec-7-ene, DIPEA = N, N-diisopropylethylamine. All the reactions were performed in the nitrogen atmosphere. |
1 |
Rose bengal |
— |
ACN |
28 |
2 |
Tio2 |
— |
ACN |
13 |
3 |
Fluorescein |
— |
ACN |
30 |
4 |
Ir(ppy)3 |
— |
ACN |
10 |
5 |
Acridine red |
— |
ACN |
21 |
6 |
Na2-Eosin-y |
— |
ACN |
33 |
7 |
Rhodamine B |
— |
ACN |
26 |
8 |
Eosin-y |
— |
ACN |
43 |
9 |
Eosin-y |
Na2CO3 (2.0) |
ACN |
58 |
10 |
Eosin-y |
Cs2CO3 (2.0) |
DMF |
53 |
11 |
Eosin-y |
2,6-Lutidine (2.0) |
DMSO |
52 |
12 |
Eosin-y |
K2CO3 (3.0) |
DMSO |
51 |
13 |
Eosin-y |
DBU (2.0) |
DMSO |
56 |
14 |
Eosin-y |
DIPEA (2.0) |
ACN |
65 |
15 |
Eosin-y |
DIPEA (3.0) |
ACN |
72 |
16 |
Eosin-y |
DIPEA (4.0) |
ACN |
71 |
17c |
Eosin-y |
DIPEA (3.0) |
ACN |
71 |
18d |
Eosin-y |
DIPEA (3.0) |
ACN |
60 |
19e |
— |
DIPEA (3.0) |
ACN |
N.D. |
20f |
Eosin-y |
DIPEA (3.0) |
ACN |
60 |
21g |
Eosin-y |
DIPEA (3.0) |
ACN |
57 |
22h |
Eosin-y |
DIPEA (3.0) |
ACN |
N.D. |
23i |
Eosin-y |
DIPEA (3.0) |
ACN |
67 |
24j |
Eosin-y |
DIPEA (3.0) |
ACN |
72 |
25k |
Eosin-y |
DIPEA (3.0) |
ACN |
51 |
3a could be obtained in 72% yield when 2.0 mol% of eosin-y was used (Table 1, entry 15). Changing the amount of catalyst used also did improve the yields further (Table 1, entries 17 and 18). Notably, in the absence of photocatalyst or light (Table 1, entry 19 & 22) no product formation was observed. Further experiment results revealed that the yield of 3a did not increase when the source of light was changed to white LED or green LED (Table 1, entries 20 & 21). And also investigated the reaction with different concentrations (Table 1, entry 23 and 24). In addition, low yield was observed when the reaction was conducted in an open atmosphere (Table 1, entry 25). Finally, the best yield (72%) was achieved by using 2 mol% of eosin-y as a catalyst and 3.0 equiv. of DIPEA as a base at room temperature under the irradiation of 3 W blue LED in nitrogen atmosphere for 16 h (for details, see ESI Scheme S4†).
Under the optimized conditions, we then turned our attention to investigate the substrate scope of C-3-alkylation of quinoxalin-2(1H)-ones via C–N bond cleavage of amine derived Katritzky salts under eosin-y catalyzed photoredox protocol and the results are summarized in Table 2. At the beginning, we evaluated the substrate scope with respect to quinoxalin-2(1H)-ones. Quinoxalin-2(1H)-ones bearing electron-withdrawing (–Fluoro) as well as electron-donating (–Methyl) substituents at 6 and 7 positions on phenyl ring underwent smoothly and leading to the desired alkylated products in excellent yields (Table 2, entries 3a–3e). Furthermore, quinoxalin-2(1H)-ones bearing N-protecting groups such as alkynes, ketones and esters were also successfully converted to the corresponding alkylated products in good yields (Table 2, entries 3f–3h). We then evaluated the scope of this C-3-alkylation of quinoxalin-2(1H)-ones with respect to amine derived Katritzky salts under our optimized conditions. It was found that this reaction proceeded smoothly with Katritzky salts bearing the benzyl groups with either electron-withdrawing and electron-donating substituents at ortho, para and meta positions on the phenyl ring and deliver the alkylated derivatives in good to excellent yields (Table 2, entries 3i–3w, 3ab, 3ac). This also indicated that there is no effect of steric hindrance on this reaction. It is worth mention that the hetero aromatic ring substituent was found to be well tolerated affording the desired product in satisfactory yield (Table 2, entries 3x). The reaction did not work well with the corresponding Katritzky salts derived from cyclohexyl amine when quinoxalin-2(1H)-ones protected with benzyl and methyl groups (Table 2, entries 3y–3z). We attempted the reaction with N-free quinoxalin-2(1H)-one, but it was unsuccessful. This may be due to the extraction of proton from the N-free quinoxalin-2(1H)-one in presence of base. In the case of phenyl amine derived Katritzky salt, we are unable to get the desired product. Electron withdrawing groups such nitro, cyano groups attached quinoxalin-2(1H)-ones failed to give the desired alkylated products. We then focused on the scope of Katritzky salts, which have been synthesized from the corresponding amino acid derivatives and the results showed that this reaction was well compatible and furnished the desired alkylated products in moderate to good yields (Table 3, entries 4a–4d). In addition to this, quinoxalin-2(1H)-ones derived from natural products such as o-vanillin and vanillin afforded the corresponding products in excellent yields (Table 3, entries 4e–4f).
