DOI:
10.1039/D1QO01395E
(Review Article)
Org. Chem. Front., 2022,
9, 499-516
The applications of catalytic asymmetric halocyclization in natural product synthesis
Received
16th September 2021
, Accepted 17th November 2021
First published on 18th November 2021
Abstract
Halocyclization of olefinic substrate enables the establishment of cyclic skeletons via intramolecular halonium-induced nucleophilic addition, which has been well utilized as a practical strategy for constructing cyclic skeletons in natural product synthesis. Recently, the renaissance and rapid evolution of organocatalysis have accelerated the development of catalytic asymmetric halocyclization. In this context, natural product synthesis powered by catalytic asymmetric halocyclization has also achieved considerable progress in recent years. In some cases, these newly developed protocols enable more concise synthetic routes for accessing enantioenriched natural products. To this end, this review summarizes the applications of catalytic asymmetric halocyclization in natural product synthesis.
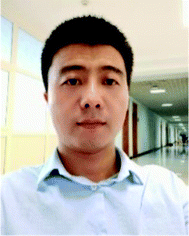 Jiahang Yan | Jiahang Yan was born in 1989 in Tianshui, Gansu Province, China. He received his M.S. in Chemistry from Northwest A&F University in 2018. Currently, he is pursuing his Ph.D. degree under the supervision of Prof. Weiqing Xie. His research interests focus on the asymmetric synthesis of indole alkaloids via asymmetric catalysis. |
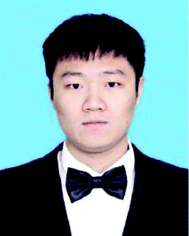 Zhiqiang Zhou | Zhiqiang Zhou was born in Shandong province. After receiving his B.S. degree in 2019 at Northwest A&F University, he has been a master's student under the guidance of Prof. Weiqing Xie. He is currently working on the development of catalytic asymmetric methodology towards the asymmetric synthesis of diterpenoids. |
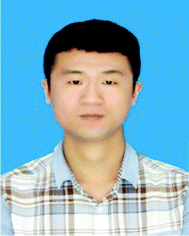 Qiaoqiao He | Qiaoqiao He was born in Gansu Province. In 2019, he began to pursue his M.S. degree under the guidance of Prof. Weiqing Xie. He is currently concentrating on the asymmetric synthesis of aspidospmerma indole alkaloids. |
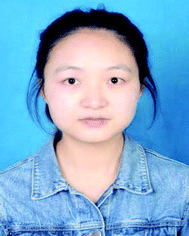 Guzhou Chen | Guzhou Chen gained her B.S. degree in applied chemistry in 2014 and M.S. degree in organic chemistry in 2017 from Shenyang Pharmaceutical University. In 2021, she obtained her Ph.D. degree in chemical biology from Northwest A&F University under the supervision of Prof. Weiqing Xie. Her current research interests included developing asymmetric methodologies and applications in the synthesis of polymeric cyclotryptamine alkaloids. |
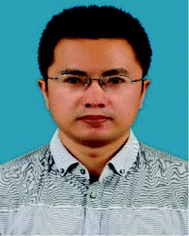 Hongbo Wei | Hongbo Wei received his B.S. degree in 2010 and M.S. degree in 2013 from Lanzhou University. He then earned his Ph.D. degree under the supervision of Professor Hongbin Zhai at the same university. In 2016, he moved to the College of Chemistry & Pharmacy of Northwest A&F University and worked as a lecturer in Prof. Xie's group. His current research interests include developing asymmetry synthetic methodologies and the total synthesis of biologically active natural products. |
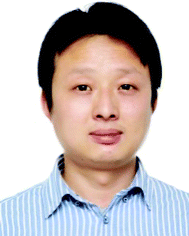 Weiqing Xie | Weiqing Xie pursued his Ph.D. degree at Shanghai Institute of Organic Chemistry (SIOC) under the supervision of Prof. Dawei Ma after obtaining his BSc degree in chemistry from Lanzhou University in 2002. In 2007, he was appointed as assistant Professor at SIOC upon receiving his Ph.D. degree. From 2009 to 2011, he worked as postdoc in John R. Falck's group at UT Southwestern Medical Center at Dallas. Then he returned to SIOC and began his academic career as associate professor in Prof. Ma's group. In 2015, he moved to Northwest A&F University and set up his research group. His research interests include the total synthesis of complex natural products and target-oriented synthetic methodologies. |
The halonium-induced functionalization of the C–C double bond is one of the fundamental transformations in organic synthesis,1 as it enables the difunctionalization of alkenes and also provides a versatile handle (the halogen) for further manipulation. In this regard, halocyclization, dihalogenation and other halo-functionalizations have found wide applications in the total synthesis of natural products.2 Mechanistically, the reaction is initiated by the addition of halonium to the C–C double bond to form a cationic cyclic halonium–alkene complex 1 (Fig. 1).3 However, little information was gained about this conceptual bridged halonium until Olah and co-workers observed this species in SbF5–SO2 solution using NMR spectroscopy.4a,b In 1969, the bromonium of adamantylideneadamantane 2 was synthesized and isolated by Wynberg4c and subsequently its structure was unambiguously established by X-ray crystallographic analysis.4d For the halonium-induced reactions, the following capture of this intermediate usually results in high diastereoselectivities, which originate from the anti-addition of nucleophiles to the bridged halonium. However, this intermediate is not always involved in all the halonium-induced transformations of the olefin. Kinetic and mechanistic studies also support the existence of the β-halo-carbenium ion in some cases, which depends on the substituents on the olefin, additives, and the reaction solvent.5 However, the stepwise pathway via cationic intermediates is not always followed. In this regard, concerted nucleophile-assisted alkene activation (NAAA) has been proposed for syn-addition in the (DHQD)2PHAL-catalyzed asymmetric halolactonization of alkenoic acid.6
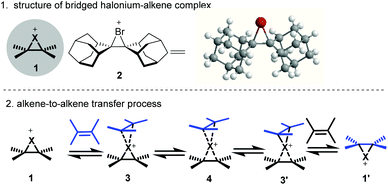 |
| Fig. 1 The cyclic halonium–alkene complex and alkene-to-alkene transfer process. | |
Although the halogenation of an alkene usually leads to excellent diastereoselectivities via the anti-addition of nucleophiles to the cyclic halonium intermediate, enantioselective halogenation remained elusive long after the discovery of halogenation reactions.1 Brown and co-workers observed that the labile bridged bromonium–alkene complex 2 underwent rapid alkene-to-alkene transfer in the presence of an alkene acceptor, presumably via the associated complexes 3, 3′ and 4 (Fig. 1).7 In this regard, the alkene acceptor could be considered the ancillary ligand to the bromonium. Later, Denmark and co-workers suggested that this process could lead to the epimerization of the enantioenriched halonium intermediate.8 The alkene-to-alkene process accounts for the low enantioselectivities when the reactions were performed using a chiral halogenating reagent.9 Therefore, it is not surprising that the catalytic asymmetric halonium-induced functionalization of the C–C double bond was not developed until 1995.10
During the past two decades, asymmetric organocatalysis has evolved into a general tool for asymmetric synthesis,11 which is powering the development of catalytic asymmetric halogenation reactions.12–14 In this context, the catalytic asymmetric chlorination of an aldehyde promoted by prolinol derivatives for accessing enantioenriched α-chloro-aldehyde was concurrently reported by Jørgensen and MacMillan.12 The following applications of this method in natural product synthesis have been well practised and reviewed.13 Besides this type of reaction, the catalytic asymmetric dihalogenation of olefins has also emerged, promoted by organocatalysts since Nicolaou's first report.14b However, these protocols have not been employed in natural product synthesis owing to the limitations of substrate scope. More recently, the catalytic asymmetric dibromination, bromochlorination, and dichlorination of allylic alcohol with aliphatic substituents have been reported by Burns and co-workers by taking advantage of the chiral Ti(IV) complex as catalyst.15a–c A host of structurally diverse halogenated natural products have been synthesized relying on these newly developed protocols, which have been summarized in Burns’ recent account.15d
On the other hand, halocyclization enables the establishment of cyclic skeletons via intramolecular halonium-induced nucleophilic addition.1d–i,k,l A variety of N- and O-heterocycles as well as carbocycles could be easily accessed from olefinic amines, alcohols, and carboxylic acids. In this regard, halocyclization has also been well capitalized for the construction of the ring systems of natural products.1d,g,2b During the last decades, catalytic asymmetric halocyclization has rapidly evolved, providing a facile entry to enantioenriched heterocycles and carbocycles.16 Based on these exciting advances, the strategic applications of catalytic asymmetric halocyclization in natural products have been nicely demonstrated. In this regard, the enantioenriched halogenated cyclic building blocks could be further transformed into advanced synthetic intermediates for natural product synthesis, relying on the versatile transformations of halogen. Additionally, catalytic asymmetric halocyclization could sometimes be directly applied in the synthesis of natural products with a stereogenic halogenated carbon center. In this review, we summarize recent progress in natural product synthesis employing catalytic asymmetric halocyclizations. To this end, the contents are categorized by the type of catalytic asymmetric halogenation.
1. Catalytic asymmetric haloaminocyclization in natural product synthesis
N-Heterocycles (e.g. pyrrolidine, piperidine) are the privileged fragments of many natural products and pharmaceuticals.17 Enabling facile entry to N-heterocycles from unsaturated amines, haloaminocyclization has been well applied in the total synthesis of alkaloids.18 In 2011, Yeung and colleagues reported the first catalytic asymmetric 5-exo bromoaminocyclization of unsaturated sulfonamide using a chiral amino-thiocarbamate catalyst.19a Subsequently, bifunctional organocatalysts, chiral Lewis bases, and chiral phosphoric acids have been developed for realizing catalytic asymmetric 5-exo and 5-endo haloaminocyclization (Fig. 2). However, most of the resulting chiral pyrrolidines are not suitable for further elaboration to natural products due to the substrate restriction. Surprisingly, the catalytic asymmetric 6-exo or 6-endo haloaminocyclization of olefinic amines remain unreported to date, despite the enantioselective haloaminocyclization of the corresponding olefinic hydrozone and N-tosylcarbamate having been documented.20
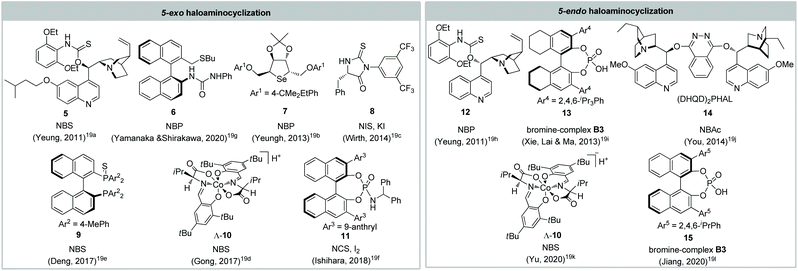 |
| Fig. 2 Reported catalysts and halogenating reagents employed for the catalytic asymmetric haloaminocyclization of olefinic amines. | |
Hexahydropyrrolo[2,3-b]indole (HPI) is a featured scaffold incorporated in a handful of indole alkaloids, which are also known as cyclotryptamine alkaloids (Fig. 3).21 The cyclotryptamine alkaloids could be categorized into two classes: monoterpenoid indole alkaloids (e.g. conolutinine, minfiensine and vincorine) with one HPI unit and polymeric cyclotryptamine alkaloids with two or more HPIs. Interestingly, the HPIs of polymeric cyclotryptamines are connected by one C3a–C3a′ bond and one or more C3a–C7′ bonds. It is noteworthy that the C3a–C3a′ linkage constitutes a contiguous quaternary carbon centers (CQCCs) skeleton, which could be a C2- (e.g. (−)-chimonanthine) or a meso-symmetric (e.g. hodgkinsines) configuration. In this context, the formidable structural features and interesting biological activities of cyclotryptamine alkaloids have attracted a lot of attention from the synthetic community.21
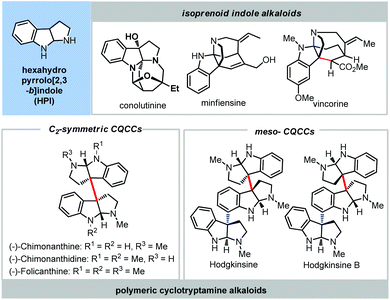 |
| Fig. 3 Monoterpenoid indole alkaloids with one HPI unit and representative polymeric cyclotryptamine indole alkaloids. | |
The enantioselective construction of the HPI ring has witnessed tremendous advances via the asymmetric catalytic dearomatization (CADA) of tryptamine or tryptaphon.22 In this regard, a unified strategy could be envisioned by using an enantioenriched C3a-bromo-HPI as a building block prepared from the catalytic asymmetric synthesis of cyclotryptamine alkaloids as bromine could be a versatile handle for subsequent cyclization and homo- or hetero-dimerization (vide infra). However, the catalytic asymmetric bromocyclization of tryptamine has posed significant challenges due to the fast uncatalyzed background reaction. To this end, Xie, Lai and Ma realized the highly enantioselective bromocyclization of tryptamine by employing 8H-R-TRIP using it as an anionic phase transfer catalyst and B3 as a bromine source in 2013 (Scheme 1).19i This novel type of brominating reagent B3 is a bench-stable yellowish solid and could be prepared on a decagram scale from easily available DABCO-derived ammonium salt and bromine. Various carbamate or sulfamide protecting groups and electron-withdrawing and donating substituents, even on the C4 or C7 of indole, were accommodated, giving C3a-bromo-HPIs in good to excellent enantioselectivities (Scheme 1). It is noteworthy that C2-alkylated tryptamines were also smoothly cyclized to C3a-bromo-HPIs with continuous chiral tetra-substituted carbon centers in good enantioselectivities (Scheme 1, 17g and 17h).
