DOI:
10.1039/D1RA08454B
(Paper)
RSC Adv., 2022,
12, 16454-16478
NiII NPs entrapped within a matrix of L-glutamic acid cross-linked chitosan supported on magnetic carboxylic acid-functionalized multi-walled carbon nanotube: a new and efficient multi-task catalytic system for the green one-pot synthesis of diverse heterocyclic frameworks†
Received
18th November 2021
, Accepted 20th April 2022
First published on 7th June 2022
Abstract
In the present study, a new L-glutamic acid cross-linked chitosan supported on magnetic carboxylic acid-functionalized multi-walled carbon nanotube (Fe3O4/f-MWCNT-CS-Glu) nanocomposite was prepared through a convenient one-pot multi-component sequential strategy. Then, nickelII nanoparticles (NiII NPs) were entrapped within a matrix of the mentioned nanocomposite. Afterward, the structure of the as-prepared Fe3O4/f-MWCNT-CS-Glu/NiII nanosystem was elucidated by various techniques, including FT-IR, PXRD, SEM, TEM, SEM-based EDX and elemental mapping, ICP-OES, TGA/DTA, and VSM. In the next part of this research, the catalytic applications of the mentioned nickelII-containing magnetic nanocomposite were assessed upon green one-pot synthesis of diverse heterocyclic frameworks, including bis-coumarins (3a–n), 2-aryl(or heteroaryl)-2,3-dihydroquinazolin-4(1H)-ones (5a–r), 9-aryl-3,3,6,6-tetramethyl-3,4,5,6,7,9-hexahydro-1H-xanthene-1,8(2H)-diones (7a–n), and 2-amino-4-aryl-7,7-dimethyl-5-oxo-5,6,7,8-tetrahydro-4H-chromene-3-carbonitriles (9a–n). The good-to-excellent yields of the desired products, satisfactory reaction rates, use of water solvent or solvent-free reaction medium, acceptable turnover numbers (TONs) and turnover frequencies (TOFs), along with comfortable recoverability and satisfying reusability of the as-prepared nanocatalyst for at least eight successive runs, and also easy work-up and purification procedures are some of the advantages of the current synthetic protocols.
1. Introduction
The preparation of new catalytic systems has particular importance and a specific position in organic synthesis and green chemistry. In this regard, the use of non-hazardous, available, cost-effective, stable, and active platforms is receiving more attention. Chitosan (CS) as the only polycationic biopolymer, which is mostly obtained from the alkaline deacetylation of chitin, has been used in various areas, especially biomedicine,1 drug delivery,2 gene delivery,3 vaccine delivery,4 dentistry,5 tissue engineering,6 wound-healing dressing,7 water remediation,8 agriculture,9 food packaging,10 catalysis,11 and many others. The existence of numerous alcoholic, primary amine, acetamide, and ether functional groups in the chitosan structure causes this biocompatible complex hetero-biopolymer to be given special attention as an exclusive organic system in catalysis development. Due to having the mentioned functional groups, chitosan has been able to participate in advanced non-magnetic and magnetic catalytic structures such as metal–organic frameworks (MOFs),12 covalent organic frameworks (COFs),13 graphene oxide (GO)- and/or ionic liquid (IL)-containing systems,14,15 Schiff base-type platforms,16 micro-reactors,17 and so on. In recent years, the publication of numerous reports on the diverse and eye-catching applications of functionalized carbon nanotubes (CNTs), including single-walled carbon nanotubes (SWCNTs), double-walled carbon nanotubes (DWCNTs), and multi-walled carbon nanotubes (MWCNTs), especially in the field of catalysis, is a valid proof of the value of these carbon scaffolds.18 Interaction between polysaccharides (particularly chitosan) and CNTs can pave the way for the development of new catalytic platforms, especially creating a gigantic CNT-CS-containing matrix with a high ability to immobilize diverse metal nanoparticles, heteropoly acids (HPAs), etc. Also, the CNTs are capable of increasing some of the chitosan attributes, such as mechanical properties.19 On the other hand, one of the significant properties of a suitable catalyst from the green chemistry point of view is the capability of easy and complete separation from the reaction environment. For this purpose, the magnetic-type catalysts are an interesting choice because they can be separated from the reaction pot with an external magnetic field that allows avoiding time-consuming separation procedures.20
Heterocyclic frameworks are among the most significant molecules for everyday life.11a,21 They exist in the structure of various compounds such as drugs, natural products, bioactive substances, vitamins, veterinary products, and many others. Among the available synthetic methods for the heterocyclic scaffolds construction, one-pot multi-component reactions (MCRs) have been considered as a powerful and fantastic strategy owing to their short reaction times, simplicity, high selectivity, etc.22 Notably, the one-pot MCRs have been classified in the first branch of green chemistry principles, viz. prevent waste. It is worthy to note that the twelve principles of green chemistry have gained significant attention, both from academia and industries, and give us a guideline for designing safer and eco-friendly methods in chemical synthesis.23 In addition to catalysis and synthetic method, the environmentally benign reaction medium is another essential parameter in the green chemistry discipline. For this purpose, water is the most desirable choice because this medium is safe, environmentally friendly, readily available, and inexpensive compared to other organic solvents.24 On the other hand, solventless medium (well-known as solvent-free reaction conditions) is a great technique to perform reactions without using any solvents. The solvent-free method has many advantages rather than solution conditions, including high yields, short reaction times, simple reaction procedures and milder conditions, easy work-up and purification, high selectivity, minimization of by-products, and so on.25
In continuation of our ongoing research program in organic synthesis and catalyst design,26 we report herein a convenient two-step preparation method for the construction of a new gigantic multi-functional catalytic system through the entrapping of the nickelII nanoparticles into a matrix of L-glutamic acid cross-linked chitosan supported on magnetic carboxylic acid-functionalized multi-walled carbon nanotube (Fe3O4/f-MWCNT-CS-Glu/NiII). Also, after the well characterization, the catalytic applications of the as-prepared Fe3O4/f-MWCNT-CS-Glu/NiII hybrid system investigated on the environmentally benign one-pot synthesis of various heterocyclic frameworks, including bis-coumarins (3a–n), 2-aryl(or heteroaryl)-2,3-dihydroquinazolin-4(1H)-ones (5a–r), 9-aryl-3,3,6,6-tetramethyl-3,4,5,6,7,9-hexahydro-1H-xanthene-1,8(2H)-diones (7a–n), and 2-amino-4-aryl-7,7-dimethyl-5-oxo-5,6,7,8-tetrahydro-4H-chromene-3-carbonitriles (9a–n) (Fig. 1). Notably, the literature scrutiny clearly shows the preparation of such magnetically gigantic nanohybrid systems is not only precious in catalysis science and organic synthesis but also could be attractive and beneficial in biomedicine, drug delivery, agriculture, wastewater treatment, various type of sensors, capturing carbon dioxide, and many others.
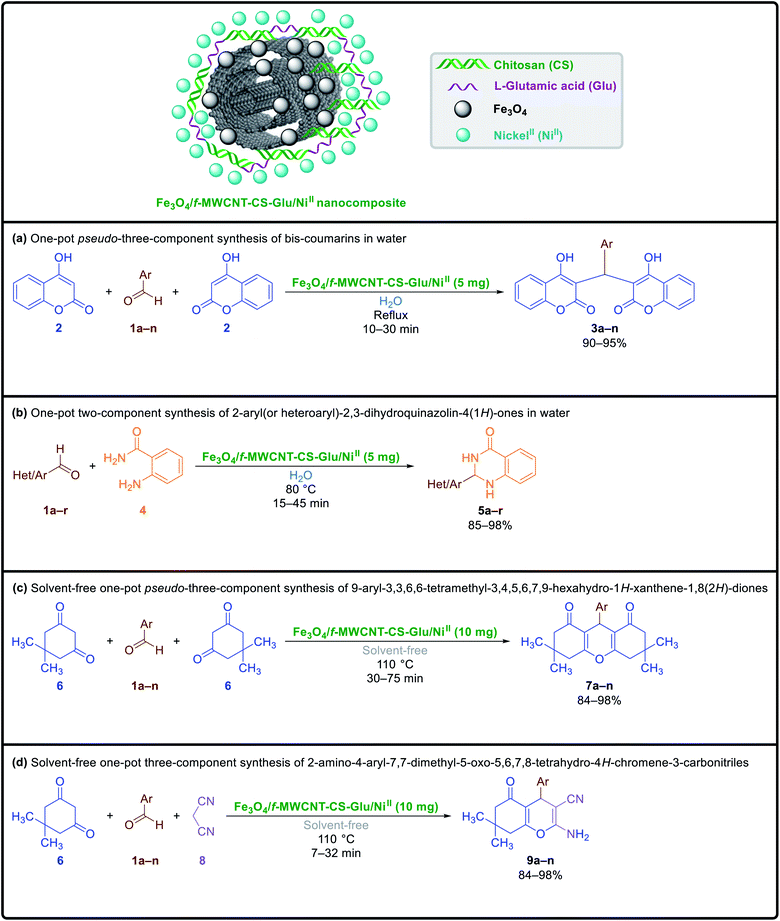 |
| Fig. 1 Catalytic applications of the as-prepared Fe3O4/f-MWCNT-CS-Glu/NiII nanocomposite in the green one-pot multi-component synthesis of diverse heterocyclic frameworks. | |
2. Results and discussion
2.1. Preparation and characterization of the Fe3O4/f-MWCNT-CS-Glu/NiII nanocomposite
In recent years, one-pot multi-component reactions are recognized as a reliable and green synthetic strategy in the preparation of gigantic frameworks and have a great place in material science. Based on this issue, we designed a new one-pot five-component sequential protocol for the L-glutamic acid cross-linked chitosan supported on magnetic carboxylic acid-functionalized multi-walled carbon nanotube (Fe3O4/f-MWCNT-CS-Glu) construction as an active, suitable, and gigantic platform for the trapping metal nanoparticles (Scheme 1). In the second step, we immobilized the nickelII nanoparticles into a matrix of the prepared Fe3O4/f-MWCNT-CS-Glu nanocomposite. After the successful preparation of the Fe3O4/f-MWCNT-CS-Glu/NiII hybrid system, we characterized the structure of the as-prepared nickelII-containing nanocomposite by Fourier transform infrared spectroscopy (FT-IR), powder X-ray diffraction (PXRD), scanning electron microscopy (SEM), transmission electron microscopy (TEM), SEM-based energy-dispersive X-ray (EDX) and elemental mapping, inductively coupled plasma-optical emission spectrometry (ICP-OES), thermogravimetric analysis/differential thermal analysis (TGA/DTA), and vibrating sample magnetometer (VSM) analysis.
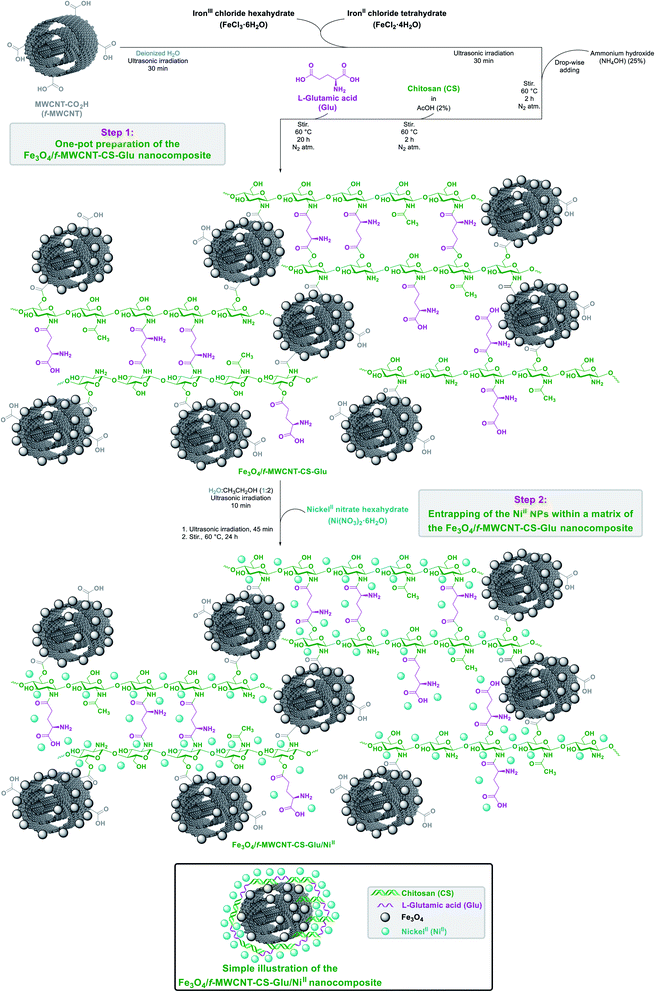 |
| Scheme 1 Preparation of the Fe3O4/f-MWCNT-CS-Glu/NiII nanocomposite. | |
The FT-IR spectroscopy of the Fe3O4, Fe3O4/f-MWCNT, Fe3O4/f-MWCNT-CS, Fe3O4/f-MWCNT-CS-Glu, and Fe3O4/f-MWCNT-CS-Glu/NiII systems was carried out using the potassium bromide (KBr) disk method, and all of the spectra were recorded in the region 400–4000 cm−1. In the FT-IR spectrum of the bare Fe3O4 nanoparticles (NPs), the absorption band at 567 cm−1 is attributed to the vibrations of the Fe–O bonds, and also the characteristic bands that appeared at 1623 cm−1 and 3418 cm−1 are approved to the bending and stretching vibrations of the surface hydroxyl (–OH) groups and adsorbed water molecules, respectively (Fig. 2, curve a). Although in the FT-IR spectrum of the Fe3O4/f-MWCNT system (Fig. 2, curve b), some index peaks are related to MWCNT-CO2H overlapped with Fe3O4 NPs (especially stretching vibrations of the hydroxyl group of –CO2H), the two distinct (weak) peaks at 2922 cm−1 and 2859 cm−1 that belong to the –CH stretching mode of the f-MWCNT nanosystem are revealed. After adding chitosan (CS) to the Fe3O4/f-MWCNT structure, a series of amide bonds were created. In the FT-IR spectrum of the Fe3O4/f-MWCNT-CS scaffold (Fig. 2, curve c), the broad band peak at 3434 cm−1 is related to the stretching vibrations of the existed –NH2, –NH–, and –OH functional groups. The peaks around 3000–2867 cm−1 belong to the C–H stretching vibrations of the –CH
(which belongs to the f-MWCNT structure) and –CH2– (which belongs to the chitosan biopolymer structure) groups. Moreover, the C
O stretching vibrations and the –NH– bending vibrations of the created amide bonds were revealed at 1634 cm−1 and 1568 cm−1, respectively. Also, the peak at 1455 cm−1 could belong to the stretching vibrations of the C–N or the C–O stretching of the secondary alcoholic groups and even the –CH2– bending vibrations in chitosan. Moreover, the C–O stretching vibrations of the primary alcoholic groups in chitosan appeared at 1411 cm−1. The bands at 1069 cm−1 and 1028 cm−1 display the C–O stretching vibrations of the etheric (C–O–C) and esteric bonds. Furthermore, the peaks at 800 cm−1, 692 cm−1, and 637 cm−1 related to the methylene and methine out-of-plane vibrations. Fig. 2 (curve d) showed the FT-IR spectrum of the Fe3O4/f-MWCNT-CS-Glu hybrid system, in which all the L-glutamic acid (Glu) functional groups peaks overlapped with some of the Fe3O4/f-MWCNT-CS peaks due to the similarity. Finally, in the FT-IR spectrum of the Fe3O4/f-MWCNT-CS-Glu/NiII magnetic nanocomposite (Fig. 2, curve e), all the distinct peaks (which thoroughly discussed above) were existed with a few differences due to the complexation of the –NH2, –NH–, –OH, and C–O–C functional groups with the nickelII nanoparticles.