Table 2 Substrate scope in batch reactor with respect to amine derived Katritzky saltsa
Standard reaction conditions: all reactions were carried out on 0.3 mmol of 1a and 0.3 mmol of 2a and 3.0 equiv. of DIPEA and 2 mol% of eosin-y in 3 mL of CH3CN. Isolated yields of chromatographically pure products. |
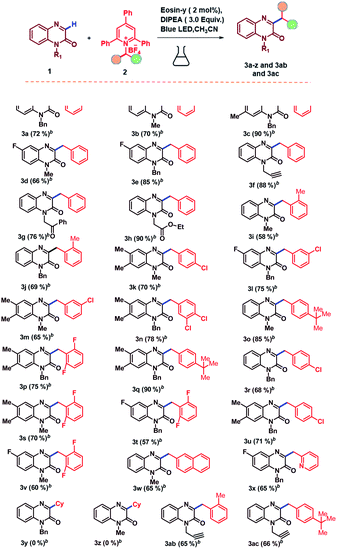 |
Table 3 Substrate scope in batch reactor with respect to in batch reactor with respect to amino acid derived Katritzky salts (4a–4d) and some complex molecules (4e–4f) and secondary alkyl amine derived Katritzky salts(4h–4n)a
Standard reaction conditions: all reactions were carried out on 0.3 mmol of 1a and 0.3 mmol of 2a and 3.0 equiv. of DIPEA and 2 mol% of eosin-y in 3 mL of CH3CN. Isolated yields of chromatographically pure products. |
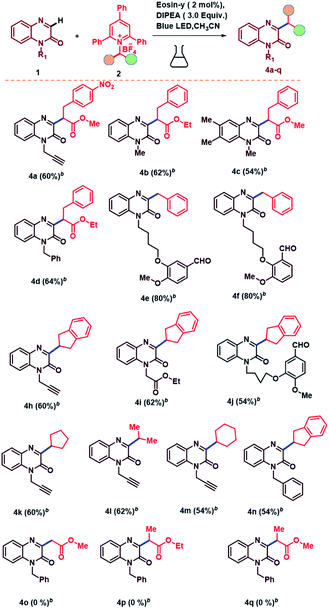 |
Remarkably, Katritzky salts derived from a broad range of unactivated secondary alkyl amines such as cyclohexyl, cyclopentyl, 2,3-dihydro-1H-indene and isopropyl functionalities were compatible under the standard reaction conditions and leading to the desired alkylated products in good to excellent yields (Table 3, entries 4h–4n). In the case of different amine acid derived Katritzky salts, we were unable to obtain the desired alkylated products; a clear conversation of Katritzky salt emerged on TLC to its by product, but the starting quinoxalin-2(1H)-ones were not converted into desired alkylated products (Table 3, entries 4o–4q). 4-Methoxy benzyl amine derived Katritzky salts failed to offer the desired alkylated product, this may be due to electronic effect of the substituent. While in the case of tertiary amines, we are unable to synthesize the Katritzky salts.