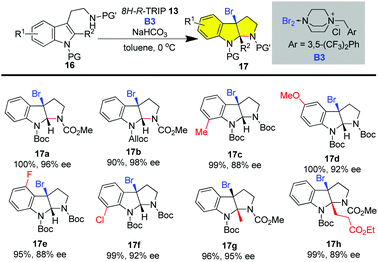 |
| Scheme 1 Catalytic asymmetric bromocyclization of tryptamine (Xie, Lai and Ma). | |
The enantioselective bromoaminocyclization of tryptamine could also be realized by using other types of catalyst system (Fig. 4).19j,k In 2014, You and co-workers reported that the combination of hydroquinine 1,4-phthalazinediyl diether ((DHQ)2PHAL) with (+)-camphorsulfonic acid (CSA) enabled the asymmetric bromocyclization of protected tryptamine in up to 74% ee using N-bromoacetamide (NBAc) as a brominating reagent.19j In 2018, Yu reported that chiral Co(III)-complex-templated Brønsted acids catalyzed the enantioselective bromoaminocyclization of tryptamines by employing NBS as a bromine source in up to 87% ee.19k
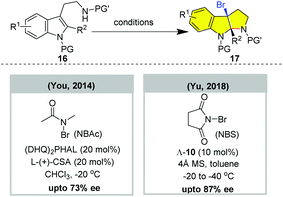 |
| Fig. 4 Other catalyst systems for the enantioselective bromocyclization of tryptamine. | |
As the representative dimeric cyclotryptamine alkaloid, the asymmetric synthesis of (−)-chimonanthine has been extensively described.21e–h,23 However, the previously reported route took longer steps either on the establishment of the CQCCs or on building up the HPIs. As shown in Scheme 2, the C3a-bromo-HPI 3 could be facilely prepared at gram scale by taking advantage of the enantioselective bromocyclization of tryptamine with comparable enantioselectivity. Reductive dimerization23a of C3a-bromo-HPI 16a mediated by Co(PPh3)Cl followed by removal of Boc and reduction of the methylcarbamates furnished (−)-chimonanthine in four steps.
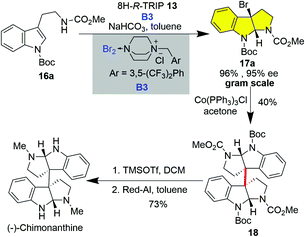 |
| Scheme 2 Four-step synthesis of (−)-chimonanthine (Xie, Lai and Ma). | |
The enantioselective bromocyclization of tryptamine also provides a new strategy for the synthesis of monoterpenoid indole alkaloids. Conolutinine, isolated from Malaysian Tabernaemontana, is a rearranged monoterpenoid indole alkaloid with a featured HPI ring, bridged diaza[4.2.2]decane ring system.24a In 2015, Xie and Lai accomplished the asymmetric synthesis of conolutinine based on the catalytic asymmetric bromocyclization of tryptamine (Scheme 3).24b The synthesis commenced with the three-step preparation of tetrahydropyrido[1,2-a]indole 20 from methylcarbamate-protected tryptamine. The 8H-S-TRIP 13 catalyzed bromocyclization of 20 smoothly gave C3a-bromo-HPI 21 in 95% yield and 91% ee on a gram scale. Subsequently, the installation of the diaza[4.2.2]decane skeleton was realized via sequential N-alkylation and intramolecular cyclization in four steps. Eventually, Mukaiyama hydration of the double bond followed by reduction of the amide bond mediated by DIBAL-H accomplished the synthesis of conolutinine in ten longest linear steps (LLS).
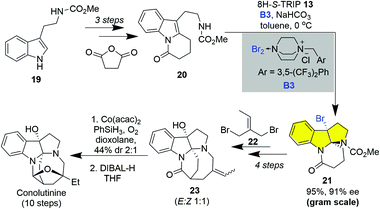 |
| Scheme 3 Ten-step asymmetric synthesis of conolutinine (Xie and Lai). | |
Higher-order polymeric cyclotryptamine alkaloids containing more than two HPIs (e.g. hodgkinsines) have posed significant challenges in the stereoselective construction of C3a–C3a′ and multiple C3a–C7′ bonds. To date, only five groups have accomplished the total synthesis of higher-order polymeric cyclotryptamine alkaloids.25 In this respect, the enantioenriched C3a-bromo-HPI could serve as an ideal building block for the modular synthesis of polymeric cyclotryptamine alkaloids. In 2017, Movassaghi and co-workers reported the collective synthesis of trimeric and tetrameric cyclotryptamine based on a stereospecific diazene-directed fragment assembly strategy.25g As delineated in Scheme 4, the enantioenriched C3a-bromo-HPI 24 was elaborated to C3a-amino-HPI 25 and C7-hydrazinyl-HPI 26 in two and three steps, respectively. Subsequently, AgOTf-mediated aminolysis of C3a-bromo-HPI 24 with C7-hydrazinyl-HPI 26 set up the first diazene linker between C3a and C7′. Then Rh(esp)2-catalyzed benzyl C–H amination of 27, coupling of the resulting sulfonamide with C3a-amino-HPI ent-25 followed by DCDHM-mediated oxidative desulfonylation built up the second diazene linker between C3a and C3a′. To this end, irradiation of a thin film of 30 under 380 nm light selectively activated the labile diazene between C3a and C3a′, stereospecifically forging the contiguous quaternary carbon centers. Further irradiation by 300 nm light forged the C3a–C7 bond via the second extrusion of nitrogen. Finally, hodgkinsine was obtained in 11 LLS via deprotection of 2-(trimethylsilyl)ethyl carbamates (Teoc) and overall reduction of the methylcarbamates of trimer 31. By following the same procedure, assembly of C3a-bromo-HPI 24 with the enantiomeric 26, then coupling with the antipode of C3a-amino-HPI 25 delivered diazene-tethered trimer 33, which was transformed to hodgkinsine B in four steps.
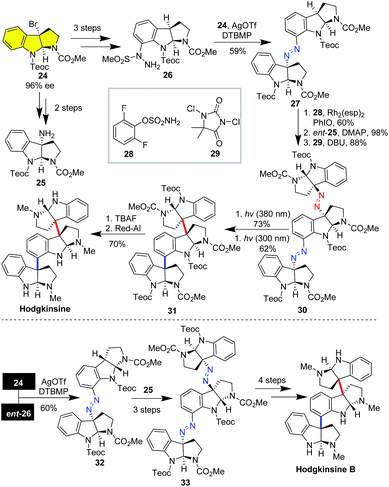 |
| Scheme 4 Modular synthesis of hodgkinsine and hodgkinsine B (Movassaghi). | |
The diazene assembly approach was also amenable to the modular synthesis of tetrameric cyclotryptamine alkaloids.25g As depicted in Scheme 5, the introduction of the benzylic amino to dimer 27via C–H amination and hydrolysis of the resulting sulfamide gave the amino-substituted dimer 34. On the other hand, Rh(esp)2-catalyzed C–H benzyl amination of 32 led to sulfamate ester 35, which was coupled with amine 34 followed by DCDHM-mediated oxidative desulfonylation to deliver the triazene-tethered tetramer 36. Selective photolysis of 36 under 380 nm ultraviolet light and subsequent irradiation with 300 nm ultraviolet light produced tetramer 37 in 32% overall yield. Notably, direct photolysis of 36 with 300 nm ultraviolet light could directly generate tetramer 20 in 22% yield with four quaternary carbon centers being stereospecifically set up in one operation. The eventual removal of Teoc and the overall reduction of methyl carbamates with allane afforded (−)-quadrigemine in 54% yield in two steps. Further treatment of (−)-quadrigemine C with aqueous acetic acid led to (−)-psycholeine in 36% yield.
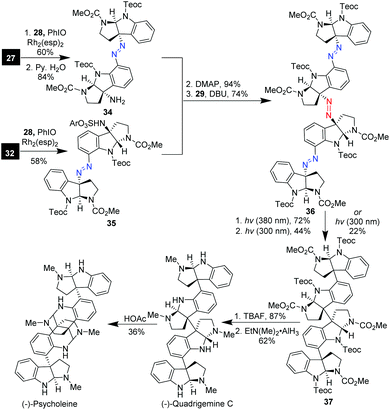 |
| Scheme 5 Asymmetric synthesis of tetrameric cyclotryptamine (−)-quadrigemine C and (−)-psycholeine (Movassaghi). | |
Communesins are a family of dimeric cyclotryptamines with a rearranged skeleton, containing a fused heptacycle, two aminal linkages, and up to six stereogenic centers, of which two are vicinal and quaternary (C3a–C3a′).26 The communesins possess interesting biological profiles, including potent cytotoxicity, and insecticidal, antiproliferative, and vasculogenetic activities. The challenging structure of communesins has spurred widespread synthetic interest and 12 total syntheses have been concluded to date.27 In 2019, Movassaghi and co-workers achieved the collective synthesis of epoxy-communesins by utilizing the diazene-directed fragment coupling strategy for stereospecifically forging the C3a–C3a′ bond.27g As shown in Scheme 6, the synthesis commenced with the asymmetric synthesis of the (−)-aurantioclavine derivative 39 from enantioenriched sulfinamide (−)-38 in 9 steps on a gram scale. On the other hand, treatment of C3a-bromo-HPI 40, prepared from the enantioselective bromocyclization of a protected tryptamine, with AgOTf in the presence of 2,6-di-tert-butyl-4-methylpyridine (DTBMP) afforded the sulfamate ester (+)-41. Union of the functionalized aurantioclavine fragment 39 with the HPI fragment 41via sulfamidation, partial reduction of the oxindole entity with LiBH4 followed by capture of the hemiaminal with TMSCN afforded aminonitrile 42 as a single diastereomer. Selective oxidation of the sulfuric diamide moiety with N-chloro-N-methylbenzamide in the presence of polystyrene-bound diazaphosphorine (BEMP) generated a sensitive diazene, which was subjected to photo-irradiation to yield heterodimer 44 in 50% yield as a single diastereoisomer via the extrusion of nitrogen. Hydrogenolysis of Cbz followed by t-BuOLi-mediated skeletal rearrangement enabled the stereoselective building up of B/E rings of the communesins. In this regard, the ring opening of the HPI moiety of 45 under basic conditions gave a sulfonamide anion, which attacked the iminium generated from the aminonitrile via elimination of cyanide to form the E ring. Meanwhile, the capture of cyclic imine by N1 enabled concurrent B-ring closure. Eventual derivatization of pentacycle 20 in one to four steps thus furnished the collective asymmetric synthesis of communesins.
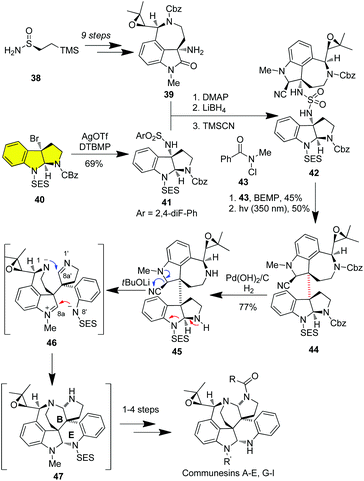 |
| Scheme 6 Collective asymmetric synthesis of communesins (Movassaghi). | |
2. Catalytic asymmetric halo-cycloetherification in natural product synthesis
Saturated cyclic ethers (e.g. epoxide, tetrahydrofuran and tetrahydro-2H-pyran) are abundantly present in a large number of natural products and pharmaceutically active molecules.28 In this context, a number of methodologies have been documented for building up cyclic ether skeletons.29 In this regard, the halo-cycloetherification of olefinic alcohols has been widely utilized in natural product synthesis2,30 as olefinic alcohols are easily prepared and usually high diastereoselectivities could be obtained via the anti-addition of oxygen to the cyclic halonium intermediate. However, the catalytic asymmetric halo-cycloetherification achieved little progress until the first catalytic asymmetric halo-cycloetherification was reported by Kang in 2003, which employed salen-Co(II) as a catalyst and I2 as a halogen source.31a Since 2011, various organocatalysts including chiral phosphoric acid, bifunctional organocatalysts and chiral metallic catalysts have been developed for catalytic asymmetric halo-cycloetherification (Fig. 5). To this end, various types of asymmetric halo-cycloetherification (e.g. 5-exo, 5-endo, 6-exo, 6-endo cyclization) have been realized, enabling the construction of enantioenriched halogenated tetrahydrofuran and tetrahydro-2H-pyran scaffolds.31
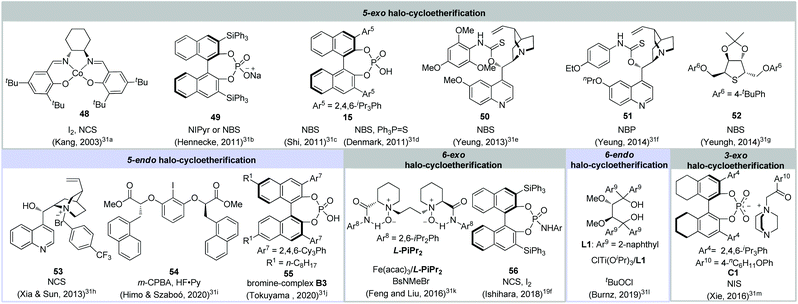 |
| Fig. 5 Developed catalysts and halogenating reagents employed for the catalytic asymmetric halo-cycloetherification. | |
In 2016, Xie and colleagues designed an ion-pair organocatalyst C1 consisting of chiral phosphoric acid and DABCO-derived quaternary ammonium, which realized the challenging catalytic asymmetric 3-exo iodo-cycloetherification of allylic alcohol 57 to afford chiral epoxide 58 with up to 98% ee (Scheme 7).31m Moreover, this protocol was amenable to the one-pot catalytic 3-exo cycloetherification/Wagner–Meerwein rearrangement promoted by BF3·OEt for producing enantioenriched cyclohexanone 60 with an aryl quaternary carbon center.