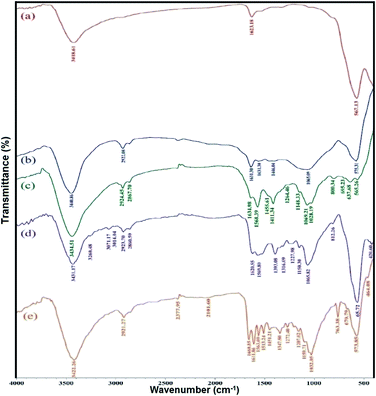 |
| Fig. 2 FT-IR spectra of the (a) Fe3O4, (b) Fe3O4/f-MWCNT, (c) Fe3O4/f-MWCNT-CS, (d) Fe3O4/f-MWCNT-CS-Glu, and (e) Fe3O4/f-MWCNT-CS-Glu/NiII nanosystems. | |
The powder X-ray diffraction (PXRD) spectra of the Fe3O4, Fe3O4/f-MWCNT, and Fe3O4/f-MWCNT-CS-Glu/NiII nanostructures are recorded in a range of Bragg's angle (2θ = 10–80°) at room temperature (Fig. 3). In the PXRD pattern of the bare Fe3O4 NPs (Fig. 3, diagram a), nine diffraction peaks at (111), (220), (311), (400), (422), (511), (440), (620), and (553), which are indexed to the cubic spinel phase of Fe3O4, clearly appeared and matched well with Joint Committee on Powder Diffraction Standards (JCPDS) cards, file no. 79-0418, 65-3107, 74-2402, 01-075-0449, and 98-007-7842. In the Fe3O4/f-MWCNT PXRD pattern (Fig. 3, diagram b), in addition to the distinct peaks of the Fe3O4 NPs, a peak around 2θ = 26° is related to the f-MWCNT structure. As shown in Fig. 3 (diagram c), the intensity of the 2θ = 26° in the PXRD pattern of the Fe3O4/f-MWCNT-CS-Glu/NiII nanocomposite is increased, which could be related to the overlapping L-glutamic acid cross-linked chitosan (CS-Glu) peak with the f-MWCNT peak. Notably, the distinct peaks of the nickelII NPs in the as-prepared nanocomposite structure were overlapped with some of the Fe3O4 NPs peaks.
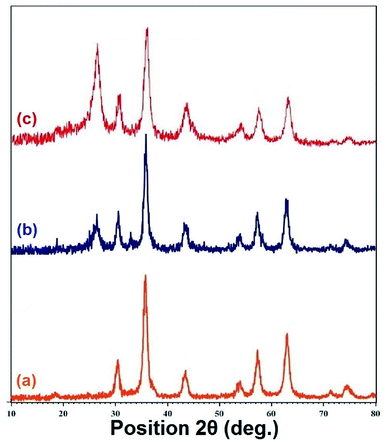 |
| Fig. 3 PXRD patterns of the (a) Fe3O4, (b) Fe3O4/f-MWCNT, and (c) Fe3O4/f-MWCNT-CS-Glu/NiII nanosystems. | |
The morphology and size of the Fe3O4/f-MWCNT-CS-Glu/NiII hybrid nanocomposite were investigated by scanning electron microscopy (SEM) and transmission electron microscopy (TEM). The SEM images of the mentioned magnetic nanosystem (Fig. 4) indicated a spaghetti-like structure with a diameter of less than 44 nm related to the f-MWCNT and L-glutamic acid cross-linked chitosan (CS-Glu), which are surrounded by the Fe3O4 and nickelII NPs. Also, in the Fe3O4/f-MWCNT-CS-Glu/NiII TEM images (Fig. 5), the existence of the Fe3O4 NPs and the entrapped NiII NPs into the matrix of the Fe3O4/f-MWCNT-CS-Glu nanocomposite can be seen clearly.
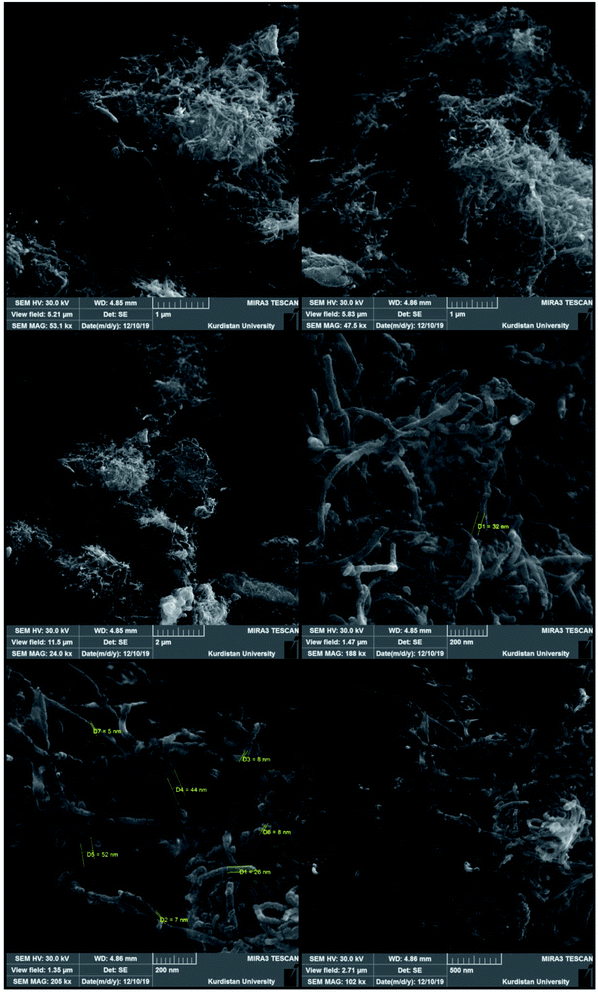 |
| Fig. 4 SEM images of the as-prepared Fe3O4/f-MWCNT-CS-Glu/NiII nanocomposite. | |
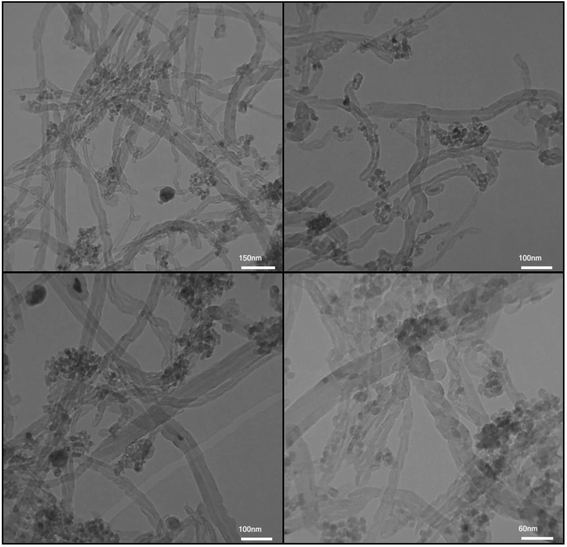 |
| Fig. 5 TEM images of the as-prepared Fe3O4/f-MWCNT-CS-Glu/NiII nanocomposite. | |
In order to further validate the composition elements of the as-prepared Fe3O4/f-MWCNT-CS-Glu/NiII nanocomposite, the SEM-based energy-dispersive X-ray (EDX) (Fig. 6) and elemental mapping (Fig. 7) analyses were performed. Both EDX and elemental mapping investigations confirm the presence of the carbon (C), nitrogen (N), oxygen (O), ferrite (Fe), and nickel (Ni) elements in the structure of the as-prepared gigantic hybrid nanosystem. The presence of the nickel element in the EDX and elemental mapping analyses inferred that the nickelII NPs were successfully entrapped into the matrix of the Fe3O4/f-MWCNT-CS-Glu nanocomposite. Also, the EDX analysis gives the percentage of the elements, including 29.10 w%, 0.32 w%, 2.28 w%, 46.27 w%, and 22.03 w% for C, N, O, Fe, and Ni, respectively. Notably, the exact amount of the entrapped nickel is 12.32 w% based on the inductively coupled plasma-optical emission spectrometry (ICP-OES) measurement.
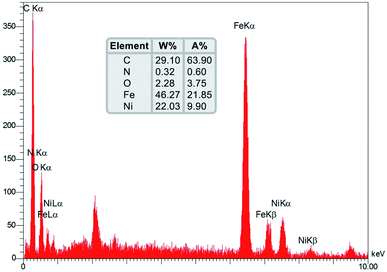 |
| Fig. 6 SEM-based EDX diagram of the as-prepared Fe3O4/f-MWCNT-CS-Glu/NiII nanocomposite. | |
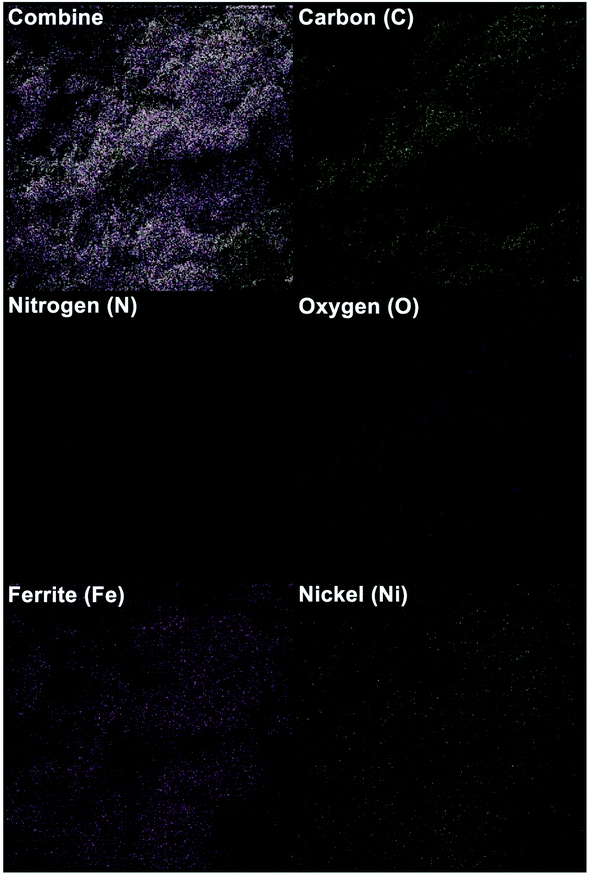 |
| Fig. 7 SEM-based elemental mapping of the as-prepared Fe3O4/f-MWCNT-CS-Glu/NiII nanocomposite. | |
Thermogravimetric analysis/differential thermal analysis (TGA/DTA) was carried out to assess the thermal stability of the as-prepared Fe3O4/f-MWCNT-CS-Glu/NiII nanocomposite by heating it up to 800 °C under a nitrogen gas atmosphere (Fig. 8). In the first step, a weight loss (1%) occurred below 240 °C is related to the solvents and moisture evaporations. In the second step, a weight loss (9%) is observed at temperature ranging from 240 °C to 352 °C, which is mainly associated to the decomposition of L-glutamic acid27 (as the cross-linker) and also is corresponding to the dehydration and depolymerization of the chitosan biopolymer.28 In the third stage, a weight loss (6%) is observed between temperature range of 352 °C to 542 °C attributed to the complete decomposition of the chitosan structure by degasification of compounds, including NH3, CO, CO2, and CH4.29 In the final step, a weight loss (12%) is observed between temperature range of 542 °C to approximately 800 °C could be related to the pyrolysis and destruction of the f-MWCNT network.30 It is worthwhile to note that the results of the TGA/DTA diagram confirm that the as-prepared Fe3O4/f-MWCNT-CS-Glu/NiII nanocomposite has enough and satisfactory stability to endure harsh temperature conditions up to 240 °C, which is so suitable for a catalytic system in organic synthesis.
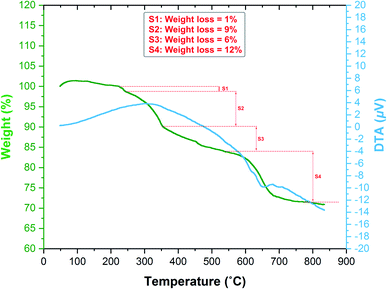 |
| Fig. 8 TGA/DTA diagram of the as-prepared Fe3O4/f-MWCNT-CS-Glu/NiII nanocomposite. | |
The magnetic behavior of the prepared Fe3O4, Fe3O4/f-MWCNT, and Fe3O4/f-MWCNT-CS-Glu/NiII nanostructures was investigated by a vibrating sample magnetometer (VSM) analysis at room temperature (Fig. 9). As it could be perceived from the resulting magnetization curves, the saturation magnetization (Ms) amounts of the Fe3O4, Fe3O4/f-MWCNT, and Fe3O4/f-MWCNT-CS-Glu/NiII nanosystems were 66.496 emu g−1, 46.519 emu g−1, and 19.743 emu g−1, respectively. The decrease in the Ms amount of the as-prepared Fe3O4/f-MWCNT-CS-Glu/NiII nanocomposite rather than Fe3O4/f-MWCNT and bare Fe3O4 is attributed to the successful attaching L-glutamic acid cross-linked chitosan (CS-Glu) on the surface of the Fe3O4/f-MWCNT magnetic system and immobilization of the nickelII NPs into the matrix of the Fe3O4/f-MWCNT-CS-Glu nanocomposite.