Continuous-flow reaction
During the last decade, continuous-flow reactions have gained significant attention from the researchers in academia and industry due to increased productivity, selectivity, safety, yield and scale-up possibilities.28 Particularly the usage of micro reactors in photochemical reactions have emerged as alternatives to batch chemistry because of their significant features such as very high surface to volume ratio, high heat and mass transfer efficiency and enhanced illumination homogeneity.29 Considering these advantages, a continuous-flow protocol for the photoredox catalysed C-3 alkylation of quinoxalin-2(1H)-ones was established. The optimal conditions established by batch process were directly translated to a continuous-flow microfluidic reactor and investigated. To this end, a continuous-flow set up was arranged by employing two syringes, T-mixer, syringe pump and PFA (id = 500 μm, length = 2.5 m) capillary micro reactor. Initially, the amine/amino acid derived Katritzky salt and quinoxalin-2(1H)-ones, photocatalyst, base in CH3CN solvent under nitrogen atmosphere were introduced through two 5 mL NORM-JECT plastic syringes and introduced into the photo micro reactor through a syringe pump which were passing through T-mixer and further passed through visible light transparent PFA capillary tubing wrapped over a glass test tube and this capillary wrapped test tube was exposed to irradiation with blue LED light source (For details, see ESI Scheme S5†). Schematic illustration of the photochemical reaction in flow micro reactor is depicted in Table 4. By employing different flow rates, the efficiency of the reaction was monitored over PFA capillary based micro reactor at various residence times (Table 4). Gratifyingly, full conversion of 1a was observed within 0.81 min residence time with flow a rate of 300 μL min−1. Significant progress in the improvement of the yield of the desired product 3a (91%) was also obtained when compared to the batch reaction (72%) that took very long reaction time (16 h).
Table 4 Effect of retention time on photocatalytic de-aminative radical alkylation of amine/amino acid derived Katritzky salts with quinoxalin-2(1H)-ones in continuous-flow microreactora
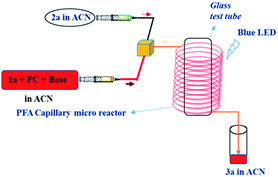
|
Entry |
Flow rate (μLmin−1) |
Residence time (min) |
Yield (%)b |
2a |
1a + PC + Base |
Standard reaction conditions: all reactions were carried out on 0.3 mmol of 1a and 0.3 mmol of 2a and 3.0 equiv. of DIPEA and 2 mol% of eosin-y in 3 mL of CH3CN. Isolated yields of chromatographically pure products. PFA microreactor with serpentine microchannel (length 2.5 meter, width 500 μm)a. |
1 |
100 |
100 |
2.45 |
86 |
2 |
200 |
200 |
1.22 |
84 |
3 |
250 |
250 |
0.98 |
91 |
4 |
300 |
300 |
0.81 |
91 |
5 |
350 |
350 |
0.70 |
79 |
6 |
400 |
400 |
0.61 |
65 |
7 |
500 |
500 |
0.49 |
43 |
This indicates that the narrow channel of the micro reactor provides opportunity to enhance uniform irradiation of the complete reaction mixture resulting in improved yield along with shorter reaction time. To prove the prospective applicability of the reaction, a gram scale reaction was carried out under standard optimized reaction conditions. Notably, the scalability procedure was established using 5 mmol of 1a, 5 mmol of 2a and PFA tubing (id = 500 μm, length = 8 m) under continuous-flow conditions over blue LED irradiation and resulting in 80% yield of 3h (1.120 g). In addition, we also employed our protocol for the synthesis of antidiabetic drug candidate30 4g by further transformation of 3h as shown in Scheme 2.
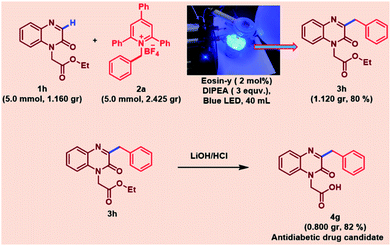 |
| Scheme 2 Scale-Up experiment and synthetic application. | |
To unambiguously elucidate into the mechanistic details of the present C-3-alkylation of quinoxalin-2(1H)-ones induced by photocatalytic protocol, a few control experiments were carried out in batch condition as shown in Scheme 3.
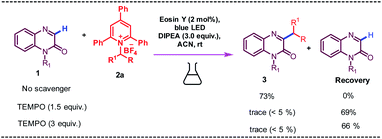 |
| Scheme 3 Control experiments. | |
The detrimental effect on reaction output was observed when we introduced 1.5 equiv. or 3 equiv. of 2,2,6,6-tetramethyl-1-piperidinyloxy (TEMPO) into the reaction mixture as a radical-trapping agent because of the formation of alkylated TEMPO product (detected by Mass, See ESI for details, Fig. S2†) and a considerable amount of starting material was recovered. These results clearly implied that the alkylation process might proceed through a radical pathway. To know the quenching property, we have conducted the UV-vis and photoluminescence studies (see ESI for details, Fig. S3†). As expected, the intensity of excited state of eosin-y could be gradually quenched with the increasing concentration of 2a. This suggests that an energy transfer process exists between the eosin-y & 2a and resulted amine/amino acid derived Katritzky salt (2a) could quench the photoexcited eosin-y effectively.
