DOI:
10.1039/D1QM01278A
(Research Article)
Mater. Chem. Front., 2022,
6, 103-116
Cu ions and cetyltrimethylammonium bromide loaded into montmorillonite: a synergistic antibacterial system for bone scaffolds
Received
17th September 2021
, Accepted 15th November 2021
First published on 19th November 2021
Abstract
Developing a novel antibacterial material with highly effective and long-lasting antibacterial activity to prevent bacterial infection during or post bone scaffold transplantation is an urgent need for bone regeneration. In this study, a synergistic antibacterial system for a polyglycolic acid (PGA) scaffold was constructed by cation exchange of montmorillonite (MMT) with Cu2+ and then intercalation of cetyltrimethylammonium bromide (CTAB) into the interlayer of MMT. On one hand, the introduced Cu2+ enlarged the interlayer spacing of MMT, facilitating the intercalation of CTAB into the MMT interlayer to impart the antibacterial effect. On the other hand, the intercalation of CTAB into MMT shifted the surface potential of MMT from negative to positive, improving the bacterial adsorption capacity by electrostatic forces so that Cu2+ could act directly on the negatively charged bacteria. As a result, the bacterial adsorption rate of Cu2+ and CTAB modified MMT (Cu-CMMT) improved by 76.5% compared with that of MMT, which could be explained by the increased zeta potential from −26.1 to 19.7 mV. Besides, the scaffold with Cu-CMMT exhibited robust antibacterial activities against E. coli with an antibacterial rate of 98.1%, which was significantly higher than that of the scaffold with Cu2+ or CTAB modified MMT individually. The scaffold with Cu-CMMT exhibited the highest level of reactive oxygen species (ROS), which was attributed to the dilapidation of bacterial cells.
1. Introduction
Bacterial infection during or post scaffold transplantation remains a clinical challenge as it might cause the failure of bone defect repair.1 A conventional method to treat bacterial infection is antibiotic therapy, which is becoming less efficient due to the increased antibiotic resistance. Recently, copper related materials have received extensive attraction because of their wide potential applications such as in hydrogen production, solar energy, catalysis, and anode materials for lithium ion batteries.2,3 Among these, copper compounds such as CuCl2 and CuSO4 have been recognized as effective antibacterial agents with an excellent antibacterial efficacy, broad bactericidal spectrum and no bacterial resistance on account of the release of Cu2+ playing a role in killing bacteria.4,5 Bai et al. fabricated a Cu2+ doped calcium alginate hydrogel filtration membrane using CuCl2 and demonstrated an excellent antibacterial ability.6 Zhu et al. used CuSO4 to trigger polydopamine and poly-(sulfobetaine methacrylate) co-deposition which endowed polyurethane with great antibacterial properties.7 However, a high initial burst release of Cu2+ will cause local or systemic toxicity and short-term antibacterial efficacy.8,9 As a consequence, it is of great significance to achieve a slow and sustained release of Cu2+ during the antibacterial process.
The loading of antibacterial materials into a carrier is a commonly used method for controlling the release of antibacterial agents.10–12 Montmorillonite (MMT) is a popular candidate for loading antibacterial materials due to its high cation exchange capacity, large specific surface area, and high platelet aspect ratio.13,14 Recently, the synthesis and application of MMT-based antibacterial materials have attracted great attention due to the unique structure of MMT. Some researchers have reported the application of modifying MMT with chitosan and chlorhexidine acetate to obtain antibacterial materials.15 It is layered silicate clay with a unique interlayer structure, and the interlayer galleries of MMT are mainly filled with exchangeable positively charged ions such as Na+, Ca2+, etc., so Cu2+ can be introduced into the MMT interlayer through a cation exchange process. More importantly, by introducing Cu2+ into the interlayer, Cu2+ is limited by the interlayer of MMT, and the sheets of MMT act as a barrier to reduce the release of Cu2+. For example, Tong et al. prepared an inorganic antibacterial agent of Cu-exchanged MMT by cation exchange, and they found that Cu2+ releasing from Cu-exchanged MMT maintained a stable ionic concentration for a long time in the solution.16 The results indicated that the loading of Cu2+ into MMT could play a long-term and effective antibacterial role, benefiting from the function of MMT as a barrier to reduce the burst release of Cu2+.