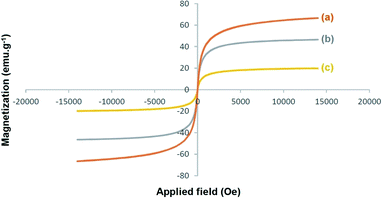 |
| Fig. 9 Magnetization curves of the prepared (a) Fe3O4, (b) Fe3O4/f-MWCNT, and (c) Fe3O4/f-MWCNT-CS-Glu/NiII nanosystems. | |
2.2. Catalytic applications of the as-prepared Fe3O4/f-MWCNT-CS-Glu/NiII nanocomposite
2.2.1. One-pot pseudo-three-component synthesis of bis-coumarins. After preparation and characterization of the Fe3O4/f-MWCNT-CS-Glu/NiII nanocomposite, to the demonstration of application merits of this new hybrid nanostructured catalyst in organic synthesis, we applied it in the one-pot synthesis of some valuable heterocyclic frameworks. In the first step, the one-pot synthesis of bis-coumarins (3a–n) was investigated. In order to optimize the reaction conditions, the simple one-pot pseudo-three-component synthesis of 3,3′-(phenylmethylene)bis(4-hydroxy-2H-chromen-2-one) (3a) through a condensation reaction between benzaldehyde (1a) and 4-hydroxycoumarin (2) was chosen as a model reaction. In the first step, the mentioned one-pot reaction was carried out in the presence of the as-prepared catalyst (5 mg) in several solvents, including water (H2O), methanol (CH3OH), ethanol (CH3CH2OH), acetonitrile (CH3CN), ethyl acetate (EtOAc), dichloromethane (CH2Cl2), and n-hexane, and also under solvent-free conditions (Table 1). Although the obtained yield in water (Table 1, entry 1), ethanol (Table 1, entry 5), and solventless (Table 1, entry 10) mediums were high and the same (viz. 95%), the reaction rate in water and ethanol under reflux was faster than in solvent-free conditions at 70 °C. Based on obtained results and have respect to the green chemistry protocols, we selected water as the best and environmentally benign solvent for this one-pot reaction. Then, we examined the effect of less than (Table 1, entry 2) and more than (Table 1, entry 3) 5 mg of the as-prepared Fe3O4/f-MWCNT-CS-Glu/NiII catalyst on the synthesis of 3a in water under reflux conditions and it was found that the optimal amount of the catalyst loading is 5 mg. Furthermore and to show the importance and effect of the as-prepared NiII-containing nanocatalyst on the synthesis of 3a, we designed and carried out some control experiments using component parts of the Fe3O4/f-MWCNT-CS-Glu/NiII nanocomposite (Table 1, entries 11–14). To our delight, the control experiments results showed that the catalytic effect of the mentioned nanocomposite is definitely better than its components. After optimizing the reaction conditions, we next examined the substrate scope for the mentioned one-pot pseudo-three-component reaction using diverse aromatic aldehyde derivatives (Scheme 2). Interestingly, both the electron-donating and electron-withdrawing groups (EDGs and EWGs) on aryl aldehydes were found tolerable to reaction conditions and provided products (3a–n) in good-to-excellent yields. Also, the turnover numbers (TONs) and turnover frequencies (TOFs) of the Fe3O4/f-MWCNT-CS-Glu/NiII nanocatalyst in this one-pot pseudo-three-component synthesis of bis-coumarins (3a–n) were calculated. As shown in Scheme 2, the determined TONs and TOFs are acceptable and satisfactory. The chemical structures of the synthesized bis-coumarins (3a–n) were characterized by FT-IR, 1H NMR, and melting points, and compared with authentic literature. Furthermore, a plausible mechanism for this valuable tandem Knoevenagel–Michael condensation in the presence of the as-prepared Fe3O4/f-MWCNT-CS-Glu/NiII hybrid nanocatalytic system is depicted in Scheme 3. Briefly, in the first stage, aromatic aldehyde (1a–n) activated by the as-prepared Fe3O4/f-MWCNT-CS-Glu/NiII nanocomposite (especially by the NiII section) and then the nucleophilic attack of 4-hydroxycoumarin (2) to the mentioned activated aryl aldehyde caused the elimination of one water molecule and formation of the 3-benzylidenechromane-2,4-dione intermediate (IA). Next, the Michael addition of another 4-hydroxycoumarin (2) to the activated α,β-unsaturated carbonyl compound (IA) yielded corresponding bis-coumarins (3a–n).
Table 1 Optimization reaction conditions for the one-pot pseudo-three-component synthesis of 3a
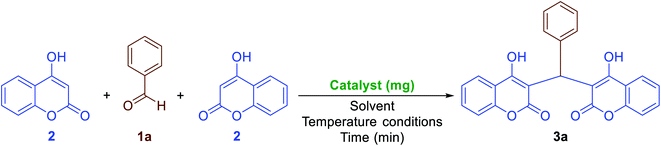
|
Entry |
Catalyst |
Catalyst loading (mg) |
Solvent |
Temperature conditions |
Time (min) |
Yield (%) |
1 |
Fe3O4/f-MWCNT-CS-Glu/NiII |
5 |
H2O |
Reflux |
10 |
95 |
2 |
Fe3O4/f-MWCNT-CS-Glu/NiII |
4 |
H2O |
Reflux |
15 |
90 |
3 |
Fe3O4/f-MWCNT-CS-Glu/NiII |
7 |
H2O |
Reflux |
10 |
95 |
4 |
Fe3O4/f-MWCNT-CS-Glu/NiII |
5 |
CH3OH |
Reflux |
20 |
65 |
5 |
Fe3O4/f-MWCNT-CS-Glu/NiII |
5 |
CH3CH2OH |
Reflux |
10 |
95 |
6 |
Fe3O4/f-MWCNT-CS-Glu/NiII |
5 |
CH3CN |
Reflux |
120 |
35 |
7 |
Fe3O4/f-MWCNT-CS-Glu/NiII |
5 |
EtOAC |
Reflux |
120 |
25 |
8 |
Fe3O4/f-MWCNT-CS-Glu/NiII |
5 |
CH2Cl2 |
35 °C |
120 |
20 |
9 |
Fe3O4/f-MWCNT-CS-Glu/NiII |
5 |
n-Hexane |
Reflux |
120 |
10 |
10 |
Fe3O4/f-MWCNT-CS-Glu/NiII |
5 |
Solvent-free |
70 °C |
15 |
95 |
11 |
Fe3O4/f-MWCNT-CS-Glu |
5 |
H2O |
Reflux |
120 |
55 |
12 |
Fe3O4/f-MWCNT-CS |
5 |
H2O |
Reflux |
120 |
55 |
13 |
Fe3O4/f-MWCNT |
5 |
H2O |
Reflux |
120 |
50 |
14 |
Fe3O4 |
5 |
H2O |
Reflux |
120 |
— |
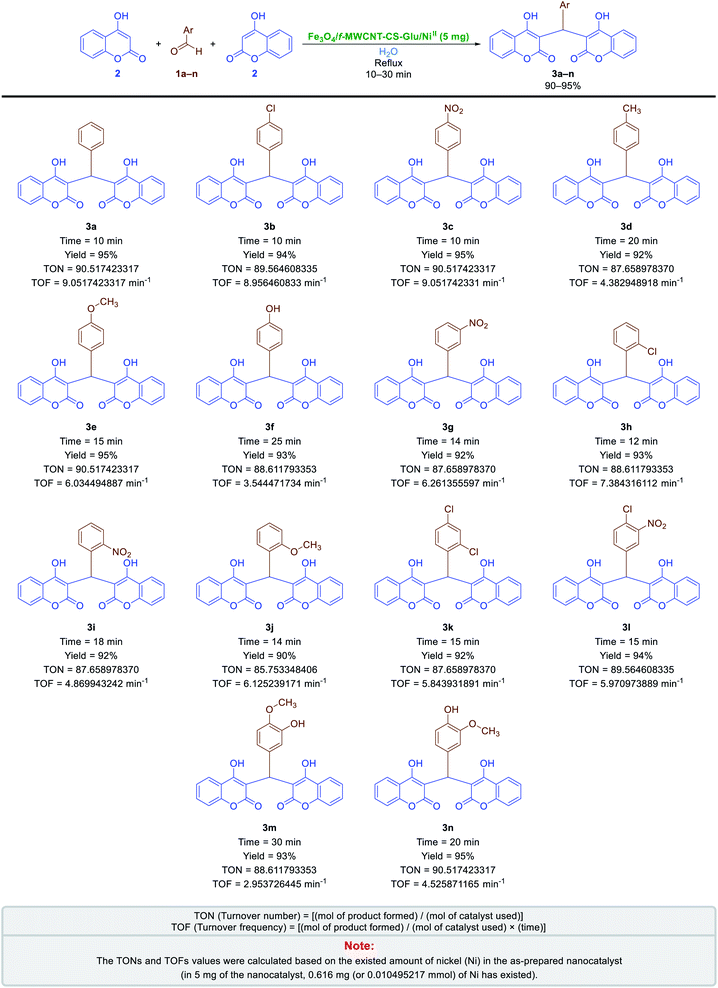 |
| Scheme 2 One-pot pseudo-three-component synthesis of bis-coumarins in water catalyzed by the as-prepared Fe3O4/f-MWCNT-CS-Glu/NiII nanocomposite. | |
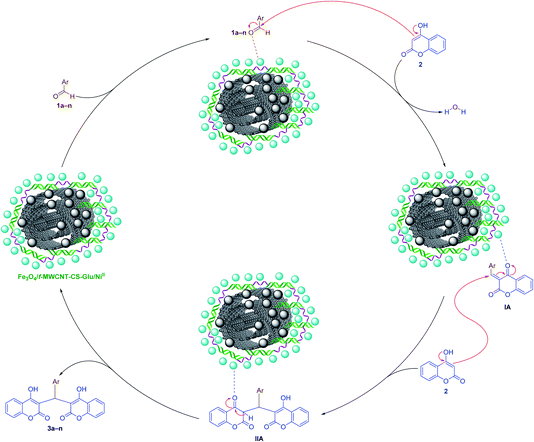 |
| Scheme 3 Plausible mechanism for the one-pot pseudo-three-component synthesis of bis-coumarins in the presence of the as-prepared Fe3O4/f-MWCNT-CS-Glu/NiII nanocatalyst. | |
2.2.2. One-pot two-component synthesis of 2-aryl(or heteroaryl)-2,3-dihydroquinazolin-4(1H)-ones. Another catalytic application of the as-prepared Fe3O4/f-MWCNT-CS-Glu/NiII nanocomposite investigated in the green and one-pot two-component synthesis of 2-aryl(or heteroaryl)-2,3-dihydroquinazolin-4(1H)-ones (5a–r). It is worthy to note that quinazolinones are fundamental building blocks in nature and part of the backbone of several naturally occurring alkaloids and biologically active compounds.31 After optimization of the main reaction parameters (Table 2), which take place on the one-pot synthesis of 2-phenyl-2,3-dihydroquinazolin-4(1H)-one (5a) through a two-component reaction of benzaldehyde (1a) and 2-aminobenzamide (also known as anthranilamide) (4), the scope and limitation of the presented strategy studied using a wide range of aromatic and heteroaromatic aldehydes under the optimized reaction conditions (Table 2, entry 1). As shown in Scheme 4, all of the desired 2-aryl(or heteroaryl)-2,3-dihydroquinazolin-4(1H)-ones (5a–r) obtained in good-to-excellent yields in water at 80 °C in the presence of 5 mg of the mentioned NiII-containing nanocomposite. On the other hand, the TONs and TOFs of the Fe3O4/f-MWCNT-CS-Glu/NiII nanocatalyst in this one-pot two-component reaction have been measured and listed in Scheme 4. The chemical structures of the obtained 2-aryl(or heteroaryl)-2,3-dihydroquinazolin-4(1H)-ones (5a–r) were confirmed by FT-IR, 1H NMR, and melting points, and compared with authentic papers. Also, a plausible mechanism for this green one-pot two-component reaction in the presence of the mentioned gigantic catalytic system is presented in Scheme 5. As shown in Scheme 5, in the first step, aromatic(or heteroaromatic) aldehyde (1a–r) was activated by the as-prepared catalytic system, and then the mentioned carbonyl group of aryl(or heteroaryl)aldehyde was attacked by the amine section of 2-aminobenzamide (4) that leads to the formation of the aldimine intermediate (IB) along with the elimination of one water molecule. In the next step, the activated IB intermediate converted to the IIB intermediate by the amide-iminol tautomerization process. After that, the IIB intermediate, which was activated by the Fe3O4/f-MWCNT-CS-Glu/NiII nanocomposite, generated desired product (5a–r) by an intramolecular heterocyclization pathway which occurs through a [1,5]-hydrogen transfer.