Based on these controlled experiments and related literature reports,6,23,31 a plausible reaction mechanism for this transformation is illustrated in Scheme 4. Under the irradiation of blue LED's, the photocatalyst eosin-y will be reversibly converted into its excited state and generate the eosin-y* species. Subsequently a single electron transfer (SET) from eosin-y* species to 2a affords pyridyl radical A, which further undergoes fragmentation to give alkyl radical C and a rearomatized pyridine B with the generation of eosin Y˙+ complex.
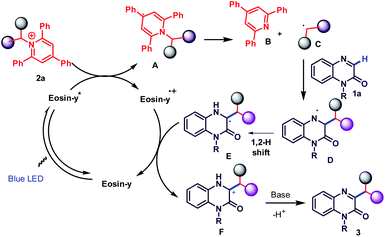 |
| Scheme 4 Plausible mechanistic pathway. | |
The alkyl radical species C adds on to C-3 position of quinoxalin-2(1H)-one(1a), thus affording a nitrogen radical intermediate D, which undergo 1,2-hydrogen shift to generate carbon radical E. This intermediate E is oxidized by eosin-y˙+ to produce the carbon cation intermediate F through a SET manner to complete the redox cycle. Finally, the resulting cation F undergo deprotonation in the presence of base to deliver the desired alkylated product 3.
Conclusions
In conclusion, we have developed a new, environmental benign, visible-light-induced continuous-flow pathway for the C-3-alkylation of quinoxalin-2(1H)-one derivatives. Redox-active pyridinium salts derived from abundant and inexpensive alkyl amines (both primary and secondary) and few of amino acids were used as alkyl radical precursors for the construction of C-3-alkylated quinoxalin-2(1H)-one derivatives in the presence of eosin-y catalyst under visible light irradiation at room temperature. Moreover, this sustainable pathway was further carried out in continuous-flow conditions using PFA capillary micro reactor that probed the efficiency with higher yields and shorter duration of reaction time i.e., 0.81 min residence time compared to the batch condition that took 16 h with slightly little lower yields. A scale-up experiment was successfully demonstrated by utilizing PFA capillary tube micro reactor. Compared to the previous methods, the present novel protocol has some advantages including simple operation, fast reaction in continuous-flow manner, mild reaction condition and scalability which makes this method a promising tool for the synthesis of natural products and diverse bio-active molecules.
Conflicts of interest
There are no conflicts to declare.
Acknowledgements
G. K., V. R. and V. R. thanks CSIR for fellowships. We thank CSIR for financial support (Ref. no. 34/1/TD-CLP/NCP-FBR 2020-RPPBDD-TMD−Se-MI). We gratefully acknowledge Director, CSIR-IICT for his support and encouragement. CSIR-IICT manuscript communication no. IICT/Pubs./2021/218.
Notes and references
-
(a) A. Carta, S. Piras, G. Loriga and G. Paglietti, Mini-Rev. Med. Chem., 2006, 6, 1179–1200 CrossRef CAS PubMed;
(b) R. Liu, Z.-H. Huang, M. G. Murray, X.-Y. Guo and G. Liu, J. Med. Chem., 2011, 54, 5747–5768 CrossRef CAS PubMed;
(c) S. A. Galal, S. H. M. Khairat, F. A. F. Ragab, A. S. Abdelsamie, M. M. Ali, S. M. Sliman, J. Mortier, G. Wolber and H. El Diwani, Eur. J. Med. Chem., 2014, 86, 122–132 CrossRef CAS PubMed;
(d) X. Qin, X. Hao, H. Han, S. Zhu, Y. Yang, B. Wu, S. Hussain, S. Parveen, C. Jing, B. Ma and C. Zhu, J. Med. Chem., 2015, 58, 1254–1267 CrossRef CAS PubMed.
-
(a) N. Udilova, A. V. Kozlov, W. Bieberschulte, K. Frei, K. Ehrenberger and H. Nohl, Biochem. Pharmacol., 2003, 65, 59–65 CrossRef CAS PubMed;
(b) J. Dudash, Y. Zhang, J. B. Moore, R. Look, Y. Liang, M. P. Beavers, B. R. Conway, P. J. Rybczynski and K. T. Demarest, Bioorg. Med. Chem. Lett., 2005, 15, 4790–4793 CrossRef CAS PubMed;
(c) A. A. Abu-Hashem, M. A. Gouda and F. A. Badria, Eur. J. Med. Chem., 2010, 45, 1976–1981 CrossRef CAS PubMed;
(d) S. Hussain, S. Parveen, X. Hao, S. Zhang, W. Wang, X. Qin, Y. Yang, X. Chen, S. Zhu, C. Zhu and B. Ma, Eur. J. Med. Chem., 2014, 80, 383–392 CrossRef CAS PubMed;
(e) D. A. E. Issa, N. S. Habib and A. E. Abdel Wahab, MedChemComm, 2015, 6, 202–211 RSC.