The combination of inorganic metal ions and organic quaternary ammonium salt can engender an obvious synergistic effect on antibiosis.17 For example, Harrison et al. combined Cu2+ and a quaternary ammonium salt of benzalkonium chloride together as an antibacterial agent, and they found out that Cu2+ and benzalkonium chloride exhibited a synergistic effect against Pseudomonas aeruginosa, whose minimum concentration for killing 50% of the tested bacteria showed a 128-fold decrease compared to that of a single-agent treatment.18 Cetyltrimethylammonium bromide (CTAB) is a popular quaternary ammonium salt which is widely used as a surfactant. Furthermore, several research studies have shown that CTAB can act as an organic antimicrobial agent to kill bacteria by disrupting the cell membrane and generating reactive oxygen species.19 For example, Chen et al. indicated that CTAB-loaded magnetic nanospheres have great potential application for rapid capturing and killing of bacteria from the contaminated source.20 Therefore, it is herein hypothesized to combine CTAB with Cu2+ loaded MMT to achieve a synergistic antibacterial activity. On one hand, the introduction of Cu2+ by cation exchange might enlarge the interlayer spacing of MMT, facilitating the intercalation of more CTAB into MMT to play the antibacterial role. On the other hand, the intercalation of CTAB into MMT might shift the surface charge of MMT in suspension from negative to positive, adsorbing more bacteria on the surface for Cu2+ to kill them.
In this work, Cu2+ was firstly introduced into the interlayer of MMT via cation exchange followed by the intercalation of CTAB to construct a synergistic antibacterial system for a polyglycolic acid (PGA) bone scaffold fabricated via selective laser sintering (SLS). The surface potential, chemical composition and surface morphologies of MMT before and after Cu2+ and CTAB modification were tested. Moreover, the antibacterial properties of the scaffold were investigated against Escherichia coli (E. coli) by bacterial morphological observation, an inhibition zone test and soaking method. Based on the experimental results, a possible synergistic antibacterial mechanism of Cu2+ and CTAB was discussed using an intracellular reactive oxygen species (ROS) level assay. In addition, compressive properties were determined by compressive tests and the corresponding reinforcement mechanism was discussed.
2. Results and discussion
A schematic to illustrate the modification of MMT for obtaining Cu-MMT, CMMT and Cu-CMMT powders was shown in Fig. 1(a). The FTIR spectra and XRD patterns of those powders are displayed in Fig. 1(b) and (c), respectively. For the results of FTIR spectra, the characteristic peaks for MMT were present at 3624 and 3427 cm−1, which were caused by O-H stretching vibrations of MMT.21,22 For CMMT and Cu-CMMT there appeared two new peaks at 2853 and 2924 cm−1 after the intercalation of CTAB due to CH2 and CH3 stretching vibrations, respectively.23,24 This could be explained by the fact that CTAB introduced alkyl chains in the MMT interlayer. There was no obvious difference between Cu-MMT and MMT, which indicated that the ion exchange of Cu2+ did not bring new functional groups. Peaks in the XRD patterns of MMT, Cu-MMT, CMMT and Cu-CMMT powders reflected the basal spacing according to Bragg's equation (2d
sin
θ = nλ)25. MMT with a peak at 2θ = 7.15° featured a basal spacing of 1.23 nm, and the peak of Cu-MMT shifted to 6.48°, indicating a larger spacing (1.36 nm) than that of MMT. In addition, CMMT and Cu-CMMT with peaks at 6.14° and 5.22° possessed basal spacings of 1.44 nm and 1.69 nm, which were higher than that of Cu-MMT, respectively. The reason might be that CTAB intercalated in the MMT layers had long alkyl chains to better extend the d-spacing of MMT than Cu2+ existing in the interlayer spacing.