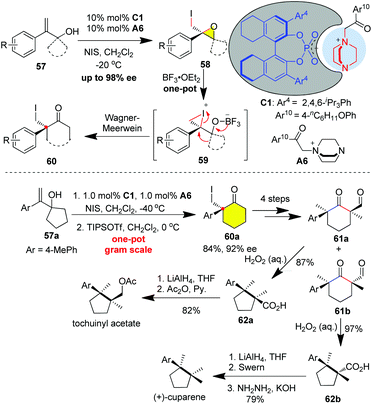 |
| Scheme 7 Total synthesis of cuparene sesquiterpenoids based on catalytic asymmetric 3-exo iodo-cycloetherification (Xie). | |
Cuparene-type sesquiterpenoids feature a cyclopentane skeleton incorporating contiguous quaternary carbon centers (CQCCs), one of which is aryl-substituted.32 The stereoselective construction of the CQCCs constitutes the major challenge in the synthesis of cuparene sesquiterpenoids.33 In this context, Xie and colleagues discovered H2O2-mediated ring contraction of α-formyl cycloketone for the stereospecific construction of CQCCs.34 By strategically combining these two novel protocols, the same group accomplished the asymmetric synthesis of cuparene-type sesquiterpenoids. As drawn in Scheme 7, the gram-scale synthesis of cyclohexanone 60a with an aryl quaternary carbon center was conveniently achieved in 85% yield with 92% ee on a gram scale only in the presence of 1.0 mol% ion-pair organocatalyst C1 (Scheme 7). Subsequent transformations of cyclohexanone 60avia the removal of iodine, formylation followed by methylation delivered the two separable diastereoisomers 61a and 61b, which were respectively subjected to H2O2-mediated oxidative ring construction to stereospecifically forge the CQCCs. Reduction of carboxylic acid 62a and acetylation of the resulting primary alcohol furnished tochuinyl acetate in 8 LLS. The other diastereoisomer 62b could be converted to cuparene in 9 LLS through sequential reduction/oxidation/Huang–Kishner reduction.
More frequently, catalytic asymmetric halo-cycloetherification has been applied in the asymmetric synthesis of natural products with a chiral O-heterocycle skeleton. (−)-Centrolobine belongs to diarylheptanoids isolated from the heartwood of Centrolobium robustum and the stem of Brosimum potabile with a cis-disubstituted tetrahydro-2H-pyran ring, which exhibits anti-inflammatory, antibacterial, and antileishmanial activities (Scheme 8).35 In 2016, Feng and Liu disclosed a catalytic asymmetric intra- and intermolecular haloetherification of enone 63 promoted by chiral N,N′-dioxides/Fe(acac)3.31k The authors disclosed that the kinetic resolution of alcoholic enone 65 could be readily realized by chiral N,N′-dioxides/Fe(acac)3 catalyzed bromo-cycloetherification to deliver cis-substituted pyran 66, which underwent debromination under the action of BnSH/Et3N to give ketone 67 in 50% yield over two steps and 95% ee with >99
:
1 dr. Reduction of the ketone 67 with LiBH4 followed by the removal of the resulting secondary alcohol mediated by Et3SiH/TFA, Ullman coupling of aryl bromide with NaOMe and removal of benzyl by hydrogenation over Pd/C finally furnished (−)-centrolobine in 6 LLS.
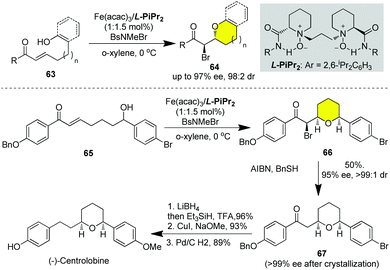 |
| Scheme 8 Asymmetric synthesis of (−)-centrolobine via catalytic asymmetric intramolecular 6-exo bromo-etherification of enone (Feng and Liu). | |
(−)-Deoxoapodine belongs to the Aspidosperma indole alkaloids incorporating a characteristic tetrahydrofuran ring.36 In 2020, Tokuyama and co-worker accomplished a 10-step synthesis of (−)-deoxoapodine relying on chiral counter ionic directed 5-endo cycloetherification for forging the chiral tetrahydrofuran ring. As shown in Scheme 9, the catalytic asymmetric 5-endo bromocyclization of homoallylic alcohol 68 promoted by chiral phosphoric acid CPA1 using bromine complex B3 as the brominating reagent was readily realized in 76% yield with 86% ee on a two-gram scale.31j Allylation of bromo-furan 70 under the effect of AIBN/allylstannane successively installed the quaternary carbon centers. Subsequent cleavage of Cbz, introduction of indole-3-acetyl and transformation of the terminal alcohol to iodide gave iodide 71. After a series of trials, the highly strained bridged nine-membered was successively established by PdI2/norbornene catalyzed intramolecular C–H alkylation, which furnished petacycle 72 in 67% yield. Reduction of the amide moiety of 72, oxidative cyclization catalyzed by Fe(S,S-PDP) for C-ring closure and the introduction of the methoxycarbonyl group delivered a sub-gram quantity (260 mg) of (−)-deoxoapodine in 10 LLS.
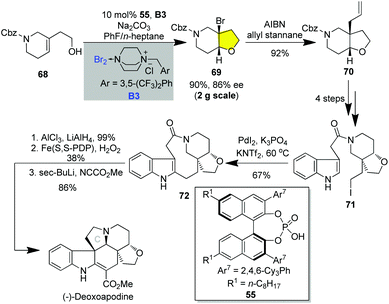 |
| Scheme 9 Asymmetric synthesis of (−)-deoxoapodine relying on catalytic asymmetric 5-endo bromo-cycloetherification (Tokuyama). | |
Halogenated natural products are widely found in nature.37 The introduction of halogen may alter the physical properties, including electronic and steric effects, which can be of importance for increasing biological affinity and selectivity. In this regard, the synthesis of natural products with chiral halogenated carbon centers has attracted a lot of attention and a plethora of strategies for setting up the chiral halogenated carbon centers has been invented.2b Azamerone is structurally unique among the napyradiomycin natural products containing two enantioenriched chlorinated tetrahydro-2H-pyran rings and an unprecedented phthalazinone ring.38 Recently, Burns and co-workers accomplished the first enantioselective synthesis of azamerone by taking advantage of asymmetric 6-endo chloro-cycloetherification of prenylated hydroxyquinone 73 promoted by a TADDOL ligated Ti(IV) using tert-butyl hypochlorite as a chloronium source (Scheme 10).31l In this context, the initial formation of octahedral titanium complex I was proposed for the stereoselective transfer of chloronium to the prenyl side chain, which undergoes a stereoselective 6-endo cyclization to afford o-quinone 74. Conversion of o-quinone to quinone diazide 75 was then achieved in three steps. On the other hand, chlorocyclohexane 76 was prepared from geranyl acetate via mercury-based polyene cyclization and resolution in six steps. The coupling of quinone diazide 75 with boronic hemiester 76 catalyzed by (SPhos)Pd-G3 successively provided phenol 77 in 46% yield. Dechlorination, protection of the tertiary alcohol with TBS and oxidation of phenol diastereoselectively gave quinone 78. The installation of the phthalazinone moiety was realized though [4 + 2]/retro[4 + 2] cycloaddition of 78 with tetrazole A promoted by bisboron complex B, which was followed by in situ air oxidative rearomatization and benzyl alcohol oxidation. Eventual cleavage of TBS mediated by HCl furnished azamerone in 10 LLS.
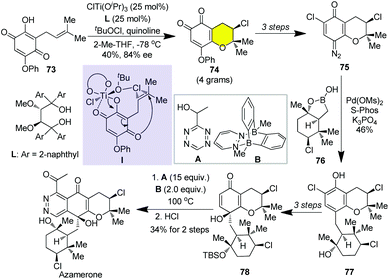 |
| Scheme 10 Enantioselective synthesis of azamerone (Burns). | |
3. Catalytic asymmetric halolactonization in natural product synthesis
The iodolactonization of alkenoic acid was first discovered by Bougoult at the turn of 20th century.1d,h Since then, halolactonization has received continuous attention from the synthetic community and has become a very useful and reliable strategy in natural product synthesis.1,2 However, the catalytic asymmetric halolactonization of olefinic acid remained underdeveloped until Gao reported the first asymmetric 5-exo iodolactonization by using cinchonine derived PTC 79 as a catalyst albeit in low enantioselectivities (Fig. 6).39a Soon after, the same group further demonstrated that salen-Co(II) 48 was capable of promoting asymmetric 5-exo iodolactonization with up to 83% ee.39b In 2010, Borhan,39c Yeung,39d Fujioka39n and Jacobsen39p respectively reported a highly enantioselective halolactonization capitalizing asymmetric organocatalyst, which unveiled a new strategy for catalytic asymmetric halocyclization.39 Since then, the catalytic asymmetric halolactonization of olefinic acid has achieved considerable advances and various asymmetric types of cyclization (e.g. 4-endo, 5-exo, 6-endo and 6-exo) were realized by employing different kinds of organocatalyst (Fig. 6).
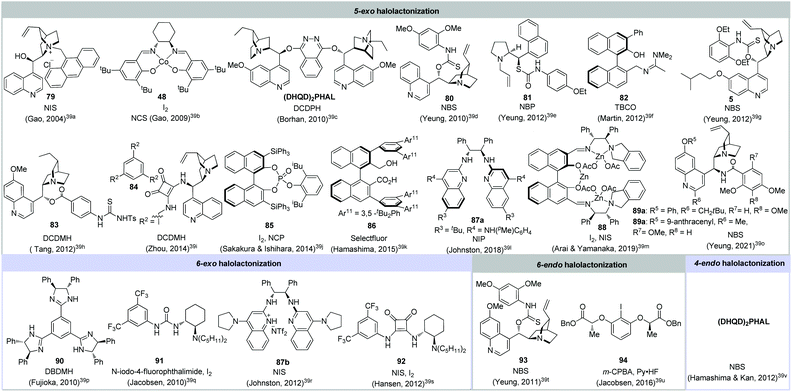 |
| Fig. 6 Developed catalysts and halogenating reagents employed for catalytic asymmetric halolactonization of alkenoic acids. | |
Surprisingly, catalytic asymmetric halolactonization is scarcely utilized in natural product synthesis. This could be ascribed to the substrate restriction, as only aromatic substituted alkenoic acids resulted in high to excellent enantioselectivities. However, these protocols provide an alternative route for constructing the enantioenriched aryl tertiary alcohol, which could be elaborated to natural products. In 2016, Hansen and co-workers reported the 6-exo asymmetric bromolactonization of δ-unsaturated carboxylic acids in the presence of 30 mol% chiral squaramide organocatalyst 97 (Scheme 11).40 To demonstrate its synthetic potential, chiral lactone 96a was prepared from carboxylic acid 95avia this protocol on a 5-gram scale in 93% yield with 81% ee, which was improved to 96% ee after recrystallization (Scheme 11). The sequence of radical debromination under the action of TMS3SiH/AIBN, treatment with an excess of MeLi and chemoselective elimination of the aliphatic tertiary alcohol mediated with POCl3/pyridine eventually gave (−)-gossonorol over seven steps in 34% yield and >99% ee. Further Shi epoxidation of (−)-gossonorol followed by TsOH-promoted cyclization of the tetrahydrofuran ring afforded (−)-boivinianin B.
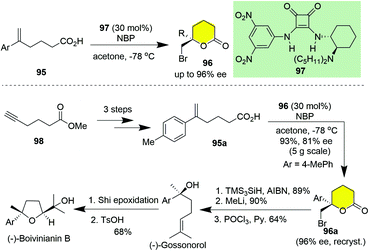 |
| Scheme 11 Hansen's asymmetric synthesis of (−)-gossonorol and (−)-boivinianin B by employing catalytic asymmetric 6-exo bromolactonization (Hansen). | |
(+)-Disparlure,41 (Z)-7,8-epoxy-2-methyloctadecane, is a sex attractant emitted by the female gypsy moth and has been utilized as the major attractant component in pheromone-containing traps for managing gypsy moth populations. In 2017, Martin and co-workers completed an 8-step synthesis of (+)-disparlure by applying the enantioselective 5-exo halolactonization of alkenoic acids catalyzed by a BINOL-amidine organocatalyst 81,42 which was previously developed by the same group.39f In this regard, the resulting chiral iodo-lactone served as the precursor of the epoxide moiety of (+)-disparlure. As shown in Scheme 12, Z-olefinic acid 101, prepared in 4 steps from pentynoic acid 100, was subjected to BINOL-amidine 81 catalyzed asymmetric 5-exo iodolactonization to produce γ-lactone 102 in 85% yield with 90% ee. Sequential semi-reduction of the lactone followed by a Wittig reaction smoothly led to the requisite epoxide 103. The selective reduction of the double bond of epoxide 102 was successively achieved by hydrogenolysis over PtO2 in hexane, thus accomplishing the catalytic enantioselective synthesis of (+)-disparlure in 33% overall yield in eight steps.