-
(a) A. Carrër, J. D. Brion, S. Messaoudi and M. Alami, Org. Lett., 2013, 15, 5606–5609 CrossRef PubMed;
(b) K. Yin and R. Zhang, Org. Lett., 2017, 19, 1530–1533 CrossRef CAS PubMed;
(c) J. Yuan, S. Liu and L. Qu, Adv. Synth. Catal., 2017, 359, 4197–4207 CrossRef CAS;
(d) S. Paul, J. H. Ha, G. E. Park and Y. R. Lee, Adv. Synth. Catal., 2017, 359, 1515–1521 CrossRef CAS;
(e) X. Zeng, C. Liu, X. Wang, J. Zhang, X. Wang and Y. Hu, Org. Biomol. Chem., 2017, 15, 8929–8935 RSC;
(f) J.-W. Yuan, J.-H. Fu, S.-N. Liu, Y.-M. Xiao, P. Mao and L.-B. Qu, Org. Biomol. Chem., 2018, 16, 3203–3212 RSC;
(g) Y. Kim and D. Y. Kim, Tetrahedron Lett., 2018, 59, 2443–2446 CrossRef CAS;
(h) M. Gao, Y. Li, L. Xie, R. Chauvin and X. Cui, Chem. Commun., 2016, 52, 2846–2849 RSC;
(i) L.-Y. Xie, Y.-L. Chen, L. Qin, Y. Wen, J.-W. Xie, J.-X. Tan, Y. Huang, Z. Cao and W.-M. He, Org. Chem. Front., 2019, 6, 3950–3955 RSC;
(j) J. Zhou, P. Zhou, T. Zhao, Q. Ren and J. Li, Adv. Synth. Catal., 2019, 361, 5371–5382 CrossRef CAS;
(k) T. T. Hoang, T. A. To, V. T. T. Cao, A. T. Nguyen, T. T. Nguyen and N. T. S. Phan, Catal. Commun., 2017, 101, 20–25 CrossRef CAS;
(l) W. Wei, L. Wang, P. Bao, Y. Shao, H. Yue, D. Yang, X. Yang, X. Zhao and H. Wang, Org. Lett., 2018, 20, 7125–7130 CrossRef CAS PubMed;
(m) K.-J. Li, K. Xu, Y.-G. Liu, C.-C. Zeng and B.-G. Sun, Adv. Synth. Catal., 2019, 361, 1033–1041 CrossRef CAS;
(n) J. Yuan, J. Zhu, J. Fu, L. Yang, Y. Xiao, P. Mao, X. Du and L. Qu, Org. Chem. Front., 2019, 6, 925–935 RSC;
(o) L. Wang, Y. Zhang, F. Li, X. Hao, H.-Y. Zhang and J. Zhao, Adv. Synth. Catal., 2018, 360, 3969–3977 CrossRef CAS;
(p) M. Sun, L. Wang, L. Zhao, Z. Wang and P. Li, ChemCatChem, 2020, 12, 5261–5268 CrossRef CAS.
-
(a) L. Q. Hu, J. W. Yuan, J. H. Fu, T. T. Zhang, L. L. Gao, Y. M. Xiao, P. Mao and L. B. Qu, Eur. J. Org. Chem., 2018, 4113–4120 CrossRef CAS;
(b) J. Yuan, J. Fu, J. Yin, Z. Dong, Y. Xiao, P. Mao and L. Qu, Org. Chem. Front., 2018, 5, 2820–2828 RSC;
(c) J. Fu, J. Yuan, Y. Zhang, Y. Xiao, P. Mao, X. Diao and L. Qu, Org. Chem. Front., 2018, 5, 3382–3390 RSC.
-
(a) L. Yang, P. Gao, X.-H. Duan, Y.-R. Gu and L.-N. Guo, Org. Lett., 2018, 20, 1034–1037 CrossRef CAS PubMed;
(b) W. Zhang, Y.-L. Pan, C. Yang, L. Chen, X. Li and J.-P. Cheng, J. Org. Chem., 2019, 84, 7786–7795 CrossRef CAS PubMed;
(c) P.-J. Xia, Y.-Z. Hu, Z.-P. Ye, X.-J. Li, H.-Y. Xiang and H. Yang, J. Org. Chem., 2020, 85, 3538–3547 CrossRef CAS PubMed.