Table 2 Optimization reaction conditions for the one-pot two-component synthesis of 5a
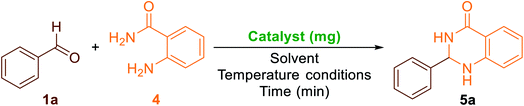
|
Entry |
Catalyst |
Catalyst loading (mg) |
Solvent |
Temperature conditions |
Time (min) |
Yield (%) |
1 |
Fe3O4/f-MWCNT-CS-Glu/NiII |
5 |
H2O |
80 °C |
20 |
98 |
2 |
Fe3O4/f-MWCNT-CS-Glu/NiII |
4 |
H2O |
80 °C |
20 |
92 |
3 |
Fe3O4/f-MWCNT-CS-Glu/NiII |
7 |
H2O |
80 °C |
20 |
98 |
4 |
Fe3O4/f-MWCNT-CS-Glu/NiII |
5 |
CH3OH |
Reflux |
30 |
84 |
5 |
Fe3O4/f-MWCNT-CS-Glu/NiII |
5 |
CH3CH2OH |
Reflux |
20 |
98 |
6 |
Fe3O4/f-MWCNT-CS-Glu/NiII |
5 |
CH3CN |
Reflux |
30 |
90 |
7 |
Fe3O4/f-MWCNT-CS-Glu/NiII |
5 |
EtOAc |
Reflux |
90 |
50 |
8 |
Fe3O4/f-MWCNT-CS-Glu/NiII |
5 |
CH2Cl2 |
35 °C |
120 |
25 |
9 |
Fe3O4/f-MWCNT-CS-Glu/NiII |
5 |
n-Hexane |
Reflux |
120 |
15 |
10 |
Fe3O4/f-MWCNT-CS-Glu/NiII |
5 |
THF![[thin space (1/6-em)]](https://www.rsc.org/images/entities/char_2009.gif) |
Reflux |
120 |
70 |
11 |
Fe3O4/f-MWCNT-CS-Glu/NiII |
5 |
Solvent-free |
70 °C |
15 |
95 |
12 |
Fe3O4/f-MWCNT-CS-Glu |
5 |
H2O |
80 °C |
120 |
30 |
13 |
Fe3O4/f-MWCNT-CS |
5 |
H2O |
80 °C |
120 |
10 |
14 |
Fe3O4/f-MWCNT |
5 |
H2O |
80 °C |
120 |
10 |
15 |
Fe3O4 |
5 |
H2O |
80 °C |
120 |
— |
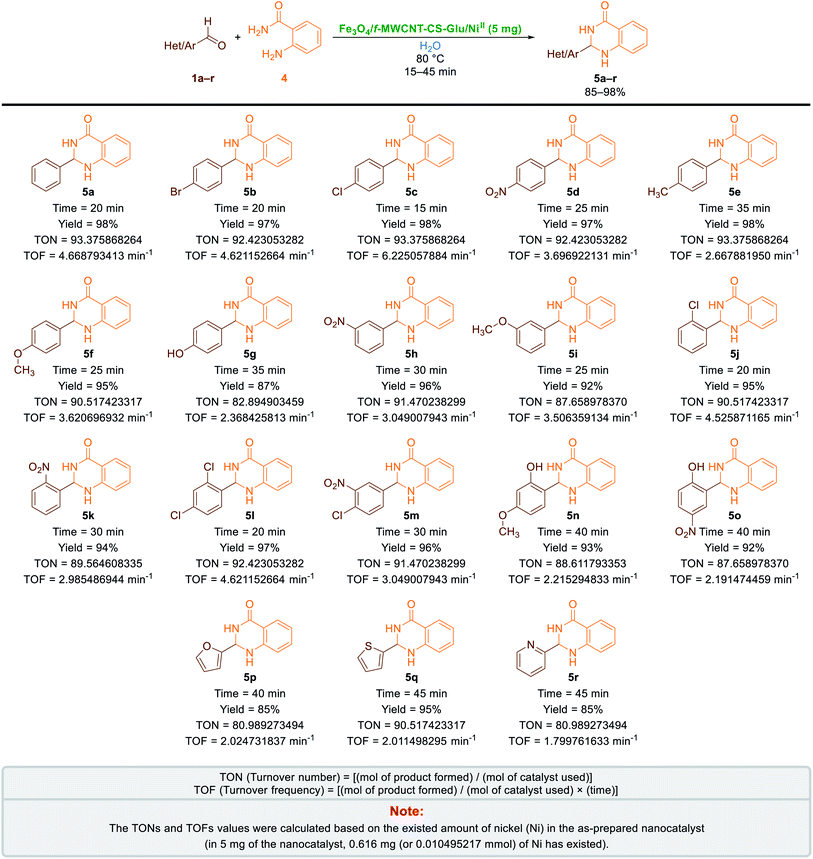 |
| Scheme 4 One-pot two-component synthesis of 2-aryl(or heteroaryl)-2,3-dihydroquinazolin-4(1H)-ones catalyzed by the as-prepared Fe3O4/f-MWCNT-CS-Glu/NiII nanocomposite in water. | |
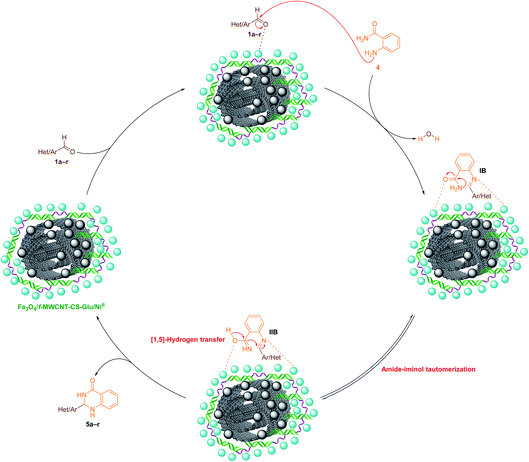 |
| Scheme 5 Plausible mechanism for the one-pot two-component synthesis of 2-aryl(or heteroaryl)-2,3-dihydroquinazolin-4(1H)-ones catalyzed by the as-prepared Fe3O4/f-MWCNT-CS-Glu/NiII nanocomposite in water. | |
2.2.3. One-pot multi-component synthesis of fused 4H-pyrans. The results related to the impressive catalytic effect of the as-prepared Fe3O4/f-MWCNT-CS-Glu/NiII hybrid nanocomposite in the green one-pot synthesis of bis-coumarins (3a–n) and 2-aryl(or heteroaryl)-2,3-dihydroquinazolin-4(1H)-ones (5a–r) encouraged us to study and develop catalytic applications of this magnetic hybrid nanocatalyst in the environmentally benign one-pot multi-component synthesis of other heterocyclic frameworks as well. To this purpose, we selected 9-aryl-3,3,6,6-tetramethyl-3,4,5,6,7,9-hexahydro-1H-xanthene-1,8(2H)-diones (7a–n) and 2-amino-4-aryl-7,7-dimethyl-5-oxo-5,6,7,8-tetrahydro-4H-chromene-3-carbonitriles (9a–n) as two important heterocyclic systems of the fused 4H-pyrans family. The optimization studies upon model reactions (Tables 3 and 4) showed that both of the two mentioned heterocyclic compounds could be successfully synthesized under solvent-free conditions in the presence of 10 mg of the mentioned nanocatalyst at 110 °C. Further studies on the scope and generality of the presented one-pot multi-component reactions indicated that the desired 9-aryl-3,3,6,6-tetramethyl-3,4,5,6,7,9-hexahydro-1H-xanthene-1,8(2H)-diones (7a–n) (Scheme 6) and 2-amino-4-aryl-7,7-dimethyl-5-oxo-5,6,7,8-tetrahydro-4H-chromene-3-carbonitriles (9a–n) (Scheme 7) could be obtained in relatively good-to-excellent yields under the optimal reaction conditions. Furthermore, the TONs and TOFs of the as-prepared Fe3O4/f-MWCNT-CS-Glu/NiII hybrid nanocatalyst in these one-pot reactions have been measured and listed in Schemes 6 and 7. The chemical structures of the synthesized fused 4H-pyrans (7a–n) and (9a–n) were confirmed by FT-IR, 1H NMR, and melting points, and compared with authentic papers. Also, the plausible mechanisms for these solvent-free one-pot multi-component reactions in the presence of the Fe3O4/f-MWCNT-CS-Glu/NiII gigantic catalytic system are presented in Schemes 8 and 9. As shown in Scheme 8, the formation of 9-aryl-3,3,6,6-tetramethyl-3,4,5,6,7,9-hexahydro-1H-xanthene-1,8(2H)-diones (7a–n) via the presented one-pot pseudo-three-component synthetic strategy started with the activation of the carbonyl group of aryl aldehyde (1a–n) by the Fe3O4/f-MWCNT-CS-Glu/NiII nanocatalyst and subsequently nucleophilic attack of dimedone (6) that leads to the formation of the Knoevenagel intermediate (IC) along with the elimination of one water molecule. In the next step, the Michael addition of the second dimedone molecule (6) upon activated IC caused the formation of IIC. In the final step, intramolecular cyclization of the activated IIC occurs after the successful elimination of another water molecule resulted desired xanthene (7a–n) and regenerated Fe3O4/f-MWCNT-CS-Glu/NiII magnetic nanocatalyst into the reaction pot. Also, as shown in Scheme 9, the reaction mechanism of the formation of 2-amino-4-aryl-7,7-dimethyl-5-oxo-5,6,7,8-tetrahydro-4H-chromene-3-carbonitriles (9a–n) in the presence of the as-prepared NiII-containing nanocatalyst started by the formation of the arylidienemalononitrile intermediate (ID) and releasing one water molecule via the Knoevenagel condensation between malononitrile (8) and the activated aromatic aldehyde (1a–n). Next, the Michael addition of the dimedone molecule (6) to the activated arylidienemalononitrile (ID) occurred to form IID intermediate. Finally, the intramolecular cyclization of the activated IID intermediate resulted desired fused heterocyclic product (9a–n).
Table 3 Optimization reaction conditions for the one-pot pseudo-three-component synthesis of 7a
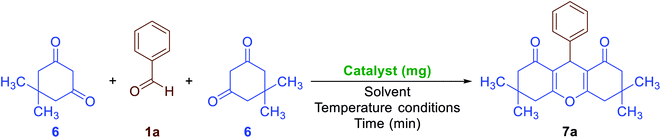
|
Entry |
Catalyst |
Catalyst loading (mg) |
Solvent |
Temperature conditions |
Time (min) |
Yield (%) |
1 |
Fe3O4/f-MWCNT-CS-Glu/NiII |
10 |
H2O |
Reflux |
120 |
50 |
2 |
Fe3O4/f-MWCNT-CS-Glu/NiII |
10 |
CH3OH |
Reflux |
40 |
70 |
3 |
Fe3O4/f-MWCNT-CS-Glu/NiII |
10 |
CH3CH2OH |
Reflux |
40 |
78 |
4 |
Fe3O4/f-MWCNT-CS-Glu/NiII |
10 |
CH3CN |
Reflux |
180 |
65 |
5 |
Fe3O4/f-MWCNT-CS-Glu/NiII |
10 |
EtOAc |
Reflux |
180 |
25 |
6 |
Fe3O4/f-MWCNT-CS-Glu/NiII |
10 |
CH2Cl2 |
35 °C |
120 |
10 |
7 |
Fe3O4/f-MWCNT-CS-Glu/NiII |
10 |
n-Hexane |
Reflux |
180 |
20 |
8 |
Fe3O4/f-MWCNT-CS-Glu/NiII |
10 |
THF![[thin space (1/6-em)]](https://www.rsc.org/images/entities/char_2009.gif) |
Reflux |
120 |
30 |
9 |
Fe3O4/f-MWCNT-CS-Glu/NiII |
10 |
Solvent-free |
110 °C |
40 |
96 |
10 |
Fe3O4/f-MWCNT-CS-Glu/NiII |
5 |
Solvent-free |
110 °C |
40 |
82 |
11 |
Fe3O4/f-MWCNT-CS-Glu/NiII |
15 |
Solvent-free |
110 °C |
40 |
96 |
12 |
Fe3O4/f-MWCNT-CS-Glu |
10 |
Solvent-free |
110 °C |
180 |
30 |
13 |
Fe3O4/f-MWCNT-CS |
10 |
Solvent-free |
110 °C |
180 |
10 |
14 |
Fe3O4/f-MWCNT |
10 |
Solvent-free |
110 °C |
180 |
10 |
15 |
Fe3O4 |
10 |
Solvent-free |
110 °C |
180 |
— |
Table 4 Optimization reaction conditions for the one-pot three-component synthesis of 9a
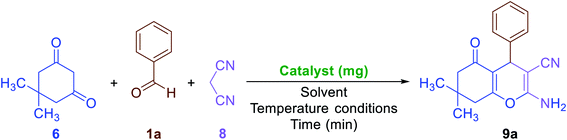
|
Entry |
Catalyst |
Catalyst loading (mg) |
Solvent |
Temperature conditions |
Time (min) |
Yield (%) |
1 |
Fe3O4/f-MWCNT-CS-Glu/NiII |
10 |
H2O |
Reflux |
120 |
65 |
2 |
Fe3O4/f-MWCNT-CS-Glu/NiII |
10 |
CH3OH |
Reflux |
30 |
60 |
3 |
Fe3O4/f-MWCNT-CS-Glu/NiII |
10 |
CH3CH2OH |
Reflux |
30 |
72 |
4 |
Fe3O4/f-MWCNT-CS-Glu/NiII |
10 |
CH3CN |
Reflux |
60 |
60 |
5 |
Fe3O4/f-MWCNT-CS-Glu/NiII |
10 |
EtOAc |
Reflux |
80 |
15 |
6 |
Fe3O4/f-MWCNT-CS-Glu/NiII |
10 |
CH2Cl2 |
35 °C |
120 |
— |
7 |
Fe3O4/f-MWCNT-CS-Glu/NiII |
10 |
n-Hexane |
Reflux |
120 |
10 |
8 |
Fe3O4/f-MWCNT-CS-Glu/NiII |
10 |
THF![[thin space (1/6-em)]](https://www.rsc.org/images/entities/char_2009.gif) |
Reflux |
40 |
45 |
9 |
Fe3O4/f-MWCNT-CS-Glu/NiII |
10 |
Solvent-free |
110 °C |
10 |
97 |
10 |
Fe3O4/f-MWCNT-CS-Glu/NiII |
5 |
Solvent-free |
110 °C |
15 |
85 |
11 |
Fe3O4/f-MWCNT-CS-Glu/NiII |
15 |
Solvent-free |
110 °C |
10 |
97 |
12 |
Fe3O4/f-MWCNT-CS-Glu |
10 |
Solvent-free |
110 °C |
120 |
40 |
13 |
Fe3O4/f-MWCNT-CS |
10 |
Solvent-free |
110 °C |
120 |
40 |
14 |
Fe3O4/f-MWCNT |
10 |
Solvent-free |
110 °C |
120 |
30 |
15 |
Fe3O4 |
10 |
Solvent-free |
110 °C |
120 |
— |
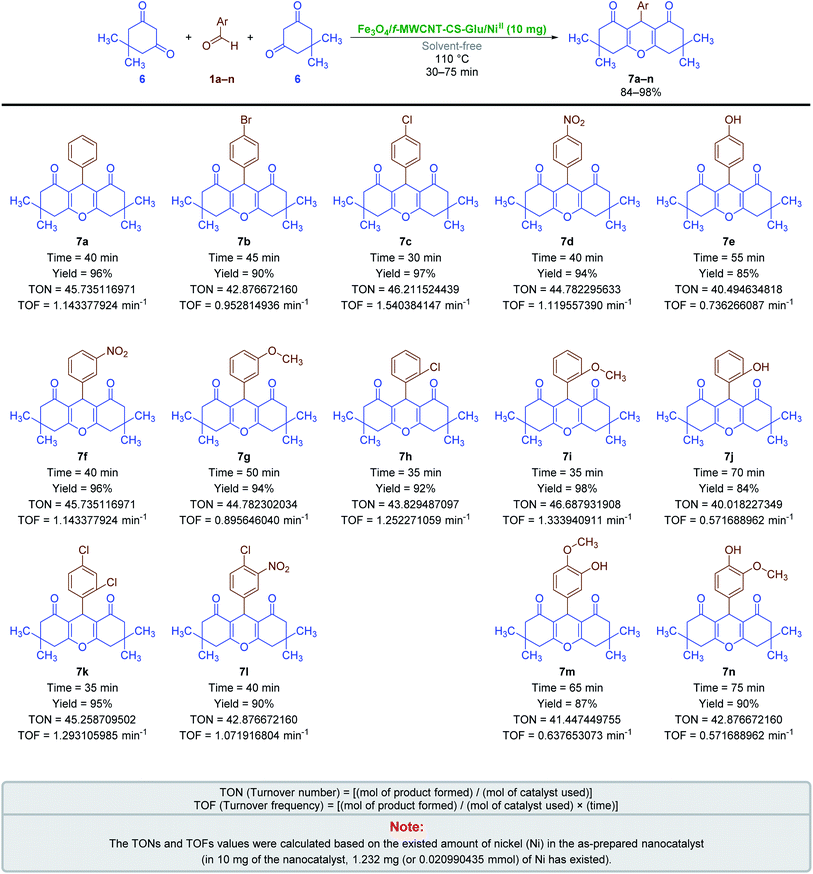 |
| Scheme 6 Solvent-free one-pot pseudo-three-component synthesis of 9-aryl-3,3,6,6-tetramethyl-3,4,5,6,7,9-hexahydro-1H-xanthene-1,8(2H)-diones catalyzed by the as-prepared Fe3O4/f-MWCNT-CS-Glu/NiII nanocomposite. | |
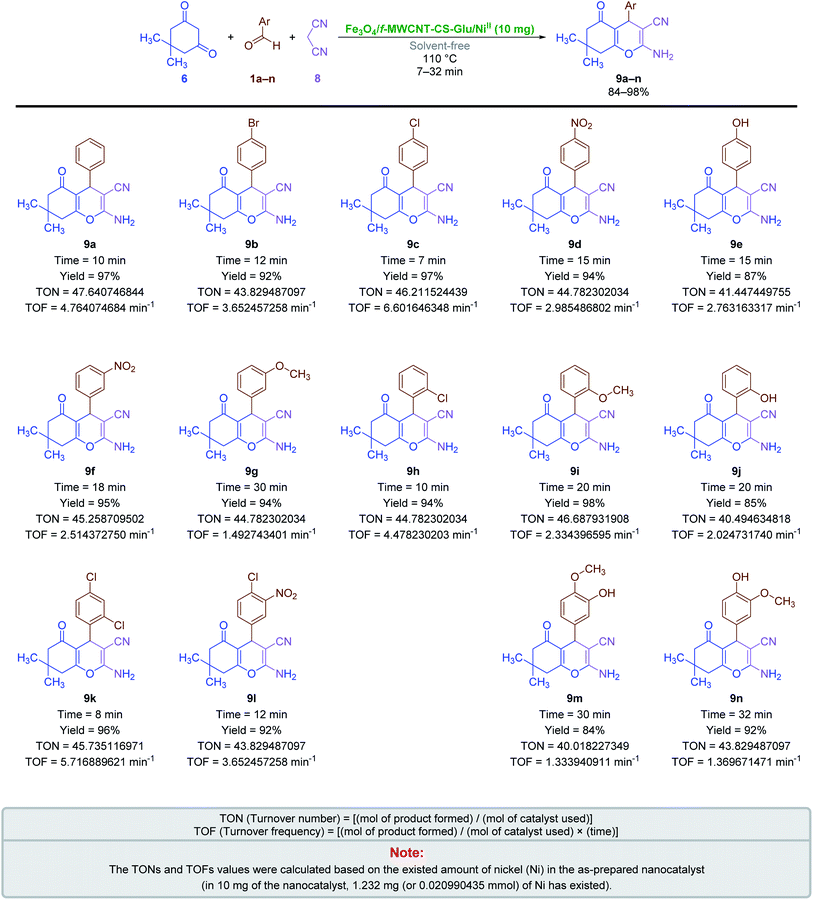 |
| Scheme 7 Solvent-free one-pot three-component synthesis of 2-amino-4-aryl-7,7-dimethyl-5-oxo-5,6,7,8-tetrahydro-4H-chromene-3-carbonitriles catalyzed by the as-prepared Fe3O4/f-MWCNT-CS-Glu/NiII nanocomposite. | |
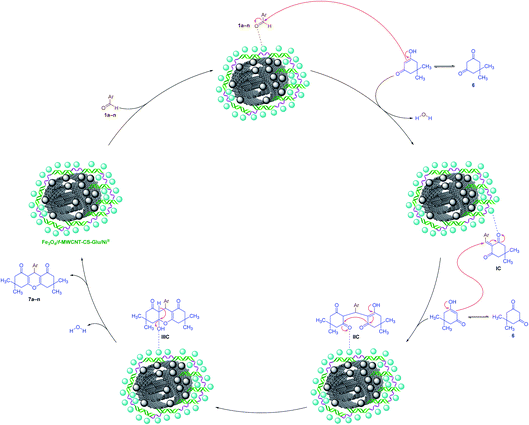 |
| Scheme 8 Plausible mechanism for the solvent-free one-pot pseudo-three-component synthesis of 9-aryl-3,3,6,6-tetramethyl-3,4,5,6,7,9-hexahydro-1H-xanthene-1,8(2H)-diones catalyzed by the as-prepared Fe3O4/f-MWCNT-CS Glu/NiII nanocomposite. | |
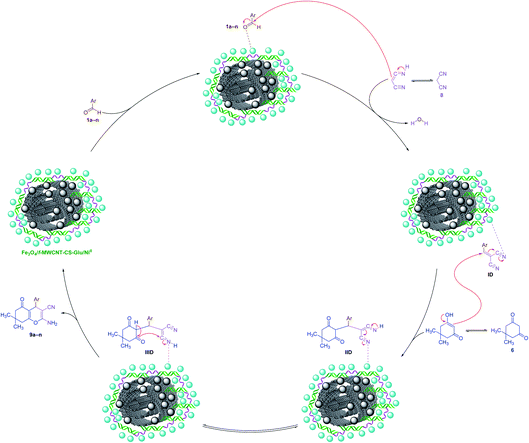 |