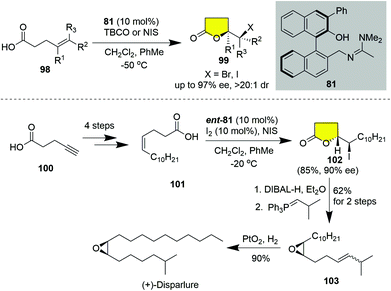 |
| Scheme 12 Total synthesis of (+)-disparlure by taking advantage of catalytic asymmetric 5-exo halolactonization (Martin). | |
4. Catalytic asymmetric halo-carbocyclization in natural product synthesis
The construction of carbocycles constitutes one of the major challenges in the total synthesis of cyclic natural products. In this regard, halo-carbocyclizations provide a facile entry to carbocycles from an olefinic substrate. In particular, the halonium-induced biomimetic polyene cyclization has been developed as a powerful tool for generating polycyclic carbocycles from linear precursors in excellent diastereoselectivities. Relying on those protocols, the racemic synthesis of polycyclic natural products has been elegantly achieved.43 However, catalytic asymmetric carbocyclization has achieved less progress compared with other types of catalytic asymmetric halocyclization. Unlike olefinic alcohols, acids and amines, the polyene substrate lacks the secondary interaction between the substrate and the catalyst in the transition state, thus leading to reduced or negligible enantioselectivities. To date, only limited successful examples of catalytic asymmetric halo-carbocyclization have been described.10
(−)-Boschnialactone is an iridoid monoterpene lactone isolated from Boschniakia rossica with insect-attracting and insecticidal activities.44 In 1995, Taguchi and co-workers developed the catalytic asymmetric iodocarbocyclization of diene 104 in the presence of a catalytic amount of Ti(TADDOLate)2, leading to the bicyclic lactone 106 in 80% yield with 99% ee upon heating after the completion of iodocyclization (Scheme 13).10 As shown in the transition state TS, the anion was derived from malonate complexed with Ti(TADDOLate)2 to form an octahedral Ti complex, which dictated the enantiofacial selectivity of the incoming iodonium and the attack of the nucleophile. Relying on this method, the desymmetrization of diene 107via Ti(TADDOLate)2-catalyzed asymmetric iodocarbocyclization was achieved in 99% ee with >12
:
1 dr (Scheme 13).45 Decarboxylation followed by reduction of the lactone and Bn-protection of the resulting diol gave bisbenzylether 109. Hydroboration/oxidation of the terminal olefin, Jones oxidation of the resulting primary alcohol and removal of benzyl by hydrogenation over Pd/C delivered lactone 110 mediated by Zn/NaI, affording (−)-boschnialactone in 10 LLS.
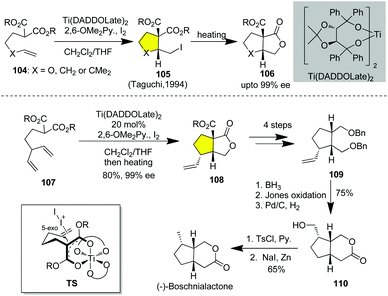 |
| Scheme 13 Catalytic asymmetric iodocarbocyclization of 4-alkenylmalonates and its application in the asymmetric synthesis of (−)-boschnialactone. | |
More recently, organocatalyzed asymmetric halo-carbocyclization has also emerged (Fig. 7). In 2016, Ishihara and Sakakura realized the first catalytic asymmetric polyene cyclization by chiral phosphite–urea bifunctional catalysts 111, albeit in low enantioselectivities, as a modification of their previous work.46 Soon after, Yamamoto and Samanta described catalytic asymmetric polyene cyclization by using a chiral BINOL-derived thiophosphoramide 112 and 1,3-dibromo-5,5-dimethylhydantoin (DBDMH) as a bromonium source with up to 94% ee.47 In 2018, Zhao and co-workers reported a chiral sulfide-catalyzed enantioselective chloro-carbocyclization of aryl-tethered diolefins and diaryl-tethered olefins via desymmetrization.48 By utilizing the same type of chiral sulfide 114,49 the same group developed the enantioselective carbocyclization of an aniline derivative for the construction of enantioenriched 3,4-functionalized tetrahydroquinolines. Although these protocols afford natural-like polycyclic skeletons, their strategic application in natural products remains unexplored.
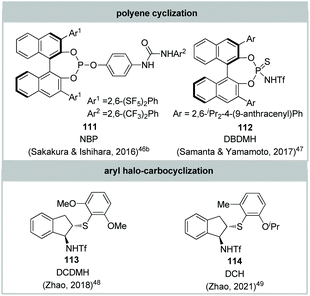 |
| Fig. 7 Other described organocatalysts and halogenating reagents employed for catalytic asymmetric halo-carbocyclization. | |
5. Conclusions and perspective
The renaissance and blooming of asymmetric organocatalysis are powering the rapid development of catalytic asymmetric halocyclization. In this context, natural product synthesis relying on catalytic asymmetric halocyclization has achieved considerable progresses in recent years. The catalytic asymmetric halocyclization not only provides a facile access to the enantioenriched cyclic skeletons of natural products, but also installs a halogen for further elaboration. In this respect, the versatile halogen has indeed exhibited great synthetic potential in some cases. However, dehalogenation of the enantioenriched halogenated building block is usually performed, thus lowering the atom economy and synthetic efficiency. On the other hand, halonium-induced catalytic asymmetric carbocyclization has witnessed little progress and is sparsely applied in complex natural product synthesis. In this context, the unsolved challenges in catalytic asymmetric halocyclization still evoke the design of more robust catalysts and powerful transformations. On the other hand, the strategic application of the developed catalytic asymmetric halocyclization in natural product synthesis still needs extensive exploration.
Conflicts of interest
There are no conflicts to declare.
Acknowledgements
We are grateful for financial support from the National Natural Science Foundation of China (grants 21722206, 21672171). Financial support from the Scientific Fund of Northwest A&F University is also acknowledged.
Notes and references
-
(a)
K. E. Harding and T. H. Tiner, in Comprehensive Organic Synthesis, ed. B. M. Trost and I. Fleming, Pergamon Press, Oxford, 1991, vol. 4, p. 363 Search PubMed
;
(b)
J. Mulzer, in Organic Synthesis Highlights, ed. J. Mulzer, H. J. Altenbach, M. Braun, K. Krohn and H. U. Reissig, VCH, Weinheim, 1991, p. 157 Search PubMed
;
(c)
F. Rodríguez and F. J. Fañanás, in Handbook of Cyclization Reactions, ed. S. Ma, Wiley-VCH, New York, 2010, vol. 4, pp. 951–990 Search PubMed
;
(d) M. D. Dowle and D. I. Davies, Synthesis and Synthetic Utility of Halolactones, Chem. Soc. Rev., 1979, 8, 171–197 RSC
;
(e) G. Cardillo and M. Orena, Stereocontrolled cyclofunctionalizations of Double Bonds through Heterocyclic Intermediates, Tetrahedron, 1990, 46, 3321–3408 CrossRef CAS
;
(f) S. Robin and G. R. Rousseaur, Electrophilic Cyclization of Unsaturated Amides, Tetrahedron, 1998, 54, 13681–13736 CrossRef CAS
;
(g) O. Kitagawa and T. Taguchi, Iodocarbocyclization and Iodoaminocyclization Reactions Mediated by a Metallic Reagent, Synlett, 1999, 1191–1199 CrossRef CAS
;
(h) A. N. French, S. Bissmire and T. Wirth, Iodine Electrophiles in Stereoselective Reactions: Recent Developments and Synthetic Applications, Chem. Soc. Rev., 2004, 33, 354–362 RSC
;
(i) S. Ranganathan, K. M. Muraleedharan, N. K. Vaish and N. Jayaraman, Halo- and Selenolactonisation: the Two Major Strategies for Cyclofunctionalisation, Tetrahedron, 2004, 60, 5273–5308 CrossRef CAS
;
(j) G. Li, S. R. S. Saibabu Kotti and C. Timmons, Recent Development of Regio- and Stereoselective Aminohalogenation Reaction of Alkenes, Eur. J. Org. Chem., 2007, 2745–2758 CrossRef CAS
;
(k) V. S. C. de Andrade and M. C. S. de Mattos, N-Halo Reagents: Modern Synthetic Approaches for Heterocyclic Synthesis, Synthesis, 2019, 51, 1841–1870 CrossRef CAS
;
(l) H. China, R. Kumar, K. Kikushima and T. Dohi, Halogen-Induced Controllable Cyclizations as Diverse Heterocycle Synthetic Strategy, Molecules, 2020, 25, 6007 CrossRef CAS PubMed
.
-
(a) B.-G. Wang, J. B. Gloer, N.-Y. Ji and J.-C. Zhao, Halogenated Organic Molecules of Rhodomelaceae Origin: Chemistry and Biology, Chem. Rev., 2013, 113, 3632–3685 CrossRef CAS PubMed
;
(b) W.-J. Chung and C. D. Vanderwal, Stereoselective Halogenation in Natural Product Synthesis, Angew. Chem., Int. Ed., 2016, 55, 4396–4434 CrossRef CAS PubMed
;
(c) I. Saikia, A. J. Borah and P. Phukan, Use of Bromine and Bromo-Organic Compounds in Organic Synthesis, Chem. Rev., 2016, 116, 6837–7042 CrossRef CAS PubMed
.
- I. Roberts and G. E. Kimball, The Halogenation of Ethylenes, J. Am. Chem. Soc., 1937, 59, 947–948 CrossRef CAS
.
-
(a) G. A. Olah and J. M. Bollinger, Stable Carbonium Ions. XLVIII. Halonium ion Formation via neighboring Halogen Participation. Tetramethylethylene Halonium Ions, J. Am. Chem. Soc., 1967, 89, 4744–4752 CrossRef CAS
;
(b) G. A. Olah, J. M. Bollinger and J. Brinich, Stable carbonium ions. LXII. Halonium Ion Formation via Neighboring Halogen Participation: Ethylenehalonium, Propylenehalonium, and 1,2-Dimethylethylenehalonium Ions, J. Am. Chem. Soc., 1968, 90, 2587–2594 CrossRef CAS
;
(c) J. Strating, J. H. Wieringa and H. Wynberg, The Isolation of a Stabilized Bromonium Ion, J. Chem. Soc. D, 1969, 907–908 RSC
;
(d) H. Slebocka-Tilk, R. G. Ball and R. S. Brown, The Question of Reversible Formation Of Bromonium Ions During the Course of Electrophilic Bromination of Olefins. 2. The Crystal and Molecular Structure of the Bromonium Ion of Adamantylideneadamantane, J. Am. Chem. Soc., 1985, 107, 4504–4508 CrossRef CAS
.
-
(a) R. E. Buckles, J. M. Bader and R. J. Thurmaier, Stereospecificity of the Addition of Bromine to cis- and trans-Stilbene, J. Org. Chem., 1962, 27, 4523–4527 CrossRef CAS
;
(b) R. C. Fahey and C. Schubert, Polar Additions to Olefins. I. The Chlorination of 2-Butene and 1-Phenylpropene, J. Am. Chem. Soc., 1965, 87, 5172–5179 CrossRef CAS
;
(c) J. H. Rolston and K. Yates, Polar Additions to the Styrene and 2-Butene Systems. I. Distribution Stereochemistry Of Bromination Products in Acetic Acid, J. Am. Chem. Soc., 1969, 91, 1469–1476 CrossRef CAS
;
(d) J. H. Rolston and K. Yates, Polar Additions to the Styrene and 2-Butene Systems. II. Medium Dependence of Bromination Products, J. Am. Chem. Soc., 1969, 91, 1477–1483 CrossRef CAS
;
(e) J. H. Rolston and K. Yates, Polar Additions to the Styrene and 2-Butene Systems. III. Kinetics annd Linear Free Energy Relations, J. Am. Chem. Soc., 1969, 91, 1483–1491 CrossRef CAS
;
(f) K. Yates and R. S. McDonald, Kinetics and Mechanisms of Electrophilic Addition. II. Thermochemical-kinetic Approach to Transition State Structure, J. Org. Chem., 1973, 38, 2465–2478 CrossRef CAS
.
-
(a) K. D. Ashtekar, M. Vetticatt, R. Yousefi, J. E. Jackson and B. Borhan, Nucleophile-Assisted Alkene Activation: Olefins Alone Are Often Incompetent, J. Am. Chem. Soc., 2016, 138, 8114–8119 CrossRef CAS PubMed
;
(b) N. S. Marzijarani, R. Yousefi, A. Jaganathan, K. D. Ashtekar, J. E. Jackson and B. Borhan, Absolute and Relative Facial Selectivities in Organocatalytic Asymmetric Chlorocyclization Reactions, Chem. Sci., 2018, 9, 2898–2908 RSC
;
(c) R. Yousefi, A. Sarkar, K. D. Ashtekar, D. C. Whitehead, T. Kakeshpour, D. Holmes, P. Reed, J. E. Jackson and B. Borhan, Mechanistic Insights into the Origin of Stereoselectivity in an Asymmetric Chlorolactonization Catalyzed by (DHQD)2PHAL, J. Am. Chem. Soc., 2020, 142, 7179–7189 CrossRef CAS PubMed
;
(d) R. Van Lommel, J. Bock, C. G. Daniliuc, U. Hennecke and F. De Proft, A Dynamic Picture of the Halolactonization reaction through a Combination of Ab Initio Metadynamics and Experimental Investigations, Chem. Sci., 2021, 12, 7746–7757 RSC
.
-
(a) G. Bellucci, R. Bianchini, C. Chiappe, F. Marioni, R. Ambrosetti, R. S. Brown and H. Slebocka-Tilk, The Solution Behavior of the Adamantylideneadamantane-Bromine System: Existence of equilibrium Mixtures of Bromonium-Polybromide Salts and a Strong 1
:
1 Molecular Charge-Transfer Complex, J. Am. Chem. Soc., 1989, 111, 2640–2647 CrossRef CAS
;
(b) A. J. Bennet, R. S. Brown, R. E. D. McClung, M. Klobukowski, G. H. M. Aarts, B. D. Santarsiero, G. Bellucci and R. Bianchini, An unprecedented Rapid And Direct Bromine(1 + ) Ion Transfer from theBbromonium Ion of Adamantylideneadamantane to Acceptor Olefins, J. Am. Chem. Soc., 1991, 113, 8532–8534 CrossRef CAS
;
(c) R. S. Brown, R. W. Nagorski, A. J. Bennet, R. E. D. McClung, G. H. M. Aarts, M. Klobukowski, R. McDonald and B. D. Santarsiero, Stable Bromonium and Iodonium Ions of the Hindered Olefins Adamantylideneadamantane and Bicyclo[3.3.1]nonylidenebicyclo[3.3.1]nonane. X-Ray Structure, Transfer of Positive Halogens to Acceptor Olefins, and ab Initio Studies, J. Am. Chem. Soc., 1994, 116, 2448–2456 CrossRef CAS
;
(d) R. S. Brown, Investigation of the Early Steps in Electrophilic Bromination through the Study of the Reaction with Sterically Encumbered Olefins, Acc. Chem. Res., 1997, 30, 131–137 CrossRef CAS
.