-
(a) W. Wei, L. Wang, H. Yue, P. Bao, W. Liu, C. Hu, D. Yang and H. Wang, ACS Sustainable Chem. Eng., 2018, 6, 17252–17257 CrossRef CAS;
(b) N. Meng, Y. Lv, Q. Liu, R. Liu, X. Zhao and W. Wei, Chin. Chem. Lett., 2021, 32, 258–262 CrossRef CAS.
- K. D. Mane, R. B. Kamble and G. Suryavanshi, New J. Chem., 2019, 43, 7403–7408 RSC.
-
(a) Z. Yan, B. Sun, X. Zhang, X. Zhuang, J. Yang, W. Su and C. Jin, Chem.–Asian J., 2019, 14, 3344–3349 CrossRef CAS PubMed;
(b) L.-Y. Xie, L.-L. Jiang, J.-X. Tan, Y. Wang, X.-Q. Xu, B. Zhang, Z. Cao and W.-M. He, ACS Sustainable Chem. Eng., 2019, 7, 14153–14160 CrossRef CAS;
(c) K. Sun, F. Xiao, B. Yu and W. -M. He, Chin. J. Catal., 2021, 42, 1921–1943 CrossRef CAS;
(d) Y. Zhang, H. Luo, H. Ma, J. Wan, Y. Ji, A. Shaginian, J. Li, Y. Deng and G. Liu, Bioconjugate Chem., 2021, 32, 1576–1580 CrossRef CAS PubMed;
(e) L.-Y. Xie, S. Peng, L.-H. Yang, C. Peng, Y.-W. Lin, X. Yu, Z. Cao, Y.-Y. Peng and W.-M. He, Green Chem., 2021, 23, 374–378 RSC;
(f) L.-Y. Xie, Y.-S. Bai, X.-Q. Xu, X. Peng, H.-S. Tang, Y. Huang, Y.-W. Lin, Z. Cao and W.-M. He, Green Chem., 2020, 22, 1720–1725 RSC.
- S. Singh, N. Dagar and S. R. Roy, Org. Biomol. Chem., 2021, 19, 5383–5394 RSC.
- X.-K. He, J. Lu, A.-J. Zhang, Q.-Q. Zhang, G.-Y. Xu and J. Xuan, Org. Lett., 2020, 22, 5984–5989 CrossRef CAS PubMed.
-
(a) J. Shen, J. Xu, L. Huang, Q. Zhu and P. Zhang, Adv. Synth. Catal., 2020, 362, 230–241 CrossRef CAS;
(b) J. Xu, H. Yang, L. He, L. Huang, J. Shen, W. Li and P. Zhang, Org. Lett., 2021, 23, 195–201 CrossRef CAS PubMed;
(c) J. Xu, L. Huang, L. He, Z.-G. Ni, J.-B. Shen, X.-L. Li, K.-X. Chen, W.-M. Li and P.-F. Zhang, Green Chem., 2021, 23, 2123–2129 RSC;
(d) J. Xu, L. He, C. Liang, X. Yue, Y. Ouyang and P. Zhang, ACS Sustainable Chem. Eng., 2021, 9, 13663–13671 CrossRef CAS.
-
(a) Y.-F. Si, K. Sun, X.-L. Chen, X.-Y. Fu, Y. Liu, F.-L. Zeng, T. Shi, L.-B. Qu and B. Yu, Org. Lett., 2020, 22, 6960–6965 CrossRef CAS PubMed;
(b) T. Shi, K. Sun, X.-L. Chen, Z.-X. Zhang, X.-Q. Huang, Y.-Y. Peng, L.-B. Qu and B. Yu, Adv. Synth. Catal., 2020, 362, 2143–2149 CrossRef.
- H.-C. Ni, X.-Z. Shi, Y. Li, X.-N. Zhang, J.-W. Zhao and F. Zhao, Org. Biomol. Chem., 2020, 18, 6558–6563 RSC.
-
(a) J. Xu, H. Zhang, J. Zhao, J. Ni, P. Zhang, B.-F. Shi and W. Li, Org. Chem. Front., 2020, 7, 4031–4042 RSC;
(b) J. Zhou, Q. Ren, N. Xu, C. Wang, S. Song, Z. Chen and J. Li, Green Chem., 2021, 23, 5753–5758 RSC;
(c) J. Xu, L. Huang, L. He, C. Liang, Y. Ouyang, J. Shen, M. Jiang and W. Li, Green Chem., 2021, 23, 6632–6638 RSC;
(d) J. Shen, J. Xu, L. He, C. Liang, Chin. Chem. Lett., 2022, 33, 1227–1235 Search PubMed;
(e) H. Zhang, J. Xu, Y. Ouyang, X. Yue, C. Zhou, Z. Ni, W. Li, Chin. Chem. Lett., DOI:10.1016/j.cclet.2021.09.069;
(f) L. Yao, D. Zhu, L. Wang, J. Liu, Y. Zhang, P. Li, Chin. Chem. Lett., 2021, 32, 4033–4037 Search PubMed.