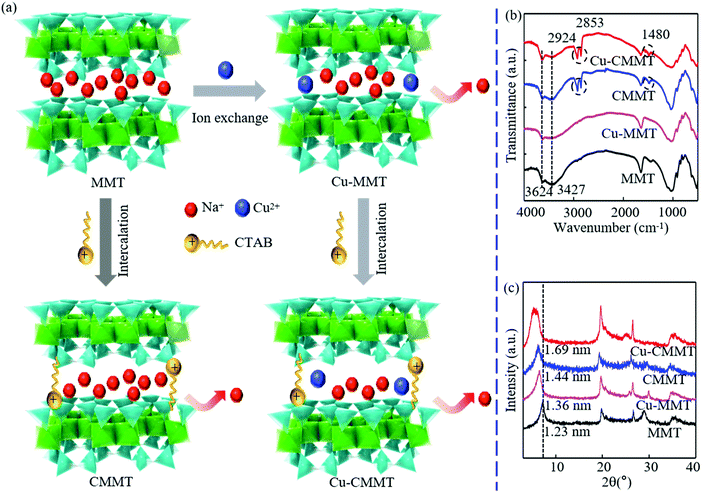 |
| Fig. 1 (a) Mechanism diagram of modifying MMT to obtain Cu-MMT, CMMT and Cu-CMMT powder; (b) FTIR spectrum; (c) XRD patterns. | |
Surface morphologies of MMT, Cu-MMT, CMMT and Cu-CMMT powders were observed using SEM (Fig. 2(a–d)), and the corresponding EDS mapping images as well as EDX spectra (Fig. 2(a1–d1)) are shown below the SEM images. It was observed that MMT sheets were predominantly between 10 and 20 μm in diameter with some bending and curling, and there were some aggregations.26,27 Comparing the four types of powders, their morphologies were almost the same, which indicated modification did not change the morphologies of the MMT powder. EDS mapping and EDX data further analyzed the element composition. The fundamental elements of MMT were Si, Na, O, Mg and Al. As a result of cation exchange and intercalation, Cu-MMT showed the appearance of Cu element and CMMT showed the appearance of N element, respectively. In addition, Cu-CMMT included both Cu and N elements from the results. What's more, it could be observed that the mapping intensity of Na element was lower after modification, indicating the decreasing content of Na+ in the MMT layer. This could be explained by cation exchange of Na+ during the modification process. EDX spectra also supported the presence of Cu2+ and CTAB in the interlayer spacing of MMT after modification.
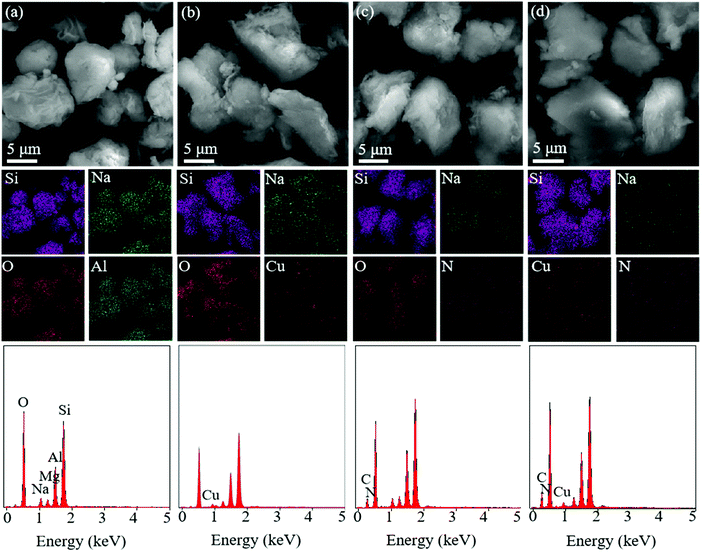 |
| Fig. 2 (a–d) SEM images, the corresponding EDS mapping and (a1–d1) EDX spectra of (a and a1) MMT, (b and b1) Cu-MMT, (c and c1) CMMT and (d and d1) Cu-CMMT powder. | |
Zeta potential was a major parameter influencing the bacterial adsorption ability of the powders, and the results are displayed in Fig. 3(a and b).28,29 Classical negative surface charge of −26.1 mV was observed for MMT, and the surface charge increased after exchange with Cu. The increased zeta potential of Cu-MMT (−1.3 mV) can be explained by the positively charged cations adsorbed on the surface of MMT under the action of electrostatic charge, which could neutralize some negative charges.30,31 With the introduction of CTAB, the zeta potential of MMT transformed from negative to positive, which was 14.2 mV for CMMT. This was because the loading of CTAB enabled a large amount of NH4+ to adsorb onto MMT, leading to a surplus of positive charge on the MMT surface. The highest zeta potential of 19.7 mV was observed in Cu-CMMT, and thus the adhesion with negatively charged bacteria could be obviously improved.