-
(a) D. C. Braddock, S. A. Hermitage, L. Kwok, R. Pouwer, J. M. Redmond and A. J. P. White, The Generation and Trapping of Enantiopure Bromonium ions, Chem. Commun., 2009, 1082–1084 RSC
;
(b) S. E. Denmark, M. T. Burk and A. J. Hoover, On the Absolute Configurational Stability of Bromonium and Chloronium Ions, J. Am. Chem. Soc., 2010, 132, 1232–1233 CrossRef CAS PubMed
.
-
(a) O. Kitagawa, T. Hanano, K. Tanabe, M. Shiro and T. Taguchi, Enantioselective Halocyclization Reaction Using a Chiral Titanium Complex, J. Chem. Soc., Chem. Commun., 1992, 1005–1007 RSC
;
(b) R. B. Grossman and R. J. Trupp, The First Reagent-Controlled Asymmetric Halolactonizations. Dihydroquinidine-Halogen Complexes as Chiral Sources of Positive Halogen Ion, Can. J. Chem., 1998, 76, 1233–1237 CrossRef CAS
;
(c) X.-L. Cui and R. S. Brown, Mechanistic Evaluation of the Halocyclization of 4-Penten-1-ol by Some Bis(2-substituted pyridine) and Bis(2,6-disubstituted pyridine)bromonium Triflates, J. Org. Chem., 2000, 65, 5653–5658 CrossRef CAS PubMed
;
(d) J. Haas, S. Piguel and T. Wirth, Reagent-Controlled Stereoselective Iodolactonizations, Org. Lett., 2002, 4, 297–300 CrossRef CAS PubMed
.
- T. Inoue, O. Kitagawa, O. Ochiai, M. Shiro and T. Taguchi, Catalytic Asymmetric Iodocarbocyclization reaction, Tetrahedron Lett., 1995, 36, 9333–9336 CrossRef CAS
.
- or selected reviews on organocatalysis, see:
(a)
Enantioselective Organocatalyzed Reactions, ed. R. Mahrwald, Springer, New York, 2011 Search PubMed
;
(b)
Comprehensive Enantioselective Organocatalysis: Catalysts, Reactions, and Applications, ed. P. I. Dalko, Wiley-VCH, Weinheim, 2013 Search PubMed
;
(c) D. W. C. MacMillan, Nature, 2008, 455, 304–308 CrossRef CAS PubMed
;
(d) S. Mukherjee, J. W. Yang, S. Hoffmann and B. List, Chem. Rev., 2007, 107, 5471–5569 CrossRef CAS PubMed
;
(e) A. Vega-Penaloza, S. Paria, M. Bonchio, L. Dell'Amico and X. Companyo, ACS Catal., 2019, 9, 6058–6072 CrossRef CAS
;
(f) T. Akiyama, Chem. Rev., 2007, 107, 5744–5758 CrossRef CAS PubMed
;
(g) R. J. Phipps, G. L. Hamilton and F. D. Toste, Nat. Chem., 2012, 4, 603–614 CrossRef CAS PubMed
;
(h) M. Mahlau and B. List, Angew. Chem., Int. Ed., 2013, 52, 518–533 CrossRef CAS PubMed
.
-
(a) M. P. Brochu, S. P. Brown and D. W. C. MacMillan, Direct and Enantioselective Organocatalytic α-Chlorination of Aldehydes, J. Am. Chem. Soc., 2004, 126, 4108–4109 CrossRef CAS PubMed
;
(b) N. Halland, A. Braunton, S. Bachmann, M. Marigo and K. A. Jørgensen, Direct Organocatalytic Asymmetric α-Chlorination of Aldehydes, J. Am. Chem. Soc., 2004, 126, 4790–4791 CrossRef CAS PubMed
.
-
(a) R. Britton and B. Kang, α-Haloaldehydes: versatile building blocks for natural product synthesis, Nat. Prod. Rep., 2013, 30, 227–236 RSC
;
(b) P. J. Chevis and S. G. Pyne, Synthesis of enantioenriched α-heteroatom functionalised aldehydes by chiral organocatalysis and their synthetic applications, Org. Chem. Front., 2021, 8, 2287–2314 RSC
.
- For review:
(a) J. Bock, S. Guria, V. Wedek and U. Hennecke, Enantioselective Dihalogenation of Alkenes, Chem. – Eur. J., 2021, 27, 4517–4530 CrossRef CAS PubMed
For selected examples:
(b) K. C. Nicolaou, N. L. Simmons, Y. Ying, P. M. Heretsch and J. S. Chen, Enantioselective Dichlorination of Allylic Alcohols, J. Am. Chem. Soc., 2011, 133, 8134–8137 CrossRef CAS PubMed
;
(c) S. M. Banik, J. W. Medley and E. N. Jacobsen, Catalytic, Diastereoselective 1,2-Difluorination of Alkenes, J. Am. Chem. Soc., 2016, 138, 5000–5003 CrossRef CAS PubMed
;
(d) W.-S. Huang, L. Chen, Z.-J. Zheng, K.-F. Yang, Z. Xu, Y.-M. Cui and L.-W. Xu, Catalytic asymmetric Bromochlorination of Aromatic Allylic Alcohols Promoted by Multifunctional Schiff Base Ligands, Org. Biomol. Chem., 2016, 14, 7927–7932 RSC
;
(e) V. Wedek, R. Van Lommel, C. G. Daniliuc, F. De Proft and U. Hennecke, Organocatalytic, Enantioselective Dichlorination of Unfunctionalized Alkenes, Angew. Chem., Int. Ed., 2019, 58, 9239–9243 CrossRef CAS PubMed
.
-
(a) D. X. Hu, G. M. Shibuya and N. Z. Burns, Catalytic Enantioselective Dibromination of Allylic Alcohols, J. Am. Chem. Soc., 2013, 135, 12960–12963 CrossRef CAS PubMed
;
(b) D. X. Hu, F. J. Seidl, C. Bucher and N. Z. Burns, Catalytic Chemo-, Regio-, and Enantioselective Bromochlorination of Allylic Alcohols, J. Am. Chem. Soc., 2015, 137, 3795–3798 CrossRef CAS PubMed
;
(c) M. L. Landry, D. X. Hu, G. M. McKenna and N. Z. Burns, Catalytic Enantioselective Dihalogenation and the Selective Synthesis of (−)-Deschloromytilipin A and (−)-Danicalipin A, J. Am. Chem. Soc., 2016, 138, 5150–5158 CrossRef CAS PubMed
For review on the synthetic applications:
(d) M. L. Landry and N. Z. Burns, Catalytic Enantioselective Dihalogenation in Total Synthesis, Acc. Chem. Res., 2018, 51, 1260–1271 CrossRef CAS PubMed
.
- For reviews on catalytic asymmetric halocyclization, see:
(a) S. France, A. Weatherwax and T. Lectka, Recent Developments in Catalytic, Asymmetric α-Halogenation: A New Frontier in Asymmetric Catalysis, Eur. J. Org. Chem., 2005, 475–479 CrossRef CAS
;
(b) G. Chen and S. Ma, Enantioselective Halocyclization Reactions for the Synthesis of Chiral Cyclic Compounds, Angew. Chem., Int. Ed., 2010, 49, 8306–8308 CrossRef CAS PubMed
;
(c) A. Castellanos and S. P. Fletcher, Current Methods for Asymmetric Halogenation of Olefins, Chem. – Eur. J., 2011, 17, 5766–5776 CrossRef CAS PubMed
;
(d) C. K. Tan, L. Zhou and Y.-Y. Yeung, Organocatalytic Enantioselective Halolactonizations: Strategies of Halogen Activation, Synlett, 2011, 1335–1339 CAS
;
(e) S. E. Denmark, W. E. Kuester and M. T. Burk, Catalytic, Asymmetric Halofunctionalization of Alkenes—A Critical Perspective, Angew. Chem., Int. Ed., 2012, 51, 10938–10953 CrossRef CAS PubMed
;
(f) U. Hennecke, New Catalytic Approaches towards the Enantioselective Halogenation of Alkenes, Chem. – Asian J., 2012, 7, 456–465 CrossRef CAS PubMed
;
(g) C. K. Tan and Y.-Y. Yeung, Recent advances in stereoselective bromofunctionalization of alkenes using N-bromoamide reagents, Chem. Commun., 2013, 49, 7985–7996 RSC
;
(h) C. B. Tripathi and S. Mukherjee, Catalytic Enantioselective Halocyclizations beyond Lactones: Emerging Routes to Enantioenriched Nitrogenous Heterocycles, Synlett, 2014, 25, 163–169 CAS
;
(i) S. Zheng, C. M. Schienebeck, W. Zhang, H.-Y. Wang and W. Tang, Cinchona Alkaloids as Organocatalysts in Enantioselective Halofunctionalization of Alkenes and Alkynes, Asian J. Org. Chem., 2014, 3, 366–376 CrossRef CAS
;
(j) M. H. Gieuw, Z. Ke and Y.-Y. Yeung, Lewis Base Catalyzed Stereo- and Regioselective Bromocyclization, Chem. Rec., 2017, 17, 287–311 CrossRef CAS PubMed
;
(k) Y. Kawato and Y. Hamashima, Enantioselective Bromocyclization of Allylic Amides Mediated by Phosphorus Catalysis, Synlett, 2018, 29, 1257–1271 CrossRef CAS
;
(l) B. Maji, Stereoselective Halonium, Thiiranium and Seleniranium Ion-Triggered Friedel–Crafts-Type Alkylations for Polyene Cyclizations, Adv. Synth. Catal., 2019, 361, 3453–3489 CrossRef CAS
;
(m) J. Wong and Y.-Y. Yeung, Recent Advances in C–Br Bond Formation, Synlett, 2021, 32, 1354–1364 CrossRef CAS
For the example of constucting polyclic scaffolds via catalytic asymmetric cascade cyclization, see:
(n) T. Zheng, X. Wang, W.-H. Ng, Y.-L. S. Tse and Y.-Y. Yeung, Catalytic enantio- and diastereoselective domino halocyclization and spiroketalization, Nat. Catal., 2020, 3, 993–1001 CrossRef CAS
.
-
(a) D. O'Hagan, Pyrrole, Pyrrolidine, Pyridine, Piperidine and Tropane Alkaloids, Nat. Prod. Rep., 2000, 17, 435–446 RSC
;
(b) F.-X. Felpin and J. Lebreton, Recent Advances in the Total Synthesis of Piperidine and Pyrrolidine Natural Alkaloids with Ring-Closing Metathesis as a Key Step, Eur. J. Org. Chem., 2003, 3693–3712 CrossRef CAS
;
(c) X. Wen-Fang, C. Xian-Chao, W. Qiang and F. Hao, Advances in Matrix Metalloproteinase Inhibitors Based on Pyrrolidine Scaffold, Curr. Med. Chem., 2008, 15, 374–385 CrossRef
.
- For selected examples of applications of halo-aminocyclization in natural product synthesis:
(a) Y. G. Kim and J. K. Cha, DivergentSyntheses of Stereoisomers of Swainsonine: (−)-8-epi-, (−)-8a-epi- and (−)-8,8a-diepi-Swainsonine, Tetrahedron Lett., 1989, 30, 5721–5724 CrossRef CAS
;
(b) Y.-Y. Yeung, S. Hong and E. J. Corey, A Short Enantioselective Pathway for the Synthesis of the Anti-Influenza Neuramidase Inhibitor Oseltamivir from 1,3-Butadiene and Acrylic Acid, J. Am. Chem. Soc., 2006, 128, 6310–6311 CrossRef CAS PubMed
;
(c) D. C. Beshore and A. B. Smith, Total Syntheses of ( + )-Lyconadin A and (−)-Lyconadin B, J. Am. Chem. Soc., 2007, 129, 4148–4149 CrossRef CAS PubMed
;
(d) D. C. Beshore and A. B. Smith, The Lyconadins: Enantioselective Total Syntheses of ( + )-Lyconadin A and (−)-Lyconadin B, J. Am. Chem. Soc., 2008, 130, 13778–13789 CrossRef CAS PubMed
;
(e) S. G. Davies, A. L. A. Figuccia, A. M. Fletcher, P. M. Roberts and J. E. Thomson, Asymmetric Syntheses of (−)-1-Deoxymannojirimycin and ( + )-1-Deoxyallonojirimycin via a Ring-Expansion Approach, Org. Lett., 2013, 15, 2042–2045 CrossRef CAS PubMed
.