-
(a) K. Niu, Y. Hao, L. Song, Y. Liu and Q. Wang, Green Chem., 2021, 23, 302–306 RSC;
(b) K.-K. Niu, L. Ding, P. Zhou, Y.-K. Hao, Y.-X. Liu, H.-J. Song and Q.-M. Wang, Green Chem., 2021, 23, 3246–3249 RSC.
-
(a) F. Liu, Z.-P. Ye, Y.-Z. Hu, J. Gao, L. Zheng, K. Chen, H.-Y. Xiang, X.-Q. Chen and H. Yang, J. Org. Chem., 2021, 86, 11905–11914 CrossRef CAS PubMed;
(b) Z.-P. Ye, F. Liu, X.-Y. Duan, J. Gao, J.-P. Guan, J.-A. Xiao, H.-Y. Xiang, K. Chen and H. Yang, J. Org. Chem., 2021, 86(23), 17173–17183 CrossRef CAS PubMed.
-
(a) S. A. Lawrence, Amines: Synthesis, Properties and Applications, Cambridge University Press, New York, 2004 Search PubMed;
(b) T. C. Nugent, Chiral Amine Synthesis, Wiley-VCH Verlag GmbH & Co. KGaA, Weinheim, Germany, 2010 CrossRef.
-
(a) K. Ouyang, W. Hao, W.-X. Zhang and Z. Xi, Chem. Rev., 2015, 115, 12045–12090 CrossRef CAS PubMed;
(b) D. Kong, P. J. Moon and R. J. Lundgren, Nat. Catal., 2019, 2, 473–476 CrossRef CAS.
-
(a) A. R. Katritzky, U. Gruntz, D. H. Kenny, M. C. Rezende and H. Sheikh, J. Chem. Soc., Perkin Trans., 1979, 1, 430–432 RSC;
(b) A. R. Katritzky and C. M. Marson, Angew. Chem., Int. Ed., 1984, 23, 420–429 CrossRef;
(c) S. Sowmiah, J. M. S. S. Esperanaa, L. P. N. Rebelo and C. A. M. Afonso, Org. Chem. Front., 2018, 5, 453–493 RSC.
-
(a) C. H. Basch, J. Liao, J. Xu, J. J. Piane and M. P. Watson, J. Am. Chem. Soc., 2017, 139, 5313–5316 CrossRef CAS PubMed;
(b) S. Plunkett, C. H. Basch, S. O. Santana and M. P. Watson, J. Am. Chem. Soc., 2019, 141(6), 2257–2262 CrossRef CAS PubMed.
- F. J. R. Klauck, M. J. James and F. Glorius, Angew. Chem., Int. Ed., 2017, 56, 12336–12339 CrossRef CAS PubMed.
-
(a) J. Wu, L. He, A. Noble and V. K. Aggarwal, J. Am. Chem. Soc., 2018, 140(34), 10700–10704 CrossRef CAS PubMed;
(b) J. Hu, G. Wang, S. Li and Z. Shi, Angew. Chem., Int. Ed., 2018, 57, 15227–15231 CrossRef CAS PubMed;
(c) F. Sandfort, F. Strieth-Kalthoff, F. J. R. Klauck, M. J. James and F. Glorius, Chem.–Eur. J., 2018, 24, 17210–17214 CrossRef CAS PubMed.
- M. Ociepa, J. Turkowska and D. Gryko, ACS Catal., 2018, 8(12), 11362–11367 CrossRef CAS.
- M.-M. Zhang and F. Liu, Org. Chem. Front., 2018, 5, 3443–3446 RSC.
-
(a) F. J. R. Klauck, H. Yoon, M.
J. James, M. Lautens and F. Glorius, ACS Catal., 2019, 9(1), 236–241 CrossRef CAS;
(b) J. Wu, P. S. Grant, X. Li, A. Noble and V. K. Aggarwal, Angew. Chem., Int. Ed., 2019, 58, 5697–5701 CrossRef CAS PubMed;
(c) X. Jiang, M.-M. Zhang, W. Xiong, L.-Q. Lu and W.-J. Xiao, Angew. Chem., Int. Ed., 2019, 58, 2402–2406 CrossRef CAS PubMed.