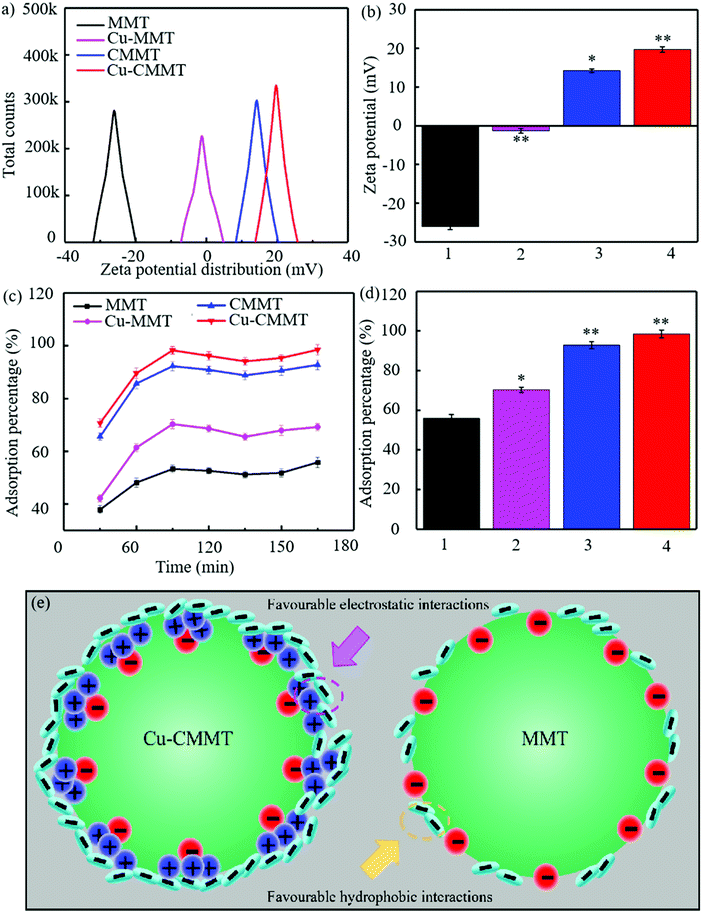 |
| Fig. 3 (a) Zeta potential distribution of MMT, Cu-MMT, CMMT and Cu-CMMT powder; (b) zeta potential; (c) effect of time on the adsorption of E. coli onto powder; (d) adsorption percentage; (e) mechanism diagram of adsorption between E. coli and powder. | |
To assess the adsorption effect between bacteria and powders, adsorption experiments were carried out (Fig. 3(c and d)). It could be found that the adsorption rate had the tendency to increase from 0 to 90 min, decline from 90 to 150 min and increase again thereafter until 210 min. For MMT, the adsorption rate of E. coli at 30 min was 37.9%, and the highest adsorption rate was 55.8% at 210 min. Cu-MMT possessed a higher adsorption rate than MMT, whose adsorption rate was ranging from 42.2% to 70.3%, indicating there were more E. coli adsorbed on it. As for CMMT, the lowest and highest adsorption rate was 65.6% and 92.8%, respectively. However, Cu-CMMT presented excellent absorption capability whose adsorption rate reached 98.5% after contacting with E. coli for 210 min. This was because of the large specific surface area of clay as well as the huge potential difference between Cu-CMMT and E. coli. The schematic is shown in Fig. 3(e) to compare the adsorption mechanism between MMT and Cu-CMMT. As described above, the loading of positively charged NH4+ of CTAB on Cu-MMT changed the zeta potential from negative into positive, thus introducing favorable charged adsorption sites to the MMT surface, which could attract negatively-charged bacteria through electrostatic and hydrophobic interaction. At the same time, the specific surface area and the absorption rate of composite materials increased and enhanced the adsorption ability of bacteria as demonstrated by some studies. For instance, Liu et al. investigated and determined the improved adsorption capacity of E. coli on the surface cetyltributylphosphonium bromide modified MMT.32 MMT without modification was capable of adsorbing bacteria through hydrophobic interactions between the methyl/benzene groups and siloxane surface.
To evaluate the mechanical properties of the scaffolds, a compression test was carried out, and the specimen before and after the test were photographed. Before compression, the height of the PGA specimen was about 10 mm, and the height reduced to 6 mm under the force as shown in Fig. 4(a). Compression strength and modulus of the PGA specimen were 26.61 MPa and 2.37 GPa, respectively. Adding 10% MMT into the PGA matrix had a reinforcing effect on the mechanical properties, whose strength and modulus were 38.12 MPa and 3.62 GPa, respectively.33,34 Cu-MMT had almost the same effect as MMT, and the strength and modulus of the PGA/Cu-MMT specimen only enhanced 2.32 MPa and 0.13 GPa compared to the PGA/MMT specimen, respectively. CMMT was much more effective at improving the mechanical properties of PGA than MMT, and the PGA/CMMT specimen exhibited a high strength and modulus of 45.60 MPa and 4.25 GPa, respectively. This could be explained by the fact that CMMT had better compatibility with the PGA matrix than MMT since the long organic tail of CTAB intercalated in CMMT could adsorb with the PGA chain, as shown in Fig. 4(c).35,36 It is necessary to organically modify MMT with cationic–organic surfactants in order to improve the compatibility of MMT with an organic monomer or polymers. A previous study of Xiong et al. indicated that the mechanical properties of polyurethane/MMT nanocomposites dramatically increased by about 600% for methylene-bis-ortho-chloroaniline as a novel reactive modifier to the compatibility between MMT and polymer.37 The strength and modulus increased by 77.4% and 86.5% than PGA for the PGA/Cu-CMMT specimen (47.2 MPa, 4.42 GPa), respectively.