- For catalytic asymmetric 5-exo aminocyclization:
(a) L. Zhou, J. Chen, C. K. Tan and Y.-Y. Yeung, Enantioselective Bromoaminocyclization Using Amino–Thiocarbamate Catalysts, J. Am. Chem. Soc., 2011, 133, 9164–9167 CrossRef CAS PubMed
;
(b) F. Chen, C. K. Tan and Y.-Y. Yeung, C2-Symmetric Cyclic Selenium-Catalyzed Enantioselective Bromoaminocyclization, J. Am. Chem. Soc., 2013, 135, 1232–1235 CrossRef CAS PubMed
;
(c) P. Mizar, A. Burrelli, E. Günther, M. Söftje, U. Farooq and T. Wirth, Organocatalytic Stereoselective Iodoamination of Alkenes, Chem. – Eur. J., 2014, 20, 13113–13116 CrossRef CAS PubMed
;
(d) H.-J. Jiang, K. Liu, J. Yu, L. Zhang and L.-Z. Gong, Switchable Stereoselectivity in Bromoaminocyclization of Olefins: Using Brønsted Acids of Anionic Chiral Cobalt(III) Complexes, Angew. Chem., Int. Ed., 2017, 56, 11931–11935 CrossRef CAS PubMed
;
(e) S.-N. Yu, Y.-L. Li and J. Deng, Enantioselective Synthesis of 2-Bromomethyl Indolines via BINAP(S)-Catalyzed Bromoaminocyclization of Allyl Aniline, Adv. Synth. Catal., 2017, 359, 2499–2508 CrossRef CAS
;
(f) Y. Lu, H. Nakatsuji, Y. Okumura, L. Yao and K. Ishihara, Enantioselective Halo-oxy- and Halo-azacyclizations Induced by Chiral Amidophosphate Catalysts and Halo-Lewis Acids, J. Am. Chem. Soc., 2018, 140, 6039–6043 CrossRef CAS PubMed
;
(g) T. Nakamura, K. Okuno, K. Kaneko, M. Yamanaka and S. Shirakawa, Chiral Bifunctional Sulfide-Catalyzed Asymmetric Bromoaminocyclizations, Org. Biomol. Chem., 2020, 18, 3367–3373 RSC
For 5-endo catalytic asymmetric aminocyclization:
(h) J. Chen, L. Zhou and Y.-Y. Yeung, A Highly Enantioselective Approach towards 2-Substituted 3-Bromopyrrolidines, Org. Biomol. Chem., 2012, 10, 3808–3811 RSC
;
(i) W. Q. Xie, G. D. Jiang, H. Liu, J. D. Hu, X. X. Pan, H. Zhang, X. L. Wan, Y. S. Lai and D. W. Ma, Highly Enantioselective Bromocyclization of Tryptamines and Its Application in the Synthesis of (-)-Chimonanthine, Angew. Chem., Int. Ed., 2013, 52, 12924–12927 CrossRef CAS PubMed
;
(j) Q. Cai, Q. Yin and S.-L. You, Chiral-Amine-Catalyzed Asymmetric Bromocyclization of Tryptamine Derivatives, Asian J. Org. Chem., 2014, 3, 408–411 CrossRef CAS
;
(k) K. Liu, H.-J. Jiang, N. Li, H. Li, J. Wang, Z.-Z. Zhang and J. Yu, Enantioselective Bromocyclization of Tryptamines Induced by Chiral Co(III)-Complex-Templated Brønsted Acids under an Air Atmosphere, J. Org. Chem., 2018, 83, 6815–6823 CrossRef CAS PubMed
;
(l) H. Wang, H. Zhong, X. Xu, W. Xu and X. Jiang, Catalytic Enantioselective Bromoaminocyclization and Bromocycloetherification, Adv. Synth. Catal., 2020, 362, 5358–5362 CrossRef CAS
.
-
(a) H. Huang, H. Pan, Y. Cai, M. Liu, H. Tian and Y. Shi, Enantioselective 6-endo bromoaminocyclization of 2,4-dienyl N-tosylcarbamates catalyzed by a chiral phosphine oxide-Sc(OTf)3 complex. A dramatic additive effect, Org. Biomol. Chem., 2015, 13, 3566–3570 RSC
;
(b) Z. Li and Y. Shi, Chiral Phosphine Oxide–Sc(OTf)3 Complex Catalyzed Enantioselective Bromoaminocyclization of 2-Benzofuranylmethyl N-Tosylcarbamates. Approach to a Novel Class of Optically Active Spiro Compounds, Org. Lett., 2015, 17, 5752–5755 CrossRef CAS PubMed
;
(c) W. Liu, H. Pan, H. Tian and Y. Shi, Enantioselective 6-exo-Bromoaminocyclization of Homoallylic N-Tosylcarbamates Catalyzed by a Novel Monophosphine-Sc(OTf)3 C omplex, Org. Lett., 2015, 17, 3956–3959 CrossRef CAS PubMed
;
(d) H. Pan, H. Huang, W. Liu, H. Tian and Y. Shi, Phosphine Oxide–Sc(OTf)3 C atalyzed Highly Regio- and Enantioselective Bromoaminocyclization of (E)-Cinnamyl Tosylcarbamates. An Approach to a Class of Synthetically Versatile Functionalized Molecules, Org. Lett., 2016, 18, 896–899 CrossRef CAS PubMed
.
- For reviews, see:
(a)
U. Anthoni, C. Christophersen and P. H. Nielsen, in Alkaloids: Chemical and Biological Perspectives, ed. S. W. Pelletier, Pergamon, New York, 1999, vol. 13, pp. 163–236 Search PubMed
;
(b)
G. A. Cordell and J. E. Saxton, in The Alkaloids: Chemistry and Physiology, ed. R. H. F. Manske and R. G. A. Rodrigo, Academic Press, New York, 1981, vol. 20, pp. 3–295 Search PubMed
;
(c)
T. Hino and M. Nakagawa, in The Alkaloids: Chemistry and Pharmacology, ed. A. Brossi, Academic Press, New York, 1989, vol. 34, pp. 1–75 Search PubMed
;
(d)
T. S. Pvenet and J. Pusset, in The Alkaloids: Chemistry and Pharmacology, ed. G. A. Cordell, Academic Press, New York, 1996, vol. 48, pp. 1–73 Search PubMed
For synthesis of cyclotryptamine alkaloids:
(e) D. Crich and A. Banerjee, Chemistry of the Hexahydropyrrolo[2,3-b]indoles: Configuration, Conformation, Reactivity, and Applications in Synthesis, Acc. Chem. Res., 2007, 40, 151–161 CrossRef CAS PubMed
;
(f) P. Ruiz-Sanchis, S. A. Savina, F. Albericio and M. Álvarez, Structure, Bioactivity and Synthesis of Natural Products with Hexahydropyrrolo[2,3-b]indole, Chem. – Eur. J., 2011, 17, 1388–1408 CrossRef CAS PubMed
;
(g) L. M. Repka and S. E. Reisman, Recent Developments in the Catalytic, Asymmetric Construction of Pyrroloindolines Bearing All-Carbon Quaternary Stereocenters, J. Org. Chem., 2013, 78, 12314–12320 CrossRef CAS PubMed
;
(h) M. Büschleb, S. Dorich, S. Hanessian, D. Tao, K. B. Schenthal and L. E. Overman, Synthetic Strategies toward Natural Products Containing Contiguous Stereogenic Quaternary Carbon Atoms, Angew. Chem., Int. Ed., 2016, 55, 4156–4186 CrossRef PubMed
.
-
(a) A. R. Pape, K. P. Kaliappan and E. P. Kundig, Transition-Metal-Mediated Dearomatization Reactions, Chem. Rev., 2000, 100, 2917–2940 CrossRef CAS PubMed
;
(b) S. P. Roche and J. A. Porco Jr., Dearomatization Strategies in the Synthesis of Complex Natural Products, Angew. Chem., Int. Ed., 2011, 50, 4068–4093 CrossRef CAS PubMed
;
(c) C. X. Zhuo, W. Zhang and S. L. You, Catalytic Asymmetric Dearomatization Reactions, Angew. Chem., Int. Ed., 2012, 51, 12662–12686 CrossRef PubMed
;
(d) C. Zheng and S. L. You, Catalytic Asymmetric Dearomatization by Transition-Metal Catalysis: A Method for Transformations of Aromatic Compounds, Chem, 2016, 1, 830–857 CrossRef CAS
;
(e) W. C. Wertjes, E. H. Southgate and D. Sarlah, Recent advances in chemical dearomatization of nonactivated arenes, Chem. Soc. Rev., 2018, 47, 7996–8017 RSC
.
- For reviews: for selected recent examples:
(a) M. Movassaghi and M. A. Schmidt, Concise total synthesis of (-)-calycanthine, ( + )-chimonanthine, and ( + )-folicanthine, Angew. Chem., Int. Ed., 2007, 46, 3725–3728 CrossRef CAS PubMed
;
(b) H. Mitsunuma, M. Shibasaki, M. Kanai and S. Matsunaga, Catalytic Asymmetric Total Synthesis of Chimonanthine, Folicanthine, and Calycanthine through Double Michael Reaction of Bisoxindole, Angew. Chem., Int. Ed., 2012, 51, 5217–5221 CrossRef CAS PubMed
;
(c) R. R. Liu and J. L. Zhang, Organocatalytic Michael Addition of Indoles to Isatylidene-3-acetaldehydes: Application to the Formal Total Synthesis of (-)-Chimonanthine, Org. Lett., 2013, 15, 2266–2269 CrossRef CAS PubMed
;
(d) S. P. Lathrop and M. Movassaghi, Application of diazene-directed fragment assembly to the total synthesis and stereochemical assignment of ( + )-desmethyl-meso-chimonanthine and related heterodimeric alkaloids, Chem. Sci., 2014, 5, 333–340 RSC
;
(e) M. Ding, K. J. Liang, R. Pan, H. B. Zhang and C. F. Xia, Total Synthesis of ( + )-Chimonanthine, ( + )-Folicanthine, and (-)-Calycanthine, J. Org. Chem., 2015, 80, 10309–10316 CrossRef CAS PubMed
;
(f) K. N. Babu, A. Roy, M. Singh and A. Bisai, Thiourea-Catalyzed Enantioselective Malonate Addition onto 3-Sulfonyl-3 ‘-indolyl-2-oxindoles: Formal Total Syntheses of (-)-Chimonanthine, (-)-Folicanthine, and ( + )-Calycanthine, Org. Lett., 2018, 20, 6327–6331 CrossRef CAS PubMed
.
-
(a) K.-H. Lim, T. Etoh, M. Hayashi, K. Komiyama and T.-S. Kam, Conolutinine, a Hexacyclic Indole Alkaloid with a Novel Ring System Incorporating a Diazaspiro Center and Fused Oxadiazepine–Tetrahydrofuran Rings, Tetrahedron Lett., 2009, 50, 752–754 CrossRef CAS
;
(b) X. Feng, G. Jiang, Z. Xia, J. Hu, X. Wan, J.-M. Gao, Y. Lai and W. Xie, Total Synthesis of (−)-Conolutinine, Org. Lett., 2015, 17, 4428–4431 CrossRef CAS PubMed
.
-
(a) A. D. Lebsack, J. T. Link, L. E. Overman and B. A. Stearns, Enantioselective Total Synthesis of Quadrigemine C and Psycholeine, J. Am. Chem. Soc., 2002, 124, 9008–9009 CrossRef CAS PubMed
;
(b) J. J. Kodanko and L. E. Overman, Enantioselective Total Syntheses of the Cyclotryptamine Alkaloids Hodgkinsine and Hodgkinsine B, Angew. Chem., Int. Ed., 2003, 42, 2528–2531 CrossRef CAS PubMed
;
(c) L. E. Overman and E. A. Peterson, Enantioselective Total Synthesis of The Cyclotryptamine Alkaloid Idiospermuline, Angew. Chem., Int. Ed., 2003, 42, 2525–2528 CrossRef CAS PubMed
;
(d) K. Foo, T. Newhouse, I. Mori, H. Takayama and P. S. Baran, Total Synthesis Guided Structure Elucidation of ( + )-Psychotetramine, Angew. Chem., Int. Ed., 2011, 50, 2716–2719 CrossRef CAS PubMed
;
(e) R. H. Snell, R. L. Woodward and M. C. Willis, Catalytic Enantioselective Total Synthesis of Hodgkinsine B, Angew. Chem., Int. Ed., 2011, 50, 9116–9119 CrossRef CAS PubMed
;
(f) C. R. Jamison, J. J. Badillo, J. M. Lipshultz, R. J. Comito and D. W. C. MacMillan, Catalyst-Controlled Oligomerization for the Collective Synthesis of Polypyrroloindoline Natural Products, Nat. Chem., 2017, 9, 1165–1169 CrossRef CAS PubMed
;
(g) P. Lindovska and M. Movassaghi, Concise Synthesis of (-)-Hodgkinsine, (-)-Calycosidine, (-)-Hodgkinsine B, (-)-Quadrigemine C, and (-)-Psycholeine via Convergent and Directed Modular Assembly of Cyclotryptamines, J. Am. Chem. Soc., 2017, 139, 17590–17596 CrossRef CAS PubMed
.
-
(a) A. Numata, C. Takahashi, Y. Ito, T. Takada, K. Kawai, Y. Usami, E. Matsumura, M. Imachi, T. Ito and T. Hasegawa, Communesins, Cytotoxic Metabolites of a Fungus Isolated from a Marine Alga, Tetrahedron Lett., 1993, 34, 2355–2358 CrossRef CAS
;
(b) H. Hayashi, H. Matsumoto and K. Akiyama, New Insecticidal Compounds, Communesins C, D and E, from Penicillium expansum Link MK-57, Biosci. Biotechnol. Biochem., 2004, 68, 753–756 CrossRef CAS PubMed
;
(c) R. Jadulco, R. A. Edrada, R. Ebel, A. Berg, K. Schaumann, V. Wray, K. Steube and P. Proksch, New Communesin Derivatives from the Fungus Penicillium sp. Derived from the Mediterranean Sponge Axinella verrucosa, J. Nat. Prod., 2004, 67, 78–81 CrossRef CAS PubMed
;
(d) P. W. Dalsgaard, J. W. Blunt, M. H. G. Munro, J. C. Frisvad and C. Christophersen, Communesins G and H, New Alkaloids from the Psychrotolerant Fungus Penicillium Rivulum, J. Nat. Prod., 2005, 68, 258–261 CrossRef CAS PubMed
.