| Scheme 9 Plausible mechanism for the solvent-free one-pot three-component synthesis of 2-amino-4-aryl-7,7-dimethyl-5-oxo-5,6,7,8-tetrahydro-4H-chromene-3-carbonitriles catalyzed by the as-prepared Fe3O4/f-MWCNT-CS-Glu/NiII nanocomposite. | |
2.3. Recoverability, reusability, leaching, and hot filtration test studies of the as-prepared Fe3O4/f-MWCNT-CS-Glu/NiII nanocomposite
Easy recovery and high reusability of catalyst are remarkably significant factors, exclusively for commercial and industrial applications and also from the green chemistry point of view. In this line, we performed some experiments to investigate the catalyst recyclability for all the aforesaid four model organic reactions on the one-pot synthesis of 3a, 5a, 7a, and 9a under the optimized reaction conditions. To do this and after each investigation, the catalyst was separated from the reaction pot with a magnet. Next, the isolated nickelII-containing catalyst was washed, dried, and then directly used in the next run of the reaction. As shown in Fig. 10, the recoverability and reusability experiments of the Fe3O4/f-MWCNT-CS-Glu/NiII nanocomposite revealed satisfactory results even after eight runs for all the four mentioned one-pot synthetic protocols. On the other hand, in order to evaluation of the Fe3O4/f-MWCNT-CS-Glu/NiII stability into the reaction environment, we carried out leaching tests, which were investigated on the one-pot two-component synthesis of 2-phenyl-2,3-dihydroquinazolin-4(1H)-one (5a). The leaching tests results obtained by ICP-OES measurements showed that the amount of nickel (Ni) after the fourth and eighth reaction cycles was 11.08 w% and 9.74 w%, respectively. The hot filtration test was also performed for the further investigation upon leaching of the NiII NPs on the one-pot synthesis of 5a in the water solvent. As shown in Fig. 11, we separated the whole of the as-prepared Fe3O4/f-MWCNT-CS-Glu/NiII nano-based catalytic system from the mentioned reaction environment (when the conversion rate was 60%), and it was found that after filtration and in the absence of the aforesaid NiII-containing catalyst, almost no significant improvement was seen in the reaction process. The conversion rate of the reaction in this stage confirms the leaching of NiII was negligible. Furthermore, the PXRD diagram (Fig. 12) of the recycled Fe3O4/f-MWCNT-CS-Glu/NiII catalytic system after the third recycling step with those of the fresh one shows that the structure of the mentioned hybrid nanocomposite remained intact.
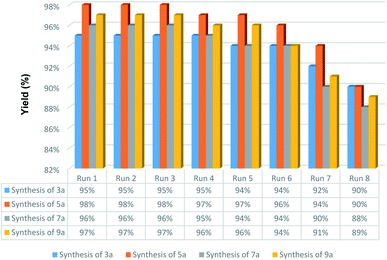 |
| Fig. 10 Recoverability and reusability experiments of the Fe3O4/f-MWCNT-CS-Glu/NiII nanocomposite. | |
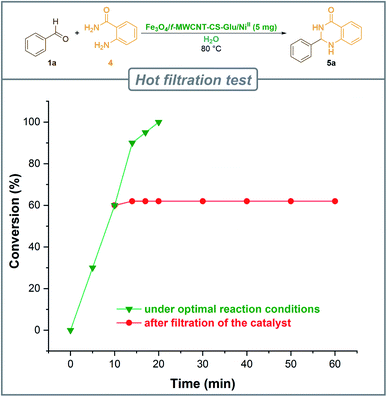 |
| Fig. 11 Hot filtration test for the heterogeneity investigation of the Fe3O4/f-MWCNT-CS-Glu/NiII nanocomposite catalytic system. | |
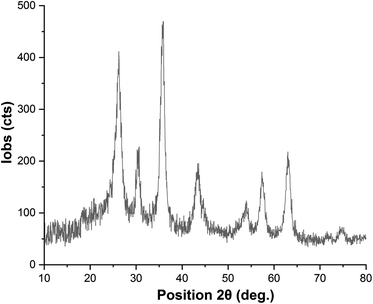 |
| Fig. 12 PXRD diagram of the recycled Fe3O4/f-MWCNT-CS-Glu/NiII nanocomposite. | |
2.4. Comparative study
To show the high value and efficiency of the presented Fe3O4/f-MWCNT-CS-Glu/NiII nanocomposite as a gigantic nanocatalytic system in the above-mentioned heterocyclic frameworks one-pot preparation, we compared it with some previously reported protocols on the synthesis of 3a, 5a, 7a, and 9a. As shown in Table 5, the new synthetic protocols which presented in this paper are better than others in terms of the catalyst loading, reaction time, favorable yield, use of green reaction mediums (water or solvent-free), and so on.
Table 5 Comparison of the catalytic activity of the as-prepared Fe3O4/f-MWCNT-CS-Glu/NiII nanocomposite with literature samples reported for the synthesis of 3a, 5a, 7a, and 9a
Entry |
TM |
Catalyst |
Solvent |
Reaction conditions |
Time (min) |
Yield (%) |
Ref |
Present work. TM = Target molecule. MW = Microwave. US = Ultrasonic. |
1 |
3a |
Fe3O4/f-MWCNT-CS-Glu/NiII (5 mg) |
H2O |
Reflux |
10 |
95 |
a |
2 |
3a |
HAP/Fe3O4 (5 mg) |
Solvent-free |
90 °C |
60 |
95 |
32 |
3 |
3a |
BFA (10 wt%) |
H2O |
Room temperature |
15 |
92 |
33 |
4 |
3a |
Ni0-Mont (20 mg) |
Solvent-free |
MW (850 W) |
5 |
95 |
34 |
5 |
3a |
Carbon-SO3H (30 mg) |
H2O : CH3CH2OH (1 : 1) |
80 °C |
10 |
97 |
35 |
6 |
3a |
P4VPy-CuO (20 mg) |
H2O |
Reflux |
20 |
90 |
36 |
7 |
3a |
RHA-SO3H (40 mg) |
H2O |
80 °C |
15 |
90 |
37 |
8 |
3a |
SO3H@Fe3O4 (15 mg) |
Solvent-free |
80 °C |
10 |
92 |
38 |
9 |
3a |
SDS (20 mol%) |
H2O |
60 °C |
120 |
85 |
39 |
10 |
3a |
Fe(SD)3 (20 mol%) |
H2O |
100 °C |
10 |
79 |
40 |
11 |
3a |
Fe3O4@SiO2@Im-bisethylFc [HC2O4] (4 mg) |
CH3CH2OH |
Reflux |
60 |
96 |
41 |
12 |
3a |
Vitamin B1 (1 mol%) |
Solvent-free |
Room temperature |
60 |
92 |
42 |
13 |
3a |
(N2H5)2SiF6 (0.2 mol%) |
CH3CH2OH |
Reflux |
3 |
95 |
43 |
14 |
5a |
Fe3O4/f-MWCNT-CS-Glu/NiII (5 mg) |
H2O |
80 °C |
20 |
98 |
a |
15 |
5a |
UiO-66-MW (1.2 mg) |
CH3CO2H |
60 °C |
240 |
85 |
44 |
16 |
5a |
VO-vanillin-MCM-41 (20 mg) |
H2O |
90 °C |
130 |
96 |
45 |
17 |
5a |
TiCl3/NFC (40 mg) |
CH3CH2OH |
Reflux |
20 |
85 |
46 |
18 |
5a |
Ompg-C3N4/SO3H (20 mg) |
CH3CH2OH |
Reflux |
3 |
98 |
47 |
19 |
5a |
Zr-MOF/rGO (20 mg) |
CH3CH2OH |
55 °C |
30 |
95 |
48 |
20 |
5a |
SBA-15@n-Pr-THAM-ZrO (7 mg) |
CH3CH2OH |
Reflux |
80 |
93 |
49 |
21 |
5a |
CoFe2O4@PrIII (50 mg) |
CH3CH2OH |
Reflux |
60 |
97 |
50 |
22 |
5a |
Ascorbic acid (10 mol%) |
H2O |
90 °C |
60 |
92 |
51 |
23 |
5a |
Fe3O4@NCs-PA (40 mg) |
H2O : CH3CH2OH (1 : 1) |
Reflux |
90 |
83 |
52 |
24 |
5a |
CoFe2O4@SiO2-CPTES-guanidine-CuII (8 mg) |
H2O |
80 °C |
20 |
96 |
53 |
25 |
5a |
Cu(NO3)2/Fe3O4-EDTA (10 mg) |
H2O |
Reflux |
30 |
98 |
54 |
26 |
7a |
Fe3O4/f-MWCNT-CS-Glu/NiII (10 mg) |
Solvent-free |
110 °C |
40 |
96 |
a |
27 |
7a |
Ag@Sep-N-CH (30 mg) |
H2O : CH3CH2OH (1 : 2) |
50 °C |
180 |
95 |
55 |
28 |
7a |
Ag@CDNS-N/PMelamine (30 mg) |
H2O : CH3CH2OH (1 : 2) |
50 °C |
180 |
92 |
56 |
29 |
7a |
SA@Sawdust (50 mg) |
CH3CH2OH |
Reflux |
50 |
92 |
57 |
30 |
7a |
[SO3H-pyrazine-SO3H]Cl2 (0.25 mmol) |
Solvent-free |
110 °C |
35 |
93 |
58 |
31 |
7a |
[TMXH]FeCl4 (0.25 mmol) |
Solvent-free |
110 °C |
10 |
92 |
59 |
32 |
7a |
UiO2-66-NH2-ILP F6−-guanidine (30 mg) |
CH3CH2OH |
25 °C |
45 |
95 |
60 |
33 |
7a |
Fe3O4@THAM-piperazine (10 mg) |
H2O : CH3CH2OH (1 : 1) |
100 °C |
15 |
88 |
61 |
34 |
7a |
NiFeTi CLDH6 (20 mg) |
CH3CH2OH |
80 °C |
60 |
96 |
62 |
35 |
7a |
Fe-X (25 mg) |
Solvent-free |
90 °C |
15 |
95 |
63 |
36 |
7a |
Fe3O4@NFC@NNS-MnII (10 mg) |
CH3CH2OH |
45 °C |
10 |
98 |
64 |
37 |
7a |
CuII-FUR-APTES/GO (20 mg) |
H2O : CH3CH2OH (1 : 1) |
50 °C |
30 |
95 |
65 |
38 |
9a |
Fe3O4/f-MWCNT-CS-Glu/NiII (10 mg) |
Solvent-free |
110 °C |
10 |
97 |
a |
39 |
9a |
Fe3O4@MCM-41@Zr (30 mg) |
H2O : CH3CH2OH (3 : 7) |
75 °C |
40 |
74 |
66 |
40 |
9a |
Zn2SnO4–SnO2 (25 mg) |
CH3CH2OH |
US (80 °C) |
120 |
80 |
67 |
41 |
9a |
AMBA-Fe3O4 (50 mg) |
CH3CH2OH |
60 °C |
21 |
92 |
68 |
42 |
9a |
HMS/Pr-Rh-Zr (50 mg) |
PEG |
80 °C |
30 |
87 |
69 |
43 |
9a |
NH2@SiO2@Fe3O4 (10 mg) |
Solvent-free |
Grinding |
4 |
94 |
70 |
44 |
9a |
BaFe12O19@IM (12 mg) |
CH3CH2OH |
Reflux |
20 |
88 |
71 |
45 |
9a |
MNPs-PhSO3H (10 mg) |
H2O : CH3CH2OH (1 : 1) |
100 °C |
10 |
91 |
72 |
46 |
9a |
Bis-Su (10 mg) |
H2O : CH3CH2OH (1 : 1) |
80 °C |
35 |
84 |
73 |
47 |
9a |
Ni@Fe-doped CeO2/Chitosan (10 wt%) |
CH3CH2OH |
60 °C |
10 |
90 |
74 |
48 |
9a |
Fe3O4@SiO2-guanidine-PAA (50 mg) |
H2O |
70 °C |
35 |
96 |
75 |
49 |
9a |
Fe3O4@GOQD-O-(propane-1-sulfonic acid) (50 mg) |
H2O |
Room temperature |
35 |
93 |
76 |
3. Experimental
3.1. Reagents, samples, and apparatus
All starting materials, reagents, and solvents were commercially available (purchased from Merck, Sigma-Aldrich, and Fluka companies) and used directly without further purification. SOLTEC SONICA 2400 MH S3 (300 W) instrument was used for ultrasonic irradiation. FT-IR spectra were recorded on Thermo Nicolet Nexus 670 spectrometer, and 1H NMR spectra were obtained by Bruker Avance 300 MHz and 400 MHz spectrometer. The crystalline structures of the prepared nanocomposites were analyzed by powder X-ray diffraction (PXRD) on a Philips PANalytical X'PertPro diffractometer (Netherlands) in 40 kV and 30 mA with a monochromatized Cu Kα radiation (λ = 1.5418 Å). The SEM images, EDX diagram, and elemental mapping obtained from FESEM-TESCAN MIRA3 electronic microscope. The TEM images were obtained from Zeiss EM10C-100 kV transmission electron microscope. The elemental analysis was carried out by inductively coupled plasma-optical emission spectrometry (Optima 7300DV ICP-OES). The magnetic properties of the prepared samples were measured using a vibrating sample magnetometer (Meghnatis Daghigh, Iran) under magnetic fields up to 20 kOe.