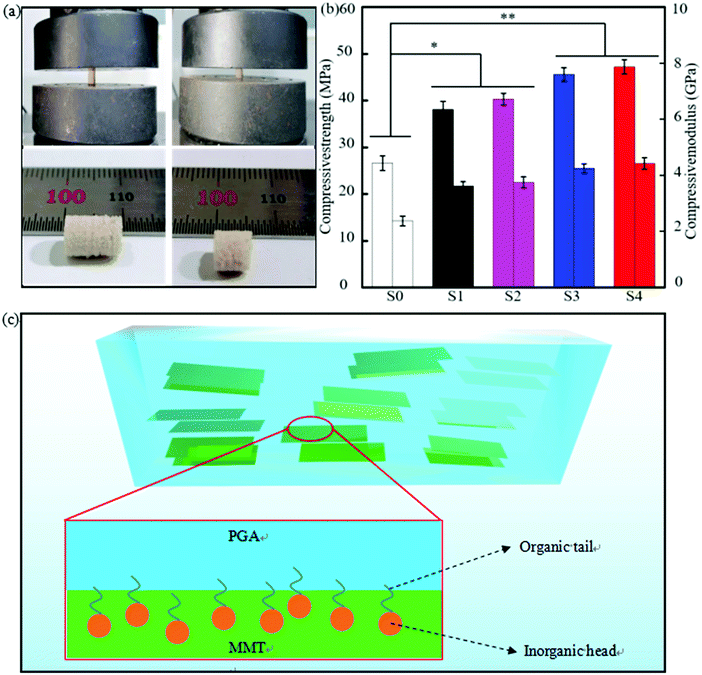 |
| Fig. 4 (a) Picture of the specimens before and after the compressive test; (b) compressive strength and modulus of the specimens; (c) mechanism diagram to illustrate the role of CTAB to improve the compatibility of PGA and MMT. | |
Representative photographs of WCA were shown to visualize the wettability (Fig. 5(a)), and the values are represented in a bar graph (Fig. 5(b)). The WCA of the PGA/MMT scaffold was 56.7°, which indicated the hydrophilicity of the materials.38 The WCA of the PGA/Cu-MMT scaffold (54.3°) was slightly lower than the PGA/MMT scaffold. As reported by other studies, the surface wettability behaviors were closely related to the exchangeable cations in MMT, and so, the wettability of MMT enhanced after cation exchange between Na+ and Cu2+.39 However, the WCA of the PGA/CMMT scaffold increased 4.7° compared to the PGA/MMT scaffold. This was due to the fact that hydrophilic MMT could be organically modified by intercalation of alkyl chains to make it more hydrophobic from previous studies.40 Many research studies investigated the modification of MMT to change the hydrophobicity of organoclays. For instance, Shah et al. demonstrated that the length of the alkyl chain and the functional groups on quaternary ammonium ions of the modifier can affect the hydrophobicity of MMT, which was prepared by modifying MMT with quaternary ammonium salts having different alkyl chain lengths and a benzyl substitute group41. As a result, the water contact angle of the PGA/Cu-CMMT scaffold (71.1°) was the largest with the most CTAB chains intercalated to modify MMT. The degradation properties were evaluated by PBS immersion, and the morphologies of the specimen surface were shown in Fig. 5(c). After 4 weeks immersion, there were numerous small holes on the PGA/MMT surface since MMT and PGA are water-absorbing materials allowing the penetration of water in the scaffold (Fig. 5(c1)). The holes existing in the PGA/Cu-MMT specimen were the most due to the best wettability, as shown in Fig. 5(c2), which seemed to complete the degradation of part of the surface material.42 The holes became less and smaller for the PGA/CMMT specimen (Fig. 5(c3)), and there was a large part of undegraded area displayed on the PGA/Cu-CMMT surface (Fig. 5(c4)). The mass loss of the specimens for different immersion periods (7, 14, 21 and 28 days) is presented in Fig. 5(d) to quantitatively analyze the degradation behavior. The mass loss of specimens gradually increased with immersion time, and the mass loss ratio of the PGA/MMT specimen increased from 4.3% (7 days) to 18.1% (28 days). There was only a small difference in mass loss among the scaffolds after immersion for 7 days which fluctuated at 4.0%. However, the mass loss of the PGA/Cu-MMT, PGA/CMMT and PGA/Cu-CMMT scaffolds reached 18.6%, 17.3% and 16.6% after immersion for 28 days, respectively.