- For reviews:
(a) P. Siengalewicz, T. Gaich and J. Mulzer, It All Began with an Error: The Nomofungin/Communesin Story, Angew. Chem., Int. Ed., 2008, 47, 8170–8176 CrossRef CAS PubMed
;
(b) Z. Zuo and D. Ma, Synthetic Studies toward Communesins, Israel J. Chem., 2011, 51, 434–441 CrossRef CAS
;
(c) B. M. Trost and M. Osipov, Recent Advances on the Total Syntheses of Communesin Alkaloids and Perophoramidine, Chem. – Eur. J., 2015, 21, 16318–16343 CrossRef CAS PubMed
For recent syntheses:
(d) S. P. Lathrop, M. Pompeo, W. T. T. Chang and M. Movassaghi, Convergent and Biomimetic Enantioselective Total Synthesis of (-)-Communesin F, J. Am. Chem. Soc., 2016, 138, 7763–7769 CrossRef CAS PubMed
;
(e) X. Liang, T. Y. Zhang, X. Y. Zeng, Y. Zheng, K. Wei and Y. R. Yang, Ir-Catalyzed Asymmetric Total Synthesis of (-)-Communesin F, J. Am. Chem. Soc., 2017, 139, 3364–3367 CrossRef CAS PubMed
;
(f) J. Park, A. Jean and D. Y.-K. Chen, Asymmetric Total Syntheses of Communesin F and a Putative Member of the Communesin Family, Angew. Chem., Int. Ed., 2017, 56, 14237–14240 CrossRef CAS PubMed
;
(g) M. M. Pompeo, J. H. Cheah and M. Movassaghi, Total Synthesis and Anti-Cancer Activity of All Known Communesin Alkaloids and Related Derivatives, J. Am. Chem. Soc., 2019, 141, 14411–14420 CrossRef CAS PubMed
.
- For selected reviews:
(a) J. Marco-Contelles, M. T. Molina and S. Anjum, Naturally Occurring Cyclohexane Epoxides: Sources, Biological Activities, and Synthesis, Chem. Rev., 2004, 104, 2857–2900 CrossRef CAS PubMed
;
(b) J. Rutkowski and B. Brzezinski, Structures and Properties of Naturally Occurring Polyether Antibiotics, BioMed. Res. Int., 2013, 2013, 162513 Search PubMed
;
(c) J. W. Blunt, B. R. Copp, R. A. Keyzers, M. H. G. Munro and M. R. Prinsep, Marine natural products, Nat. Prod. Rep., 2016, 33, 382–431 RSC
.
- For selected reviews:
(a) A. Deiters and S. F. Martin, Synthesis of Oxygen- and Nitrogen-Containing Heterocycles by Ring-Closing Metathesis, Chem. Rev., 2004, 104, 2199–2238 CrossRef CAS PubMed
;
(b) M. Inoue, Convergent Strategies for Syntheses of trans-Fused Polycyclic Ethers, Chem. Rev., 2005, 105, 4379–4405 CrossRef CAS PubMed
;
(c) I. Kadota and Y. Yamamoto, Synthetic Strategies of Marine Polycyclic Ethers via Intramolecular Allylations: Linear and Convergent Approaches, Acc. Chem. Res., 2005, 38, 423–432 CrossRef CAS PubMed
;
(d) N. Li, Z. Shi, Y. Tang, J. Chen and X. Li, Recent Progress On The Total Synthesis of Acetogenins from Annonaceae, Beilstein J. Org. Chem., 2008, 4, 48 Search PubMed
;
(e) I. Vilotijevic and T. F. Jamison, Epoxide-Opening Cascades in the Synthesis of Polycyclic Polyether Natural Products, Angew. Chem., Int. Ed., 2009, 48, 5250–5281 CrossRef CAS PubMed
;
(f) T. Nakata, SmI2-Induced Reductive Cyclizations for the Synthesis of Cyclic Ethers and Applications In Natural Product Synthesis, Chem. Soc. Rev., 2010, 39, 1955–1972 RSC
;
(g) R. L. Davis, J. Stiller, T. Naicker, H. Jiang and K. A. Jørgensen, Asymmetric Organocatalytic Epoxidations: Reactions, Scope, Mechanisms, and Applications, Angew. Chem., Int. Ed., 2014, 53, 7406–7426 CrossRef CAS PubMed
;
(h) T. Martín, J. I. Padrón and V. S. Martín, Strategies for the Synthesis of Cyclic Ethers of Marine Natural Products, Synlett, 2014, 25, 12–32 CrossRef
.
- Selected examples:
(a) Y. Adachi, N. Kamei, S. Yokoshima and T. Fukuyama, Total Synthesis of (−)-Histrionicotoxin, Org. Lett., 2011, 13, 4446–4449 CrossRef CAS PubMed
;
(b) S. A. Snyder, A. P. Brucks, D. S. Treitler and I. Moga, Concise Synthetic Approaches for the Laurencia Family: Formal Total Syntheses of (±)-Laurefucin and (±)-E- and (±)-Z-Pinnatifidenyne, J. Am. Chem. Soc., 2012, 134, 17714–17721 CrossRef CAS PubMed
;
(c) N. Alnafta, J. P. Schmidt, C. L. Nesbitt and C. S. P. McErlean, Total Synthesis of ( + )-Panacene, Org. Lett., 2016, 18, 6520–6522 CrossRef CAS PubMed
;
(d) J. Clarke, K. J. Bonney, M. Yaqoob, S. Solanki, H. S. Rzepa, A. J. P. White, D. S. Millan and D. C. Braddock, Epimeric Face-Selective Oxidations and Diastereodivergent Transannular Oxonium Ion Formation Fragmentations: Computational Modeling and Total Syntheses of 12-Epoxyobtusallene IV, 12-Epoxyobtusallene II, Obtusallene X, Marilzabicycloallene C, and Marilzabicycloallene D, J. Org. Chem., 2016, 81, 9539–9552 CrossRef CAS PubMed
;
(e) Y. Ogura, H. Sato and S. Kuwahara, Total Synthesis of Amphirionin-4, Org. Lett., 2016, 18, 2399–2402 CrossRef CAS PubMed
;
(f) Y. Yoshikawa, M. Yamakawa, T. Kobayashi, K. Murai, M. Arisawa, M. Sumimoto and H. Fujioka, First Asymmetric Total Synthesis and Insight into the Structure of Laurenidificin, Eur. J. Org. Chem., 2017, 2017, 2715–2718 CrossRef CAS
;
(g) A. Matsuzawa, J. Shiraiwa, A. Kasamatsu and K. Sugita, Enantioselective, Protecting-Group-Free Total Synthesis of Boscartin F, Org. Lett., 2018, 20, 1031–1033 CrossRef CAS PubMed
.
- For 5-exo halo-cycloetherifications:
(a) S. H. Kang, S. B. Lee and C. M. Park, Catalytic Enantioselective Iodocyclization of γ-Hydroxy-cis-alkenes, J. Am. Chem. Soc., 2003, 125, 15748–15749 CrossRef CAS PubMed
;
(b) U. Hennecke, C. H. Müller and R. Fröhlich, Enantioselective Haloetherification by Asymmetric Opening of meso-Halonium Ions, Org. Lett., 2011, 13, 860–863 CrossRef CAS PubMed
;
(c) D. Huang, H. Wang, F. Xue, H. Guan, L. Li, X. Peng and Y. Shi, Enantioselective Bromocyclization of Olefins Catalyzed by Chiral Phosphoric Acid, Org. Lett., 2011, 13, 6350–6353 CrossRef CAS PubMed
;
(d) S. E. Denmark and M. T. Burk, Enantioselective Bromocycloetherification by Lewis Base/Chiral Brønsted Acid Cooperative Catalysis, Org. Lett., 2012, 14, 256–259 CrossRef CAS PubMed
;
(e) Y. Zhao, X. Jiang and Y.-Y. Yeung, Catalytic, Enantioselective, and Highly Chemoselective Bromocyclization of Olefinic Dicarbonyl Compounds, Angew. Chem., Int. Ed., 2013, 52, 8597–8601 CrossRef CAS PubMed
;
(f) Z. Ke, C. K. Tan, F. Chen and Y.-Y. Yeung, Catalytic Asymmetric Bromoetherification and Desymmetrization of Olefinic 1,3-Diols with C2-Symmetric Sulfides, J. Am. Chem. Soc., 2014, 136, 5627–5630 CrossRef CAS PubMed
;
(g) D. W. Tay, G. Y. C. Leung and Y.-Y. Yeung, Desymmetrization of Diolefinic Diols by Enantioselective Amino-thiocarbamate-Catalyzed Bromoetherification: Synthesis of Chiral Spirocycles, Angew. Chem., Int. Ed., 2014, 53, 5161–5164 CrossRef CAS PubMed
For 5-endo halo-cycloetherifications:
(h) X. Zeng, C. Miao, S. Wang, C. Xia and W. Sun, Asymmetric 5-endo Chloroetherification of Homoallylic Alcohols toward the Synthesis of Chiral β-chlorotetrahydrofurans, Chem. Commun., 2013, 49, 2418–2420 RSC
;
(i) Q. Wang, M. Lübcke, M. Biosca, M. Hedberg, L. Eriksson, F. Himo and K. J. Szabó, Enantioselective Construction of Tertiary Fluoride Stereocenters by Organocatalytic Fluorocyclization, J. Am. Chem. Soc., 2020, 142, 20048–20057 CrossRef CAS PubMed
;
(j) K. Yoshida, K. Okada, H. Ueda and H. Tokuyama, A Concise Enantioselective Total Synthesis of (−)-Deoxoapodine, Angew. Chem., Int. Ed., 2020, 59, 23089–23093 CrossRef CAS PubMed
For 6-exo halo-cycloetherification:
(k) P. Zhou, Y. Cai, X. Zhong, W. Luo, T. Kang, J. Li, X. Liu, L. Lin and X. Feng, Catalytic Asymmetric Intra- and Intermolecular Haloetherification of Enones: An Efficient Approach to (−)-Centrolobine, ACS Catal., 2016, 6, 7778–7783 CrossRef CAS
For 6-endo halo-cycloetherification:
(l) M. L. Landry, G. M. McKenna and N. Z. Burns, Enantioselective Synthesis of Azamerone, J. Am. Chem. Soc., 2019, 141, 2867–2871 CrossRef CAS PubMed
For 3-exo halo-cycloetherification:
(m) Z. Shen, X. Pan, Y. Lai, J. Hu, X. Wan, X. Li, H. Zhang and W. Xie, Chiral Ion-pair Organocatalyst Promotes Highly Enantioselective 3-exo Iodo-cycloetherification of Allyl Alcohols, Chem. Sci., 2015, 6, 6986–6990 RSC
.
-
(a) C. Enzell and H. Erdtman, The Chemistry of the Natural Order Cupressales—XXI: Cuparene and Cuparenic Acid, Two Sesquiterpenic Compounds with a New Carbon
Skeleton, Tetrahedron, 1958, 4, 361–368 CrossRef CAS
;
(b) Y. Asakawa, R. Matsuda, W. B. Schofield and S. R. Gradstein, Cuparane- and isocuparane-type sesquiterpenoids in liverworts of the genus Herbertus, Phytochemistry, 1982, 21, 2471–2473 CrossRef CAS
;
(c) H. Irita, T. Hashimoto, Y. Fukuyama and Y. Asakawa, Herbertane-type sesquiterpenoids from the liverwort Herbertus sakuraii, Phytochemistry, 2000, 55, 247–253 CrossRef CAS PubMed
.
- For selected recent examples:
(a) B. R. Aavula, Q. Cui and E. A. Mash, Synthesis of (S)-(-)-beta-Cuparenone and (S)-(-)-Cuparene, Tetrahedron: Asymmetry, 2000, 11, 4681–4686 CrossRef CAS
;
(b) T. Cohen, T. Kreethadumrongdat, X. J. Liu and V. Kulkarni, Use of Aromatic Radical-anions in the Absence of THF. Tandem Formation and Cyclization of Benzyllithiums Derived From the Attack of Homo- and Bishomoallyllithiums on alpha-Methylstyrenes: Two-pot Synthesis of Cuparene, J. Am. Chem. Soc., 2001, 123, 3478–3483 CrossRef CAS PubMed
;
(c) F. G. Favaloro, C. A. Goudreau, B. P. Mundy, T. Poon, S. V. Slobodzian and B. L. Jensen, Natural Products via Reetz Chemistry Synthesis of ( + /-)-Cuparene, Synth. Commun., 2001, 31, 1847–1855 CrossRef CAS
;
(d) R. S. Grainger and A. Patel, Photomediated Asymmetric Synthesis of (2)-Cuparene, Chem. Commun., 2003, 1072–1073 RSC
;
(e) A. Nayek, M. G. B. Drew and S. Ghosh, Convenient Route to Enantiopure aryl Cyclopentanes via Diels-Alder reaction of Asymmetric Dienes. Total synthesis of ( + )-Herbertene and ( + )-Cuparene, Tetrahedron, 2003, 59, 5175–5181 CrossRef CAS
;
(f) T. Paul, A. Pal and D. Mukherjee, Stereocontrolled total Synthesis of ( + /-)-Tochuinyl Acetate and Facile Total Synthesis of ( + /-)-alpha-Cuparenone and ( + /-)-Cuparene, ARKIVOC, 2003, 104–114 Search PubMed
;
(g) R. J. Boxall, L. Ferris and R. S. Grainger, Synthesis of C-13 Oxidised Cuparene and Herbertane Sesquiterpenes via a Paterno-Büchi Photocyclisation-Oxetane Fragmentation Strategy: Total Synthesis of 1,13-Herbertenediol, Synlett, 2004, 2379–2381 CAS
;
(h) F. Secci, A. Frongia, J. Ollivier and P. P. Piras, Convenient Formal Synthesis of ( + /-)-Cuparene, ( + /-)-Enokipodins A and B, and ( + /-)-Cuparene-1,4-quinone, Synthesis, 2007, 999–1002 CAS
;
(i) A. Srikrishna, G. Satyanarayana and K. R. Prasad, RCM-Based Approach to ( + /-)-Cuparene, Synth. Commun., 2007, 37, 1511–1516 CrossRef CAS
;
(j) M. R. Luderer, M. J. Mealy and W. F. Bailey, Asymmetric Intramolecular Carbolithiation of Achiral Substrates: Synthesis of Enantioenriched (R)-( + )-Cuparene and (R)-( + )-Herbertene, J. Org. Chem., 2014, 79, 10722–10726 CrossRef CAS PubMed
.