3.2. One-pot preparation of the Fe3O4/f-MWCNT-CS-Glu nanocomposite
Firstly, in a three-necked round-bottom flask (250 mL), MWCNT-CO2H (0.48 g) was dispersed in deionized water (100 mL) by ultrasonic irradiation for thirty minutes. Then, Fe3O4·6H2O (0.6 g) and FeCl2·4H2O (0.45 g) were added into the mentioned pot and sonicated for another thirty minutes. After that, the 40 mL ammonia solution was added portion wisely into the reaction environment at 60 °C under a nitrogen atmosphere and stirred for two hours. In the next step, the dissolved chitosan (0.18 g) in two percent acetic acid solution was added into the mentioned three-necked round-bottom flask and stirred for two hours at 60 °C under the nitrogen atmosphere. Finally, L-glutamic acid (0.2 g) was added to the reaction mixture and stirred for twenty hours under the same temperature and atmospheric conditions. The prepared Fe3O4/f-MWCNT-CS-Glu nanocomposite collected from the reaction pot, washed with deionized water and ethanol, and then dried at 50 °C.
3.3. Preparation of the Fe3O4/f-MWCNT-CS-Glu/NiII nanocomposite
In a round-bottom flask (100 mL), the prepared Fe3O4/f-MWCNT-CS-Glu (0.5 g) was dispersed in a 1
:
2 mixture of water and ethanol (70 mL) by ultrasonic irradiation for ten minutes. Next, nickelII nitrate hexahydrate (Ni(NO3)2·6H2O) (0.25 g) was added into the mentioned reaction pot and sonicated for another forty-five minutes, and then stirred for twenty-four hours at 60 °C under air atmosphere. The as-prepared Fe3O4/f-MWCNT-CS-Glu/NiII nanocomposite collected from the reaction environment, washed with deionized water and ethanol, and then dried at 50 °C.
3.4. General procedure for the one-pot pseudo-three-component synthesis of bis-coumarins (3a–n) catalyzed by the as-prepared Fe3O4/f-MWCNT-CS-Glu/NiII nanocomposite
As a representative example, in a round-bottom flask (10 mL), which equipped with a magnetic stirrer, a mixture of benzaldehyde (1 mmol), 4-hydroxycoumarin (2 mmol), and the as-prepared Fe3O4/f-MWCNT-CS-Glu/NiII (5 mg) in the water solvent (3 mL) was prepared and stirred under reflux conditions for an appropriate time (10 minutes). As soon as the mentioned one-pot reaction was completed, the Fe3O4/f-MWCNT-CS-Glu/NiII nanocatalyst was separated from the reaction pot using an external magnet. Then, after cooling to room temperature, the resulting mixture was extracted with ethanol (2 × 5 mL), followed by drying over anhydrous sodium sulfate (Na2SO4). The solvent evaporation under reduced pressure affords the crude 3,3′-(phenylmethylene)bis(4-hydroxy-2H-chromen-2-one) (3a) product, which was subsequently purified by recrystallization from hot ethanol.
3.5. General procedure for the one-pot two-component synthesis of 2-aryl(or heteroaryl)-2,3-dihydroquinazolin-4(1H)-ones (5a–r) catalyzed by the as-prepared Fe3O4/f-MWCNT-CS-Glu/NiII nanocomposite
As a representative example, in a round-bottom flask (10 mL), which equipped with a magnetic stirrer, a mixture of benzaldehyde (1 mmol), anthranilamide (1 mmol), and the as-prepared Fe3O4/f-MWCNT-CS-Glu/NiII (5 mg) in the water solvent (3 mL) was prepared and stirred at 80 °C for twenty minutes. As soon as the mentioned one-pot reaction was completed, the Fe3O4/f-MWCNT-CS-Glu/NiII nanocatalyst was separated from the reaction pot using an external magnet. Then, after cooling to room temperature, the resulting mixture was extracted with ethanol (2 × 5 mL), followed by drying over Na2SO4. The solvent evaporation under reduced pressure affords the crude 2-phenyl-2,3-dihydroquinazolin-4(1H)-one (5a) product, which was subsequently purified by recrystallization from hot ethanol.
3.6. General procedure for the one-pot pseudo-three-component synthesis of 9-aryl-3,3,6,6-tetramethyl-3,4,5,6,7,9-hexahydro-1H-xanthene-1,8(2H)-diones (7a–n) catalyzed by the as-prepared Fe3O4/f-MWCNT-CS-Glu/NiII nanocomposite
As a representative example, in a simple experimental tube, which equipped with a magnetic stirrer, a mixture of benzalaldehyde (1 mmol), 5,5-dimethyl-1,3-cyclohexanedione (dimedone) (2 mmol), and the as-prepared Fe3O4/f-MWCNT-CS-Glu/NiII (10 mg) was prepared and heated at 110 °C under solvent-free conditions for forty minutes. After completion of the mentioned one-pot pseudo-three-component reaction and cooling it to room temperature, ethanol (3 mL) was added and the reaction mixture was stirred for two minutes. Then, the Fe3O4/f-MWCNT-CS-Glu/NiII nanocatalyst was magnetically separated from the reaction environment. Next, the resulting solution was dried over Na2SO4. The solvent evaporation under reduced pressure affords the pure 9-phenyl-3,3,6,6-tetramethyl-3,4,5,6,7,9-hexahydro-1H-xanthene-1,8(2H)-dione (7a) product.
3.7. General procedure for the one-pot three-component synthesis of 2-amino-4-aryl-7,7-dimethyl-5-oxo-5,6,7,8-tetrahydro-4H-chromene-3-carbonitriles (9a–n) catalyzed by the as-prepared Fe3O4/f-MWCNT-CS-Glu/NiII nanocomposite
As a representative example, in a simple experimental tube, which equipped with a magnetic stirrer, a mixture of benzalaldehyde (1 mmol), malononitrile (1 mmol), 5,5-dimethyl-1,3-cyclohexanedione (dimedone) (1 mmol), and the as-prepared Fe3O4/f-MWCNT-CS-Glu/NiII (10 mg) was prepared and heated at 110 °C under solvent-free conditions for ten minutes. After completion of the mentioned one-pot three-component reaction and cooling it to room temperature, ethanol (3 mL) was added and the reaction mixture was stirred for two minutes. Then, the Fe3O4/f-MWCNT-CS-Glu/NiII nanocatalyst was magnetically separated from the reaction environment. Next, the resulting solution was dried over Na2SO4. The solvent evaporation under reduced pressure affords the pure 2-amino-4-phenyl-7,7-dimethyl-5-oxo-5,6,7,8-tetrahydro-4H-chromene-3-carbonitrile (9a) product.
4. Conclusions
In summary, a novel magnetic nickelII-containing nanocatalyst denoted as Fe3O4/f-MWCNT-CS-Glu/NiII was successfully fabricated and characterized by various techniques, including FT-IR, PXRD, SEM, TEM, SEM-based EDX and elemental mapping, ICP-OES, TGA/DTA, and VSM. Interestingly, the as-prepared Fe3O4/f-MWCNT-CS-Glu/NiII hybrid nanosystem was an excellent catalyst for the preparation of various type of heterocyclic compounds, including bis-coumarins (3a–n), 2-aryl(or heteroaryl)-2,3-dihydroquinazolin-4(1H)-ones (5a–r), 9-aryl-3,3,6,6-tetramethyl-3,4,5,6,7,9-hexahydro-1H-xanthene-1,8(2H)-diones (7a–n), and 2-amino-4-aryl-7,7-dimethyl-5-oxo-5,6,7,8-tetrahydro-4H-chromene-3-carbonitriles (9a–n) in good-to-excellent yields and satisfactory TONs and TOFs through the convenient and green one-pot multi-component synthetic procedures. Notably, other catalytic applications of the mentioned Fe3O4/f-MWCNT-CS-Glu/NiII magnetic nanocomposite are currently under investigation in our lab and will be reported in due course.
Conflicts of interest
There are no conflicts to declare.
Acknowledgements
The author thanks Urmia University for support of this work.
References
-
(a) W. Wang, Q. Meng, Q. Li, J. Liu, M. Zhou, Z. Jin and K. Zhao, Int. J. Mol. Sci., 2020, 21, 487 CrossRef CAS PubMed;
(b) L. A. Frank, G. R. Onzi, A. S. Morawski, A. R. Pohlmann, S. S. Guterres and R. V. Contri, React. Funct. Polym., 2020, 147, 104459 CrossRef CAS;
(c) Z. Shariatinia, Int. J. Biol. Macromol., 2018, 120, 1406–1419 CrossRef CAS;
(d) B. Sharma, S. Sharma and P. Jain, Int. J. Biol. Macromol., 2021, 169, 414–427 CrossRef CAS PubMed;
(e) L. Tian, A. Singh and A. V. Singh, Int. J. Biol. Macromol., 2020, 153, 533–538 CrossRef CAS PubMed;
(f) M. Kurakula and N. R. Naveen, Int. J. Biol. Macromol., 2020, 165, 1924–1938 CrossRef CAS PubMed;
(g) S. Kumar, J. Dutta, P. K. Dutta and J. Koh, Int. J. Biol. Macromol., 2020, 160, 470–481 CrossRef CAS PubMed;
(h) A. B. da Silva, K. B. Rufato, A. C. de Oliveira, P. R. Souza, E. P. da Silva, E. C. Muniz, B. H. Vilsinski and A. F. Martins, Int. J. Biol. Macromol., 2020, 161, 977–998 CrossRef CAS PubMed;
(i) X. Wang, H. S. Almoallim, Q. Cui, S. A. Alharbi and H. Yang, Int. J. Biol. Macromol., 2021, 171, 198–207 CrossRef CAS.
-
(a) D. Wu, L. Zhu, Y. Li, X. Zhang, S. Xu, G. Yang and T. Delair, Carbohydr. Polym., 2020, 238, 116126 CrossRef CAS;
(b) R. Shanmuganathan, T. N. J. I. Edison, F. LewisOscar, P. Kumar, S. Shanmugam and A. Pugazhendhi, Int. J. Biol. Macromol., 2019, 130, 727–736 CrossRef CAS PubMed;
(c) A. Ali and S. Ahmed, Int. J. Biol. Macromol., 2018, 109, 273–286 CrossRef CAS PubMed;
(d) S. Pramanik and V. Sali, Int. J. Biol. Macromol., 2021, 169, 103–121 CrossRef CAS PubMed.
-
(a) N. Saranya, A. Moorthi, S. Saravanan, M. P. Devi and N. Selvamurugan, Int. J. Biol. Macromol., 2011, 48, 234–238 CrossRef CAS;
(b) D. Chuan, T. Jin, R. Fan, L. Zhou and G. Guo, Adv. Colloid Interface Sci., 2019, 268, 25–38 CrossRef.
-
(a) I. M. van der Lubben, J. C. Verhoef, G. Borchard and H. E. Junginger, Eur. J. Pharm. Sci., 2001, 14, 201–207 CrossRef CAS PubMed;
(b) R. C. Red, S. C. Naylor, C. W. Potter, J. Bond, I. Jabbal-Gill, A. Fisher, L. Illum and R. Jennings, Vaccine, 2005, 23, 4367–4374 CrossRef;
(c) L. Xing, Y.-T. Fan, T. J. Zhou, J.-H. Gong, L.-H. Cui, K.-H. Cho, Y.-J. Choi, H. Jiang and C.-S. Cho, Molecules, 2018, 23, 229 CrossRef PubMed.
-
(a) M. Cicciù, L. Fiorillo and G. Cervino, Mar. Drugs, 2019, 17, 417 CrossRef;
(b) E. Fakhri, H. Eslami, P. Maroufi, F. Pakdel, S. Taghizadeh, K. Gunbarov, M. Yousefi, A. Tanomand, B. Yousefi, S. Mahmoudi and H. Samadi Kafil, Int. J. Biol. Macromol., 2020, 162, 956–974 CrossRef CAS;
(c) C. Zhang, D. Hui, C. Du, H. Sun, W. Peng, X. Pu, Z. Li, J. Sun and C. Zhou, Int. J. Biol. Macromol., 2021, 167, 1198–1210 CrossRef CAS PubMed.
-
(a) A. Di Martino, M. Sittinger and M. V. Risbud, Biomaterials, 2005, 26, 5983–5990 CrossRef CAS PubMed;
(b) J.-K. F. Suh and H. W. T. Matthew, Biomaterials, 2000, 21, 2589–2598 CrossRef CAS;
(c) J. Venkatesan and S.-K. Kim, Mar. Drugs, 2010, 8, 2252–2266 CrossRef CAS PubMed;
(d) S. Ranganthan, K. Balagangadharan and N. Selvamurugan, Int. J. Biol. Macromol., 2019, 133, 354–364 CrossRef PubMed;
(e) S. Khan, M. Garg, S. Chockalingam, P. Gopinath and P. P. Kundu, Int. J. Biol. Macromol., 2020, 143, 285–296 CrossRef CAS;
(f) M. Tamimi, S. Rajabi and M. Pezeshki-Modaress, Int. J. Biol. Macromol., 2020, 164, 389–402 CrossRef CAS PubMed.