-
(a) T. P. Yoon, M. A. Ischay and J. N. Du, Nat. Chem., 2010, 2, 527–532 CrossRef CAS PubMed;
(b) J. M. R. Narayanam and C. R. J. Stephenson, Chem. Soc. Rev., 2011, 40, 102–113 RSC;
(c) S. Fukuzumi and K. Ohkubo, Org. Biomol. Chem., 2014, 12, 6059–6071 RSC;
(d) N. A. Romero and D. A. Nicewicz, Chem. Rev., 2016, 116(17), 10075–10166 CrossRef CAS PubMed;
(e) M. H. Shaw, J. Twilton and D. W. C. MacMillan, J. Org. Chem., 2016, 81, 6898–6926 CrossRef CAS PubMed.
-
(a) P. R. Adiyala, K. N. V. Sastry, J. Kovvuri, A. Nagarajan, V. G. Reddy, I. B. Sayeed, V. L. Nayak, R. A. Maurya and A. Kamal, ChemistrySelect, 2017, 2, 8158–8161 CrossRef CAS;
(b) P. R. Adiyala, S. Jang, N. K. Vishwakarma, Y.-H. Hwang and D.-P. Kim, Green Chem., 2020, 22, 1565–1571 RSC;
(c) V. Ramesh, M. Gangadhar, J. B. Nanubolu and P. R. Adiyala, J. Org. Chem., 2021, 86(18), 12908–12921 CrossRef CAS PubMed.
-
(a) B. Gutmann, D. Cantillo and C. O. Kappe, Angew. Chem., Int. Ed., 2015, 54, 6688–6728 CrossRef CAS PubMed;
(b) H. Lin, C. Dai, T. F. Jamison and K. F. Jensen, Angew. Chem., Int. Ed., 2017, 56, 8870–8873 CrossRef CAS PubMed;
(c) V.-E. H. Kassin, R. Gerardy, T. Toupy, D. Collin, E. Salvadeo, F. Toussaint, K. Van Hecke and J.-C. M. Monbaliu, Green Chem., 2019, 21, 2952–2966 RSC;
(d) P. Zhang, N. Weeranoppanant, D. A. Thomas, K. Tahara, T. Stelzer, M. G. Russell, M. O'Mahony, A. S. Myerson, H. Lin, L. P. Kelly, K. F. Jensen, T. F. Jamison, C. Dai, Y. Cui, N. Briggs, R. L. Beingessner and A. Adamo, Chem.–Eur. J., 2018, 24(11), 2776–2784 CrossRef CAS PubMed.
-
(a) C. Sambiagio and T. Noël, Trends Chem., 2020, 2, 92–106 CrossRef CAS;
(b) J. W. Tucker, Y. Zhang, T. F. Jamison and C. R. J. Stephenson, Angew. Chem., Int. Ed., 2012, 124(17), 4220–4223 CrossRef;
(c) Y. Su, N. J. W. Straathof, V. Hessel and T. Noel, Chem.–Eur. J., 2014, 20, 10562–10589 CrossRef CAS PubMed;
(d) D. Cambié, C. Bottecchia, N. J. W. Straathof, V. Hessel and T. Noël, Chem. Rev., 2016, 116, 10276–10341 CrossRef PubMed;
(e) R. Grainger, T. D. Heightman, S. V. Ley, F. Lima and C. N. Johnson, Chem. Sci., 2019, 10, 2264–2271 RSC;
(f) C. Sambiagio, M. Ferrari, K. van Beurden, N. d. Ca', J. van Schijndel and T. Noël, Org. Lett., 2021, 23, 2042–2047 CrossRef CAS PubMed;
(g) T. Wan, L. Capaldo, G. Laudadio, A. Nyuchev, J. Rincon, P. Garcia-Losada, C. Mateos, M. O. Frederick, M. Nuno and T. Noel, Angew. Chem., Int. Ed., 2021, 60, 17893–17897 CrossRef CAS PubMed.
- B. Wu, Y. Yang, X. g. Qin, S. Zhang, C. Jing, C. Zhu and B. Ma, ChemMedChem, 2013, 8, 1913–1917 CrossRef CAS PubMed.
-
(a) Q. Ke, G. Yan, J. Yu and X. Wu, Org. Biomol. Chem., 2019, 17, 5863–5881 RSC;
(b) J. T. M. Correia, V. A. Fernandes, B. T. Matsuo, J. A. C. Delgado, W. C. de Souza and M. W. Paixao, Chem. Commun., 2020, 56, 503–514 RSC.
|
This journal is © The Royal Society of Chemistry 2022 |
Click here to see how this site uses Cookies. View our privacy policy here.