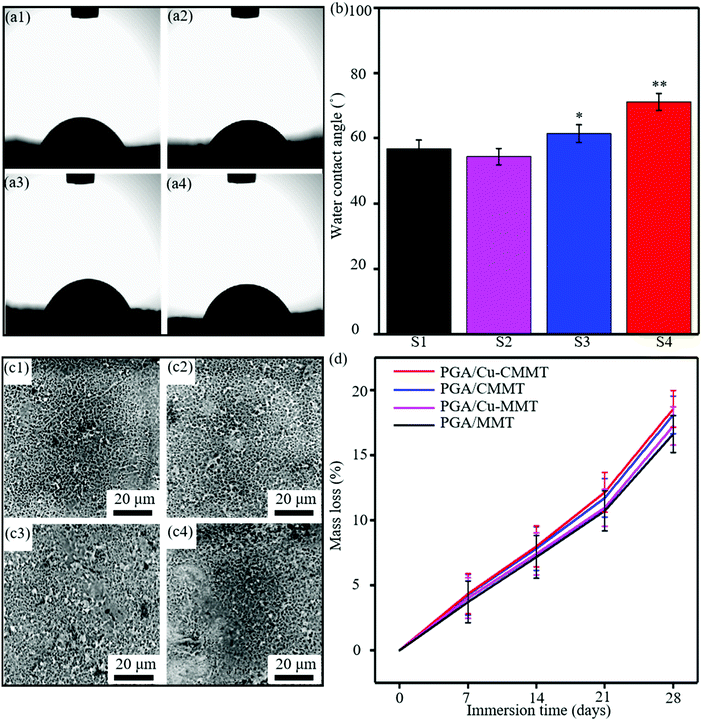 |
| Fig. 5 (a1–a4) Pictures of (a1) PGA/MMT, (a2) PGA/Cu-MMT, (a3) PGA/CMMT and (a4) PGA/Cu-CMMT scaffolds in the water contact angle test; (b) the water contact angle of scaffolds; (c1–c4) degradation morphologies of (c1) PGA/MMT, (c2) PGA/Cu-MMT, (c3) PGA/CMMT and (c4) PGA/Cu-CMMT scaffolds after immersion for 28 days; (d) mass loss of scaffolds for different periods of immersion. | |
The synergistic effect of Cu2+ and CTAB on the antibacterial activity of the scaffold could be observed by antibacterial tests including the turbidimetric test, bacterial inhibition rate, inhibition zone test and bacterial adhesion morphologies. The photograph of the blank control, negative control, and E. coli suspensions after incubating with scaffolds for 24 h is displayed in Fig. 6(a). The turbidity of the E. coli suspensions with the PGA/MMT scaffold is close to the negative control, which indicates a low antibacterial rate.43,44 The turbidity of the E. coli suspensions with the PGA/Cu-MMT, PGA/CMMT and PGA/Cu-CMMT scaffolds was higher than the blank control, showing an obvious antibacterial activity. To qualitatively analyze the antibacterial effect, the antibacterial rate was calculated according to the absorbance of the bacterial suspension (Fig. 6(b)). Corresponding to the results of turbidity, the lowest antibacterial rate was 16.2% for the E. coli suspensions with the PGA/MMT scaffold. The antibacterial rates of other E. coli suspensions with scaffolds were all over 75%, and the highest was obtained in the E. coli suspensions with the PGA/Cu-CMMT scaffold (98.1%), indicating that most of the bacteria were killed by Cu2+ and CTAB.45,46 In addition, the PGA/Cu-MMT scaffold displayed a better antibacterial effect (90.4%) than the PGA/CMMT scaffold (77.8%) due to the larger reactive oxygen species (ROS) production of Cu2+.