-
(a) A. Moeini, P. Pedram, P. Makvandi, M. Malinconico and G. Gomez d'Ayala, Carbohydr. Polym., 2020, 233, 115839 CrossRef CAS PubMed;
(b) S. S. Biranje, P. V. Madiwale, K. C. Patankar, R. Chhabra, P. Dandekar-Jain and R. V. Adivarekar, Int. J. Biol. Macromol., 2019, 121, 936–946 CrossRef CAS;
(c) M. Ishihara, K. Nakanishi, K. Ono, M. Sato, M. Kikuchi, Y. Saito, H. Yura, T. Matsui, H. Hattori, M. Uenoyama and A. Kurita, Biomaterials, 2002, 23, 833–840 CrossRef CAS;
(d) P. S. Kaparekar, S. Pathmanapan and S. K. Anandasadagopan, Int. J. Biol. Macromol., 2020, 165, 930–947 CrossRef CAS;
(e) A. Mazloom-Jalili, Z. Shariatinia, I. Ashrafi Tamai, S.-R. Pakzad and J. Malakootikhah, Int. J. Biol. Macromol., 2020, 153, 421–432 CrossRef PubMed;
(f) N. Sakthiguru and M. A. Sithique, Int. J. Biol. Macromol., 2020, 152, 873–883 CrossRef CAS.
-
(a) P. M. Pakdel and S. J. Peighambardoust, Carbohydr. Polym., 2018, 201, 264–279 CrossRef;
(b) J. Desbrières and E. Guibal, Polym. Int., 2018, 67, 7–14 CrossRef;
(c) S. Sarode, P. Upadhyay, M. A. Khosa, T. Mak, A. Shakir, S. Song and A. Ullah, Int. J. Biol. Macromol., 2019, 121, 1086–1100 CrossRef CAS PubMed.
-
(a) R. L. Kashyap, X. Xiang and P. Heiden, Int. J. Biol. Macromol., 2015, 77, 36–51 CrossRef PubMed;
(b) B. Qu and Y. Luo, Int. J. Biol. Macromol., 2020, 152, 437–448 CrossRef CAS PubMed;
(c) K. B. M. Ahmed, M. M. A. Khan, H. Siddiqui and A. Jahan, Carbohydr. Polym., 2020, 227, 115331 CrossRef;
(d) R. C. Choudhary, R. V. Kumaraswamy, S. Kumari, S. S. Sharma, A. Pal, R. Raliya, P. Biswas and V. Saharan, Int. J. Biol. Macromol., 2019, 127, 126–135 CrossRef CAS PubMed;
(e) G. L. Vanti, S. Masaphy, M. Kurjogi, S. Chakrasali and V. B. Nargund, Int. J. Biol. Macromol., 2020, 156, 1387–1395 CrossRef CAS PubMed.
-
(a) K. Rambabu, G. Bharath, F. Banat, P. L. Show and H. Hernández Cocoletzi, Int. J. Biol. Macromol., 2019, 126, 1234–1243 CrossRef CAS;
(b) S. Kumar, A. Makherjee and J. Dutta, Trends Food Sci. Technol., 2020, 97, 196–209 CrossRef CAS;
(c) H. Wang, J. Qian and F. Ding, J. Agric. Food Chem., 2018, 66, 395–413 CrossRef CAS PubMed;
(d) L. O. Xavier, W. G. Sganzerla, G. B. Rosa, C. G. Da Rosa, L. Agostinetto, A. P. De Lima Veeck, L. C. Bretanha, G. A. Micke, M. D. Costa, F. C. Bertodi, P. L. M. Barreto and M. R. Nunes, Int. J. Biol. Macromol., 2021, 169, 183–193 CrossRef CAS.
-
(a) H. Mousavi, Int. J. Biol. Macromol., 2021, 186, 1003–1166 CrossRef CAS PubMed;
(b) Á. Molnár, Coord. Chem. Rev., 2019, 388, 126–171 CrossRef;
(c) A. Dhakshinamoorthy, M. Jacob, N. S. Vignesh and P. Varalakshmi, Int. J. Biol. Macromol., 2021, 167, 807–833 CrossRef CAS PubMed;
(d) M. Dohendou, K. Pakzad, Z. Nezafat, M. Nasrollahzadeh and M. G. Dekamin, Int. J. Biol. Macromol., 2021, 192, 771–819 CrossRef CAS PubMed.
- F. Ghaffarian, M. A. Ghasemzadeh and S. S. Aghaei, J. Mol. Struct., 2019, 1186, 204–211 CrossRef CAS.
- L.-G. Ding, B.-J. Yao, F. Li, S.-C. Shi, N. Huang, H.-B. Yin, Q. Guan and Y.-B. Dong, J. Mater. Chem. A, 2019, 7, 4689–4698 RSC.
-
(a) M. Maleki and R. Paydar, RSC Adv., 2015, 5, 33177–33184 RSC;
(b) A. Maleki and R. Paydar, React. Funct. Polym., 2016, 109, 120–124 CrossRef CAS;
(c) S. Kumar, M. Y. Wani, J. Koh, J. M. Gil and A. J. F. N. Sobral, J. Environ. Sci., 2018, 69, 77–84 CrossRef CAS PubMed;
(d) M. Mahdavinasab, M. Hamzehlouian and Y. Sarrafi, Int. J. Biol. Macromol., 2019, 138, 764–772 CrossRef CAS PubMed.
-
(a) J. Sun, J. Wang, W. Cheng, J. Zhang, X. Li, S. Zhang and Y. She, Green Chem., 2012, 14, 654–660 RSC;
(b) C. Jing-Xian, J. Bi, D. Wei-Li, D. Sen-Lin, C. Liu-Ren, C. Zong-Jie, L. Sheng-Lian, L. Xu-Biao, T. Xin-Man and A. Chak-Tong, Appl. Catal., A, 2014, 484, 26–32 CrossRef;
(c) M. Taheri, M. Ghiaci and A. Shchukarev, New J. Chem., 2018, 42, 587–597 RSC;
(d) M. U. Khan, S. Siddiqui and Z. N. Siddiqui, ACS Omega, 2019, 4, 7586–7595 CrossRef CAS PubMed;
(e) A. Khalafi-Nezhad and S. Mohammadi, RSC Adv., 2013, 3, 4362–4371 RSC.
-
(a) M. Chtchigrovsky, A. Primo, P. Gonzalez, K. Molvinger, M. Robitzer, F. Quignard and F. Taran, Angew. Chem., Int. Ed., 2009, 48, 5916–5920 CrossRef CAS PubMed;
(b) Anuradha, S. Layek, B. Agrahari and D. D. Pathak, ChemistrySelect, 2017, 2, 6865–6876 CrossRef CAS;
(c) H. Naeimi and S. Lahouti, Appl. Organomet. Chem., 2017, 31, e3732 CrossRef;
(d) H. Naeimi and S. Lahouti, Transition Met. Chem., 2018, 43, 221–229 CrossRef CAS;
(e) J. Rakhtshah and F. Yaghoobi, Int. J. Biol. Macromol., 2019, 139, 904–916 CrossRef CAS PubMed.
- K. C. Basavaraju, S. Sharma, A. K. Singh, D. J. Im and D.-P. Kim, ChemSusChem, 2014, 7, 1864–1869 CrossRef CAS PubMed.
-
(a) S. Philippe and E. Castillejos, ChemCatChem, 2010, 2, 41–47 CrossRef;
(b) V. Campisciano, M. Gruttadauria and F. Giacalone, ChemCatChem, 2019, 11, 90–133 CrossRef CAS;
(c) A. C. Ghogia, A. Nzihou, P. Serp, K. Soulantica and D. P. Minh, Appl. Catal., A, 2021, 609, 117906 CrossRef CAS;
(d) S. Ansari, A. Khorshidi and S. Shariati, RSC Adv., 2020, 10, 3554–3565 RSC;
(e) M. S. Ghasemzadeh and B. Akhlaghinia, ChemistrySelect, 2019, 4, 1542–1555 CrossRef CAS.
-
(a) S. Chatterjee, M. W. Lee and S. H. Woo, Carbon, 2009, 47, 2933–2939 CrossRef CAS;
(b) S. Karbasi and Z. M. Alizadeh, Bull. Mater. Sci., 2017, 40, 1247–1253 CrossRef CAS;
(c) I. M. Garnica-Palafox, H. O. Estrella-Monroy, N. A. Vázquez-Torres, M. Áalvarez-Camacho, A. E. Castell-Rodríguez and F. M. Sánchez-Arévalo, Carbohydr. Polym., 2020, 236, 115971 CrossRef CAS PubMed.
-
(a) V. Polshetiwar, R. Luque, A. Fihri, H. Zhu, M. Bouhara and J.-M. Basset, Chem. Rev., 2011, 111, 3036–3075 CrossRef PubMed;
(b) S. Shylesh, V. Schünemann and W. R. Thiel, Angew. Chem., Int. Ed., 2010, 49, 3428–3459 CrossRef CAS PubMed;
(c) D. Wang and D. Astruc, Chem. Rev., 2014, 114, 6949–6985 CrossRef CAS PubMed;
(d) M. B. Gawande, R. Luque and R. Zboril, ChemCatChem, 2014, 6, 3312–3313 CrossRef CAS;
(e) B. Karimi, F. Mansouri and H. M. Mirzaei, ChemCatChem, 2015, 7, 1736–1789 CrossRef CAS;
(f) G. Mohammadi Ziarani, Z. Kheilkordi, F. Mohajer, A. Badiei and R. Luque, RSC Adv., 2021, 11, 17456–17477 RSC;
(g) A. Pawar, S. Gajare, A. Patil, R. Kurane, G. Rashinkar and S. Patil, Res. Chem. Intermed., 2021, 47, 2801–2820 CrossRef CAS;
(h) M. Aghaei-Hashjin, A. Yahyazadeh and E. Abbaspour-Gilandeh, RSC Adv., 2021, 11, 23491–23505 RSC;
(i) F. Mohammadsaleh, M. Dehdashti Jahromi, A. R. Hajipour, S. M. Hosseini and K. Niknam, RSC Adv., 2021, 11, 20812–20823 RSC;
(j) N. Hosseini Mohtasham and M. Gholizadeh, Res. Chem. Intermed., 2021, 47, 2507–2525 CrossRef CAS;
(k) A. Naikwade, M. Jagadale, D. Kale and G. Rashinkar, Aust. J. Chem., 2020, 73, 1088–1097 CrossRef CAS;
(l) M. Mohammadi and A. Ghorbani-Choghamarani, RSC Adv., 2022, 12, 2770–2787 RSC.
-
(a) B. Eftekhari-Sis, M. Zirak and A. Akbari, Chem. Rev., 2013, 113, 2958–3043 CrossRef CAS PubMed;
(b) B. Eftekhari-Sis and M. Zirak, Chem. Rev., 2015, 115, 151–264 CrossRef CAS PubMed.
-
(a) A. Dömling, W. Wang and K. Wang, Chem. Rev., 2012, 112, 3083–3135 CrossRef PubMed;
(b) S. Abu-Melha, Z. A. Muhammad, A. S. Abouzid, M. M. Edrees, A. S. Abo Dena, S. Nabil and S. M. Gomha, J. Mol. Struct., 2021, 1234, 130180 CrossRef CAS;
(c) H. G. O. Alvim, E. N. da Silva Júnior and B. A. D. Neto, RSC Adv., 2014, 4, 54282–54299 RSC;
(d) S. Kamalifar and H. Kiyani, Res. Chem. Intermed., 2019, 45, 5975–5987 CrossRef CAS;
(e) L. Wu, S. Yan, W. Wang and Y. Li, Res. Chem. Intermed., 2020, 46, 4311–4322 CrossRef CAS;
(f) A. Patil, S. Shinde, G. Rashinkar and R. Salunkhe, Res. Chem. Intermed., 2020, 46, 63–73 CrossRef CAS;
(g) S. Zhi, X. Ma and W. Zhang, Org. Biomol. Chem., 2019, 17, 7632–7650 RSC;
(h) N. Nikooei, M. G. Dekamin and E. Valiey, Res. Chem. Intermed., 2020, 46, 3891–3909 CrossRef CAS;
(i) M. Rimaz and H. Mousavi, Turk. J. Chem., 2013, 37, 252–261 CAS;
(j) M. Rimaz, H. Mousavi, L. Ozzar and B. Khalili, Res. Chem. Intermed., 2019, 45, 2673–2694 CrossRef CAS;
(k) M. Rimaz, H. Mousavi, B. Khalili and L. Sarvari, J. Iran. Chem. Soc., 2019, 16, 1687–1701 CrossRef CAS;
(l) M. Rimaz, B. Khalili, G. Khatyal, H. Mousavi and F. Aali, Aust. J. Chem., 2017, 70, 1274–1284 CrossRef CAS;
(m) M. Rimaz, H. Mousavi, M. Behnam, L. Sarvari and B. Khalili, Curr. Chem. Lett., 2017, 6, 55–68 CrossRef;
(n) M. Rimaz, H. Mousavi, L. Nikpey and B. Khalili, Res. Chem. Intermed., 2017, 43, 3925–3937 CrossRef CAS;
(o) M. Rimaz, H. Mousavi, P. Keshavarz and B. Khalili, Curr. Chem. Lett., 2015, 4, 159–168 CrossRef;
(p) P. Mane, B. Shinde, P. Mundada, B. Karale and A. Burungale, Res. Chem. Intermed., 2021, 47, 1743–1758 CrossRef CAS;
(q) I. Yellapurkar, S. Bhabal, M. M. V. Ramana, K. Jangam, V. Salve, S. Patange and P. More, Res. Chem. Intermed., 2021, 47, 2669–2687 CrossRef CAS;
(r) R. L. Mohlala, E. M. Coyanis, M. A. Fernandes and M. L. Bode, RSC Adv., 2021, 11, 24466–24473 RSC;
(s) F. Haji Norouzi, N. Foroughifar, A. Khajeh-Amiri and H. Pasdar, RSC Adv., 2021, 11, 29948–29959 RSC;
(t) B. M. Bizzarri, A. Fanelli, L. Botta, M. De Angelis, A. T. Palamara, L. Nencioni and R. Saladino, RSC Adv., 2021, 11, 30020–30029 RSC;
(u) Z. Ghanbari and H. Naeimi, RSC Adv., 2021, 11, 31377–31384 RSC;
(v) P. Ghamari Kargar, G. Bagherzade and H. Eshghi, RSC Adv., 2021, 11, 4339–4355 RSC;
(w) P. Ghamari Kargar and G. Bagherzade, RSC Adv., 2021, 11, 23192–23206 RSC;
(x) P. Ghamari Kargar, M. Noorian, E. Chamani, G. Bagherzade and Z. Kiani, RSC Adv., 2021, 11, 17413–17430 RSC;
(y) S. D. Dhengale, C. V. Rode, G. B. Kolekar and P. V. Anbhule, RSC Adv., 2022, 12, 2083–2093 RSC;
(z) A. Ghasemi-Ghahsareh, J. Safaei-Ghomi and H. S. Oboudatian, RSC Adv., 2022, 12, 1319–1330 RSC;
(a
a) S. K. Dangolian, E. Niknam, O. Shahraki and A. Khalafi-Nezhad, J. Mol. Struct., 2021, 1245, 131061 CrossRef;
(a
b) F. Kamali and F. Shirini, J. Mol. Struct., 2021, 1227, 129654 CrossRef CAS;
(a
c) S. Saeedi and A. Rahmati, RSC Adv., 2022, 12, 11740–11749 RSC;
(a
d) B. Borah, J. Bora, P. Ramesh and L. R. Chowhan, RSC Adv., 2022, 12, 12843–12857 RSC;
(a
e) M. Rimaz, H. Mousavi, B. Khalili and F. Aali, J. Chin. Chem. Soc., 2018, 65, 1389–1397 CrossRef CAS.