- X. Yu, J. Hu, Z. Shen, H. Zhang, J.-M. Gao and W. Xie, Stereospecific Construction of Contiguous Quaternary All-Carbon Centers by Oxidative Ring Contraction, Angew. Chem., Int. Ed., 2017, 56, 350–353 CrossRef CAS PubMed
.
- L. V. Alegrio, R. Braz-filho and O. R. Gottlieb, Diarylheptanoids and Isoflavonoids from Centrolobium Species, Phytochemistry, 1989, 28, 2359–2362 CrossRef CAS
.
- A.-M. Bui, B. C. Das and P. Potier, Étude Chimiotaxonomique de Hazunta Modesta, Phytochemistry, 1980, 19, 1473–1475 CrossRef CAS
.
-
(a) G. W. Gribble, Naturally Occurring Organohalogen Compounds, Acc. Chem. Res., 1998, 31, 141–152 CrossRef CAS
;
(b) F. H. Vaillancourt, E. Yeh, D. A. Vosburg, S. Garneau-Tsodikova and C. T. Walsh, Nature's Inventory of Halogenation Catalysts: Oxidative Strategies Predominate, Chem. Rev., 2006, 106, 3364–3378 CrossRef CAS PubMed
.
- J. Y. Cho, H. C. Kwon, P. G. Williams, P. R. Jensen and W. Fenical, Azamerone, a Terpenoid Phthalazinone from a Marine-Derived Bacterium Related to the Genus Streptomyces (Actinomycetales), Org. Lett., 2006, 8, 2471–2474 CrossRef CAS PubMed
.
- For 5-exo halolactonization:
(a) M. Wang, L. X. Gao, W. P. Mai, A. X. Xia, F. Wang and S. B. Zhang, Enantioselective Iodolactonization Catalyzed by Chiral Quaternary Ammonium Salts Derived from Cinchonidine, J. Org. Chem., 2004, 69, 2874–2876 CrossRef CAS PubMed
;
(b) Z. Ning, R. Jin, J. Ding and L. Gao, Enantioselective Iodolactonizations of 4-Pentenoic Acid Derivatives Mediated by Chiral Salen-Co(II) Complex, Synlett, 2009, 2291–2294 CAS
;
(c) D. C. Whitehead, R. Yousefi, A. Jaganathan and B. Borhan, An Organocatalytic Asymmetric Chlorolactonization, J. Am. Chem. Soc., 2010, 132, 3298–3300 CrossRef CAS PubMed
;
(d) L. Zhou, C. K. Tan, X. Jiang, F. Chen and Y.-Y. Yeung, Asymmetric Bromolactonization Using Amino-thiocarbamate Catalyst, J. Am. Chem. Soc., 2010, 132, 15474–15476 CrossRef CAS PubMed
;
(e) X. Jiang, C. K. Tan, L. Zhou and Y.-Y. Yeung, Enantioselective Bromolactonization Using an S-Alkyl Thiocarbamate Catalyst, Angew. Chem., Int. Ed., 2012, 51, 7771–7775 CrossRef CAS PubMed
;
(f) C. K. Tan, C. Le and Y.-Y. Yeung, Enantioselective Bromolactonization of cis-1,2-Disubstituted Olefinic Acids Using an Amino-Thiocarbamate Catalyst, Chem. Commun., 2012, 48, 5793–5795 RSC
;
(g) D. H. Paull, C. Fang, J. R. Donald, A. D. Pansick and S. F. Martin, Bifunctional Catalyst Promotes Highly Enantioselective Bromolactonizations To Generate Stereogenic C–Br Bonds, J. Am. Chem. Soc., 2012, 134, 11128–11131 CrossRef CAS PubMed
;
(h) W. Zhang, N. Liu, C. M. Schienebeck, K. Decloux, S. Zheng, J. B. Werness and W. Tang, Catalytic Enantioselective Halolactonization of Enynes and Alkenes, Chem. – Eur. J., 2012, 18, 7296–7305 CrossRef CAS PubMed
;
(i) X. Han, C. Dong and H.-B. Zhou, C3-Symmetric Cinchonine-Squaramide-Catalyzed Asymmetric Chlorolactonization of Styrene-Type Carboxylic Acids with 1,3-Dichloro-5,5-dimethylhydantoin: An Efficient Method to Chiral Isochroman-1-ones, Adv. Synth. Catal., 2014, 356, 1275–1280 CrossRef CAS
;
(j) H. Nakatsuji, Y. Sawamura, A. Sakakura and K. Ishihara, Cooperative Activation with Chiral Nucleophilic Catalysts and N-Haloimides: Enantioselective Iodolactonization of 4-Arylmethyl-4-pentenoic Acids, Angew. Chem., Int. Ed., 2014, 53, 6974–6977 CrossRef CAS PubMed
;
(k) H. Egami, J. Asada, K. Sato, D. Hashizume, Y. Kawato and Y. Hamashima, Asymmetric Fluorolactonization with a Bifunctional Hydroxyl Carboxylate Catalyst, J. Am. Chem. Soc., 2015, 137, 10132–10135 CrossRef CAS PubMed
;
(l) M. T. Knowe, M. W. Danneman, S. Sun, M. Pink and J. N. Johnston, Biomimetic Desymmetrization of a Carboxylic Acid, J. Am. Chem. Soc., 2018, 140, 1998–2001 CrossRef CAS PubMed
;
(m) T. Arai, K. Horigane, O. Watanabe, J. Kakino, N. Sugiyama, H. Makino, Y. Kamei, S. Yabe and M. Yamanaka, Association of Halogen Bonding and Hydrogen Bonding in Metal Acetate-Catalyzed Asymmetric Halolactonization, iScience, 2019, 12, 280–292 CrossRef CAS PubMed
;
(n) Y.-C. Chan, X. Wang, Y.-P. Lam, J. Wong, Y.-L. S. Tse and Y.-Y. Yeung, A Catalyst-Controlled Enantiodivergent Bromolactonization, J. Am. Chem. Soc., 2021, 143, 12745–11275 CrossRef CAS PubMed
For 6-exo halolactonization:
(o) K. Murai, T. Matsushita, A. Nakamura, S. Fukushima, M. Shimura and H. Fujioka, Asymmetric Bromolactonization Catalyzed by a C3-Symmetric Chiral Trisimidazoline, Angew. Chem., Int. Ed., 2010, 49, 9174–9177 CrossRef CAS PubMed
;
(p) G. E. Veitch and E. N. Jacobsen, Tertiary Aminourea-Catalyzed Enantioselective Iodolactonization, Angew. Chem., Int. Ed., 2010, 49, 7332–7335 CrossRef CAS PubMed
;
(q) M. C. Dobish and J. N. Johnston, Achiral Counterion Control of Enantioselectivity in a Brønsted Acid-Catalyzed Iodolactonization, J. Am. Chem. Soc., 2012, 134, 6068–6071 CrossRef CAS PubMed
;
(r) J. E. Tungen, J. M. J. Nolsøe and T. V. Hansen, Asymmetric Iodolactonization Utilizing Chiral Squaramides, Org. Lett., 2012, 14, 5884–5887 CrossRef CAS PubMed
For 6-endo halolactonization:
(s) C. K. Tan, L. Zhou and Y.-Y. Yeung, Aminothiocarbamate-Catalyzed Asymmetric Bromolactonization of 1,2-Disubstituted Olefinic Acids, Org. Lett., 2011, 13, 2738–2741 CrossRef CAS PubMed
;
(t) E. M. Woerly, S. M. Banik and E. N. Jacobsen, Enantioselective, Catalytic Fluorolactonization Reactions with a Nucleophilic Fluoride Source, J. Am. Chem. Soc., 2016, 138, 13858–13861 CrossRef CAS PubMed
For 4-exo halolactonization:
(u) K. Ikeuchi, S. Ido, S. Yoshimura, T. Asakawa, M. Inai, Y. Hamashima and T. Kan, Catalytic Desymmetrization of Cyclohexadienes by Asymmetric Bromolactonization, Org. Lett., 2012, 14, 6016–6019 CrossRef CAS PubMed
.
- M. Aursnes, J. E. Tungen and T. V. Hansen, Enantioselective Organocatalyzed Bromolactonizations: Applications in Natural Product Synthesis, J. Org. Chem., 2016, 81, 8287–8295 CrossRef CAS PubMed
.
-
(a) B. A. Bierl, M. Beroza and C. W. Collier, Potent Sex Attractant of the Gypsy Moth: Its Isolation, Identification, and Synthesis, Science, 1970, 170, 87–89 CrossRef CAS PubMed
;
(b) A. M. Liebhold and P. C. Tobin, Population Ecology of Insect Invasions and Their Management, Annu. Rev. Entomol., 2008, 53, 387–408 CrossRef CAS PubMed
.
- D. W. Klosowski and S. F. Martin, Synthesis of ( + )-Disparlure via Enantioselective Iodolactonization, Org. Lett., 2018, 20, 1269–1271 CrossRef CAS PubMed
.
- For review:
(a) A. Sakakura and K. Ishihara, Stereoselective Electrophilic Cyclization, Chem. Rec., 2015, 15, 728–742 CrossRef CAS PubMed
;
(b) A. C. A. D'Hollander, L. Peilleron, T. D. Grayfer and K. Cariou, Halonium-Induced Polyene Cyclizations, Synthesis, 2019, 51, 1753–1769 CrossRef
For selected examples:
(c) E. E. van Tamelen and E. J. Hessler, The Direct Brominative Cyclization of Methyl Farnesate, Chem. Commun., 1966, 411–413 RSC
;
(d) A. G. González, J. D. Martín, C. Pérez and M. A. Ramírez, Bromonium ion-induced Cyclization of Methyl Farnesate: Application to the Synthesis of Snyderol, Tetrahedron Lett., 1976, 17, 137–138 CrossRef
;
(e) L. E. Wolinsky and D. J. Faulkner, Biomimetic Approach to the Synthesis of Laurencia Metabolites. Synthesis of 10-bromo-alpha-Chamigrene, J. Org. Chem., 1976, 41, 597–600 CrossRef CAS
;
(f) Y. Yamaguchi, T. Uyehara and T. Kato, Biogenetic type synthesis of (±)-concinndiol and (±)-aplysin 20, Tetrahedron Lett., 1985, 26, 343–346 CrossRef CAS
;
(g) J. Barluenga, M. Trincado, E. Rubio and J. M. González, Intramolecular Arylation Reactions of Alkenes: A Flexible Approach to Chromans and Tetrahydroquinoline Derivatives, J. Am. Chem. Soc., 2004, 126, 3416–3417 CrossRef CAS PubMed
;
(h) S. A. Snyder and D. S. Treitler, Et2SBr·SbCl5Br: An Effective Reagent for Direct Bromonium-Induced Polyene Cyclizations, Angew. Chem., Int. Ed., 2009, 48, 7899–7903 CrossRef CAS PubMed
;
(i) S. A. Snyder, D. S. Treitler and A. P. Brucks, Simple Reagents for Direct Halonium-Induced Polyene Cyclizations, J. Am. Chem. Soc., 2010, 132, 14303–14314 CrossRef CAS PubMed
.
- T. Sakan, Y. Hayashi, Y. Honda, T. Shono, M. Nakajima and M. Kato, Structure and Stereochemistry of Boschniakine, Boschnialactone, and Boschnialinic acid, an Oxidation Product of Boschnialactone, Tetrahedron, 1967, 23, 4635–4652 CrossRef CAS
.
- T. Inoue, O. Kitagawa, A. Saito and T. Taguchi, Catalytic Asymmetric Iodocarbocyclization Reaction of 4-Alkenylmalonates and Its Application to Enantiotopic Group Selective Reaction, J. Org. Chem., 1997, 62, 7384–7389 CrossRef CAS PubMed
.
-
(a) A. Sakakura, A. Ukai and K. Ishihara, Enantioselective Halocyclization Of Polyprenoids Induced By Nucleophilic Phosphoramidites, Nature, 2007, 445, 900–903 CrossRef CAS PubMed
;
(b) Y. Sawamura, Y. Ogura, H. Nakatsuji, A. Sakakura and K. Ishihara, Enantioselective Bromocyclization of 2-Geranylphenols induced by Chiral Phosphite–urea Bifunctional Catalysts, Chem. Commun., 2016, 52, 6068–6071 RSC
.
- R. C. Samanta and H. Yamamoto, Catalytic Asymmetric Bromocyclization of Polyenes, J. Am. Chem. Soc., 2017, 139, 1460–1463 CrossRef CAS PubMed
.
- Q. Cao, J. Luo and X. Zhao, Chiral Sulfide Catalysis for Desymmetrizing Enantioselective Chlorination, Angew. Chem., Int. Ed., 2019, 58, 1315–1319 CrossRef CAS PubMed
.
- J. Luo, Y. Zhang, F. Zhong and X. Zhao, Catalytic Enantioselective Construction of Chiral Benzo-Fused N-Heterocycles through Friedel–Crafts-Type Electrophilic Chlorination, CCS Chem., 2021 DOI:10.31635/ccschem.021.202100777
.
|
This journal is © the Partner Organisations 2022 |