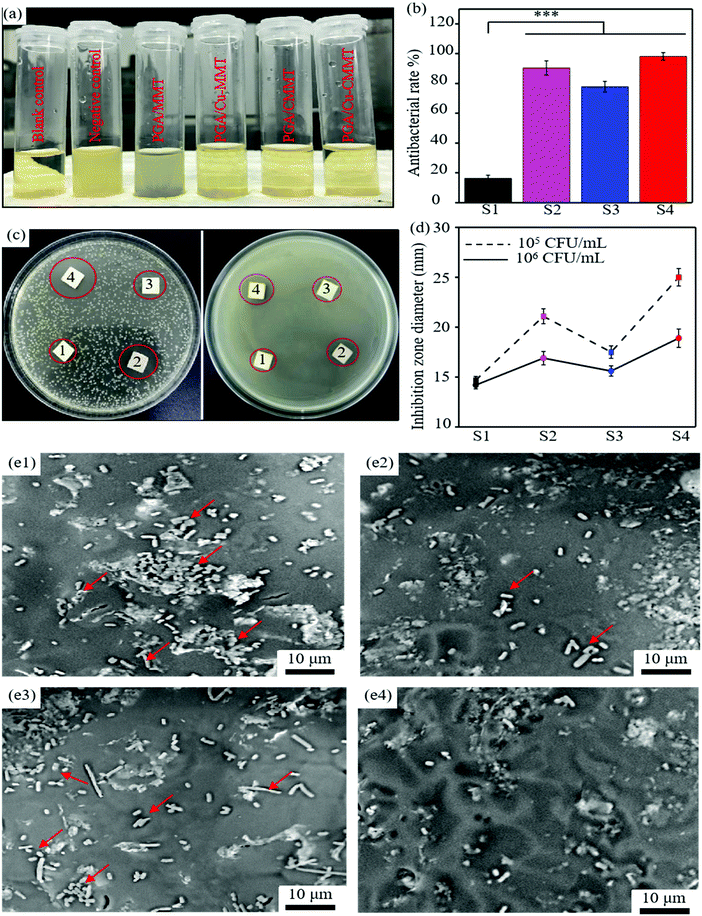 |
| Fig. 6 (a) Photographs of turbidities; (b) antibacterial rate; (c) inhibition zone picture of scaffolds; (d) diameter of inhibition zone; (e1–e4) adhesion morphologies of E. coli on (e1) PGA/MMT, (e2) PGA/Cu-MMT, (e3) PGA/CMMT and (e4) PGA/Cu-CMMT scaffolds. | |
An inhibition zone test of E. coli suspensions mixed with agar medium at different densities (1 × 105 CFU mL−1 and 1 × 106 CFU mL−1) was performed. Photographs of the disks are shown in Fig. 6(c), and the diameter of the inhibition zones from the disks is shown in Fig. 6(d). From the results, there were obvious inhibition zones around all the scaffolds except for the PGA/MMT scaffold. It is known that the more obvious the antibacterial effect was, the larger the diameter of the inhibition zones would be.47 By comparing the two media, it was observed that the diameter of the inhibition zones with an E. coli suspension density of 1 × 105 CFU mL−1 was larger than that of 1 × 106 CFU mL−1. Moreover, the PGA/Cu-CMMT scaffold exhibited a higher inhibition zone diameter (25.0, 18.9 mm) than both the PGA/Cu-MMT (21.1, 16.9 mm) and PGA/CMMT scaffolds (17.5, 15.6 mm). The results suggested that although increasing the E. coli suspension density weakened the antibacterial effect, the PGA/Cu-CMMT scaffold still exhibited a good synergistic antibacterial activity.48,49 Bacterial morphologies were observed by SEM as shown in Fig. 6(e1–e4). A large amount of rod-like E. coli (pointed by red arrows) was adhered on the surface of the PGA/MMT scaffold (Fig. 6(e1)). The number of E. coli adhered on the surface declined for the PGA/Cu-MMT (Fig. 6(e2)) and PGA/CMMT scaffolds (Fig. 6(e3)) compared to the PGA/MMT scaffold. Interestingly, there were only a few E. coli on the PGA/Cu-MMT scaffold in Fig. 6(e4), indicating the strong antibacterial effect suppressing bacterial adhesion and destroying the bacterial structure.50
To understand the mechanism of antibacterial activity, the generation of intracellular ROS was detected based on the fact that DCFH could be oxidized to generate 20,70-dichlorofuorescein (DCF) with green fluorescence with the help of ROS.51 The fluorescence images of bacteria treated with the PGA/MMT scaffolds (Fig. 7(a1)) showed almost no green color, indicating that MMT did not cause a ROS response. The fluorescence images of bacteria treated with the PGA/CMMT scaffolds (Fig. 7(a2)) exhibited weak green fluorescence, and the green fluorescence was increased for the PGA/Cu-CMMT scaffolds in Fig. 7(a3). Obviously, the most green fluorescence existed in the bacterial