-
(a) H. C. Erythropel, J. B. Zimmerman, T. M. de Winter, L. Petitjean, F. Melnikov, C. H. Lam, A. W. Lounsbury, K. E. Mellor, N. Z. Janković, Q. Tu, L. N. Pincus, M. M. Falinski, W. Shi, P. Coish, D. L. Plata and P. A. Anastas, Green Chem., 2018, 20, 1929–1961 RSC;
(b) M. Pérez-Venegas and E. Juaristi, ACS Sustainable Chem. Eng., 2020, 8, 8881–8893 CrossRef;
(c) M. C. Bryan, B. Dillon, L. G. Hamann, G. J. Hughes, M. E. Kopach, E. A. Peterson, M. Pourashraf, I. Raheem, P. Richardson, D. Richter and H. F. Sheddon, J. Med. Chem., 2013, 56, 6007–6021 CrossRef CAS PubMed;
(d) G. Náray-Szabó and L. T. Mika, Green Chem., 2018, 20, 2171–2191 RSC;
(e) P. T. Anastas and J. B. Zimmerman, Green Chem., 2019, 21, 6545–6566 RSC;
(f) M. Rimaz, Z. Jalalian, H. Mousavi and R. H. Prager, Tetrahedron Lett., 2016, 57, 105–109 CrossRef CAS;
(g) M. Rimaz, H. Mousavi, M. Behnam and B. Khalili, Curr. Chem. Lett., 2016, 5, 145–154 CrossRef.
-
(a) R. N. Butler and A. C. Coyne, Chem. Rev., 2010, 110, 6302–6337 CrossRef CAS PubMed;
(b) A. Chanda and V. V. Fokin, Chem. Rev., 2009, 109, 725–748 CrossRef CAS PubMed;
(c) M. Rimaz, J. Khalafy, H. Mousavi, S. Bohlooli and B. Khalili, J. Heterocycl. Chem., 2017, 54, 3174–3186 CrossRef CAS;
(d) M. Rimaz, J. Khalafy and H. Mousavi, Res. Chem. Intermed., 2016, 42, 8185–8200 CrossRef CAS;
(e) M. H. Sayahi, S. Bahadorikhalili, S. J. Saghanezhad, M. A. Miller and M. Mahdavi, Res. Chem. Intermed., 2020, 46, 491–507 CrossRef CAS;
(f) H. Mousavi, J. Mol. Struct., 2021, 1251, 131742 CrossRef.
-
(a) M. A. P. Martins, C. P. Frizzo, D. N. Moreira, L. Buriol and P. Machado, Chem. Rev., 2009, 109, 4140–4182 CrossRef CAS PubMed;
(b) M. S. Singh and S. Chowdhury, RSC Adv., 2012, 2, 4547–4592 RSC;
(c) M. Tavakolian, S. Vahdati-Khajeh and S. Asgari, ChemCatChem, 2019, 11, 2943–2977 CrossRef CAS.
-
(a) H. Mousavi, B. Zeynizadeh, R. Younesi and M. Esmati, Aust. J. Chem., 2018, 71, 595–660 CrossRef CAS;
(b) B. Zeynizadeh, R. Younesi and H. Mousavi, Res. Chem. Intermed., 2018, 44, 7331–7352 CrossRef CAS;
(c) B. Zeynizadeh, F. Mohammad Aminzadeh and H. Mousavi, Res. Chem. Intermed., 2019, 45, 3329–3357 CrossRef CAS;
(d) B. Zeynizadeh, H. Mousavi and S. Zarrin, J. Chin. Chem. Soc., 2019, 66, 928–933 CrossRef CAS;
(e) B. Zeynizadeh, F. Mohammad Aminzadeh and H. Mousavi, Green Process. Synth., 2019, 8, 742–755 CAS;
(f) B. Zeynizadeh, F. Sepehraddin and H. Mousavi, Ind. Eng. Chem. Res., 2019, 58, 16379–16388 CrossRef CAS;
(g) R. Bakhshi, B. Zeinizadeh and H. Mousavi, J. Chin. Chem. Soc., 2020, 67, 623–637 CrossRef CAS;
(h) B. Zeynizadeh, H. Mousavi and F. Sepehraddin, Res. Chem. Intermed., 2020, 46, 3361–3382 CrossRef CAS;
(i) B. Zeynizadeh, F. Mohammad Aminzadeh and H. Mousavi, Res. Chem. Intermed., 2021, 47, 3289–3312 CrossRef CAS;
(j) B. Zeynizadeh, H. Mousavi and F. Mohammad Aminzadeh, J. Mol. Struct., 2022, 1253, 131926 CrossRef;
(k) M. Hasanpour Galehban, B. Zeynizadeh and H. Mousavi, RSC Adv., 2022, 12, 11164–11189 RSC.
- S. Nisar, A. S. Pandit, M. Nadeem, A. H. Pandit, M. M. A. Rizvi and S. Rattan, Int. J. Biol. Macromol., 2021, 182, 37–50 CrossRef CAS PubMed.
-
(a) J. Singh, P. K. Dutta, J. Dutta, A. J. Hunt, D. J. Macquarrie and J. H. Clark, Carbohydr. Polym., 2009, 76, 188–195 CrossRef CAS;
(b) J. A. Sirviö, A. M. Kantola, S. Komulainen and S. Filonenko, Biomacromolecules, 2021, 22, 2119–2128 CrossRef PubMed;
(c) M. Esfandiari, A. K. Abbas, M. R. Vakili, H. Shahbazi-Alavi and J. Safaei-Ghomi, Res. Chem. Intermed., 2021, 47, 483–496 CrossRef CAS.
- J. M. Tan, S. Bullo, S. Fakurazi and M. Z. Hussein, Sci. Rep., 2020, 10, 16941 CrossRef CAS PubMed.
-
(a) P. C. Rath, B. P. Singh, L. Bersa and S. Bhattacharjee, J. Am. Chem. Soc., 2012, 95, 2725–2731 CAS;
(b) M. A. Gizawy, H. A. Shamsel-Din, I. M. Abdelmonem, M. I. A. Ibrahim, L. A. Mohamed and E. Metwally, Int. J. Biol. Macromol., 2020, 163, 79–86 CrossRef CAS PubMed;
(c) R. Afshari, S. E. Hooshmand, M. Atharnezhad and A. Shaabani, Polyhedron, 2020, 175, 114238 CrossRef.
-
(a) G. Yang, C. Cheng, G.-B. Xu, L. Tang, K.-L. Chua and Y.-Y. Yang, Bioorg. Med. Chem., 2020, 28, 115606 CrossRef CAS PubMed;
(b) N. S. O'Brein and A. McCluskey, Aust. J. Chem., 2020, 73, 1176–1186 CrossRef;
(c) G. Yashwantro, V. P. Jejukar, R. Kshatriya and S. Saha, ACS Sustainable Chem. Eng., 2019, 7, 13551–13558 CrossRef;
(d) Z. Lin, J. Qian, P. Lu and Y. Wang, J. Org. Chem., 2020, 85, 11766–11777 CrossRef CAS PubMed;
(e) S. Balaji, G. Balamurugan, R. Ramesh and D. Semeril, Organometallics, 2021, 40, 725–734 CrossRef CAS.
- B. Akhlaghinia, P. Sanati, A. Mohammadinezhad and Z. Zarei, Res. Chem. Intermed., 2019, 45, 3215–3235 CrossRef CAS.
- C. Patil, S. K. Shinde, U. P. Patil, A. T. Birajdar and S. S. Patil, Res. Chem. Intermed., 2021, 47, 1675–1691 CrossRef.
- S. Rahmani and B. Zeynizadeh, Res. Chem. Intermed., 2019, 45, 1227–1248 CrossRef CAS.
- D. K. Agarwal, A. Sethiya, P. Teli, A. Manhas, J. Sani, N. Sahiba, P. C. Jha, S. Agarwal and P. K. Goyal, J. Heterocycl. Chem., 2020, 57, 3294–3309 CAS.
- F. Shirini, A. Fallah-Shojaei, L. Samavi and M. A. Abedini, RSC Adv., 2016, 6, 48469–48478 RSC.
- M. Seddighi, F. Shirini and M. Mamaghani, RSC Adv., 2013, 3, 24046–24053 RSC.
- X. Wu and W.-X. Peng, J. Chin. Chem. Soc., 2020, 67, 2129–2148 CrossRef CAS.
- H. Mehrabi and H. Abusaidi, J. Iran. Chem. Soc., 2010, 7, 890–894 CrossRef CAS.
- N. O. Mahmoodi, Z. Jalalifard and G. Pirbasti Fathanbari, J. Chin. Chem. Soc., 2020, 67, 172–182 CrossRef CAS.
- R. Teimuri-Mofrad, S. Tahmasebi and E. Payami, Appl. Organomet. Chem., 2019, 33, e4773 CrossRef.
- S. Mathavan, K. Kannan and R. B. R. D. Yamajala, Org. Biomol. Chem., 2019, 17, 9620–9626 RSC.
- E. El hajri, Z. Benzekri, S. Sibous, A. Ouasri, S. Boukhris, A. Hassikou, A. Rhandour and A. Souizi, J. Mol. Struct., 2021, 1230, 129890 CrossRef.
- L. H. T. Nguyen, T. T. T. Nguyen, Y. T. Dang, P. H. Tran and T. L. H. Doan, Asian J. Org. Chem., 2019, 8, 2276–2281 CrossRef CAS.
- M. Nikoorazm and M. Khanmoradi, J. Chin. Chem. Soc., 2020, 67, 1477–1489 CrossRef CAS.
- H. Heidari, K. Nikoofar and Y. Shahedi, Iran. J. Catal., 2019, 9, 71–77 CAS.
- H. Ghafuri, N. Goodarzi, A. Rashidzadeh and M. A. Douzandegi Fard, Res. Chem. Intermed., 2019, 45, 5027–5043 CrossRef CAS.
- G. Kumar, N. K. Mogha and D. T. Masram, ACS Appl. Nano Mater., 2021, 4, 2682–2693 CrossRef CAS.
- A. Ghorbani-Choghamarani, H. Aghavandi and M. Mohammadi, J. Porous Mater., 2021, 28, 1167–1186 CrossRef CAS.
- T. Tamoradi, S. M. Mousavi and M. Mohammadi, New J. Chem., 2020, 44, 3012–3020 RSC.
- G. C. Pariyar, B. Mitra, S. Mukherjee and P. Ghosh, ChemistrySelect, 2020, 5, 104–108 CrossRef.
- B. B. F. Mirjalili, Z. Zaghaghi and A. Monfared, J. Chin. Chem. Soc., 2020, 67, 197–201 CrossRef CAS.
- L. Heidari and L. Shiri, Appl. Organomet. Chem., 2019, 33, e4636 CrossRef.
- L. Shiri, A. Ghorbani-Choghamarani and M. Kazemi, Appl. Organomet.
Chem., 2019, 33, e4636 CrossRef.
- F. Ghoreyshi Kahangi, M. Mehrdad, M. M. Heravi and S. Sadjadi, Sci. Rep., 2020, 10, 15285 CrossRef PubMed.
- S. Sadjadi, F. Ghoreyshi Kahangi, M. Dorraj and M. M. Heravi, Molecules, 2020, 25, 241 CrossRef CAS PubMed.
- S. Karhale, Res. Chem. Intermed., 2020, 46, 3085–3096 CrossRef CAS.
- S. E. Sadati Sorkhi, M. M. Hashemi and A. Ezabadi, Res. Chem. Intermed., 2020, 46, 2229–2246 CrossRef CAS.
- M. Salami and A. Ezabadi, Res. Chem. Intermed., 2020, 46, 4611–4626 CrossRef CAS.
- S. Askari, M. M. Khodaei and M. Jafarzadeh, Res. Chem. Intermed., 2021, 47, 2881–2899 CrossRef CAS.
- H. Foroughi Niya, N. Hazeri, M. Fatahpour and M. T. Maghsoodlou, Res. Chem. Intermed., 2020, 46, 3651–3666 CrossRef.
- G. Rathee, S. Kohli, N. Singh, A. Awasthi and R. Chandra, ACS Omega, 2020, 5, 15673–15680 CrossRef CAS PubMed.
- S. F. Hojati, M. Moosavifar and N. Moeinieghbali, J. Chem. Sci., 2020, 132, 38 CrossRef CAS.
- P. G. Kargar, G. Bagherzadeh and H. Eshghi, RSC Adv., 2021, 11, 4339–4355 RSC.
- Subodh, N. K. Mogha, K. Chaudhary, G. Kumar and D. T. Masram, ACS Omega, 2018, 3, 16377–16385 CrossRef CAS PubMed.
- R. Pourhasan-Kisomi, F. Shirini and M. Golshekan, Appl. Organomet. Chem., 2018, 32, e4371 CrossRef.
- M. Ziyaadini, N. Nemat-Bakhsh, S. J. Roudbaraki and M. Ghashang, Polycyclic Aromat. Compd., 2022, 42, 460–474 CrossRef CAS.
- M. M. Khodaei, A. Alizadeh and M. Haghipour, Res. Chem. Intermed., 2020, 46, 1033–1045 CrossRef CAS.
- S. Abdolahi, M. Hajjami and F. Gholamian, Res. Chem. Intermed., 2021, 47, 1883–1904 CrossRef CAS.
- P. Singh, P. Yadav, A. Mishra and S. K. Awasthi, ACS Omega, 2020, 5, 4223–4232 CrossRef CAS PubMed.
- S. Amirnejat, A. Nosrati, R. Peymanfar and S. Javanshir, Res. Chem. Intermed., 2020, 46, 3683–3701 CrossRef CAS.
- H. Foroughi Niya, N. Hazeri, M. Rezaie Kahkhaie and M. T. Maghsoodlou, Res. Chem. Intermed., 2020, 46, 1685–1704 CrossRef.
- F. Hasaanzadeh, N. Daneshvar, F. Shirini and M. Mamaghani, Res. Chem. Intermed., 2020, 46, 4971–4984 CrossRef.
- A. Mahajan and M. Gupta, Appl. Organomet. Chem., 2021, 35, e6161 CrossRef CAS.
- P. Mohammadi and H. Sheibani, Mater. Chem. Phys., 2019, 228, 140–146 CrossRef CAS.
- M. Khaleghi Abbasabadi, D. Azarifar and H. R. Esmaili Zand, Appl. Organomet. Chem., 2020, 34, e6004 CAS.
|
This journal is © The Royal Society of Chemistry 2022 |