treated with the PGA/Cu-CMMT scaffolds (Fig. 7(a4)), which suggested a significant ROS level.52 The fluorescent signal intensity of DCF was quantitatively analyzed by a fluorescence spectrophotometer, whose result is shown in Fig. 7(b). The ROS level was proportional with fluorescence intensity, and it could be seen that the fluorescence intensity increased as the antibacterial activity in Fig. 6 increased. Therefore, it could be concluded that the ROS level was correlated with the antibacterial activity of the scaffold. To further verify the types of ROS, ESR signals were detected by a spectrometer. As shown in Fig. 7(c), the ESR spectrum of PGA/Cu-CMMT containing DMPO and H2O2 was a typical DMPO-OH spin adduct spectrum in water solutions. Besides, typical ESR signals of ˙O2− induced by PGA/Cu-CMMT can be seen in Fig. 7(d). These results revealed the ROS produced by the PGA/Cu-CMMT scaffold which was the most according to the above DCFH-DA staining method was ˙OH and ˙O2−.53
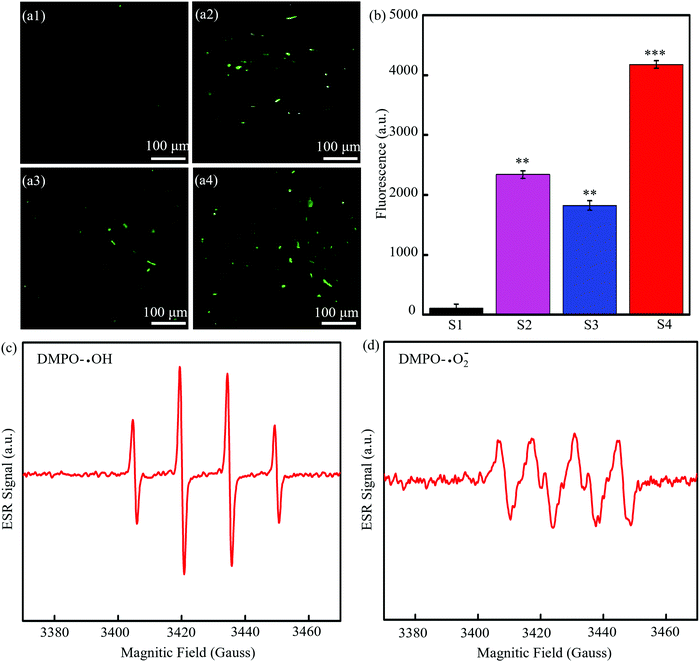 |
| Fig. 7 (a1–a4) Fluorescent microscopic images of the E. coli stained with DCFH-DA after incubating with (a1) PGA/MMT, (a2) PGA/Cu-MMT, (a3) PGA/CMMT and (a4) PGA/Cu-CMMT scaffolds for 2 h respectively; (b) the ROS levels of the E. coli represented by the fluorescence intensity of DCF. (c) DMPO spin-trapping ESR spectra recorded for ˙O2− in PGA/Cu-CMMT; (d) DMPO spin-trapping ESR spectra recorded for ˙OH in PGA/Cu-CMMT. | |
It might be inferred that the antibacterial activity was mainly focused on ROS formation, and the possible synergistic antibacterial mechanism of Cu-CMMT against E. coli was depicted in Fig. 8. As described before, Cu-CMMT possessed high bacterial adsorption ability due to the positive surface potential. Moreover, the release of Cu2+ and CTAB both possessed an antibacterial effect against E. coli. In detail, bacteria can be attacked by the penetration of Cu2+ and CTAB into the cell via the cell membrane. Some studies reported that Cu2+ as transition metal ions could induce a Fenton reaction to generate ROS and CTAB as a cationic surfactant and could also play an antibacterial role to induce superoxide stress and ROS in bacteria.54,55 As a result, the intrusive Cu2+ and CTAB induced the formation of ROS to kill the E. coli. From previous studies, a high ROS level leads to increased oxidative stress in cells, which can damage the cell membrane, DNA and the proteins.56,57 The damage of the cell membrane increased the cell membrane permeability and accelerated the disruption in membrane integrity, leading to the leakage of cell content.
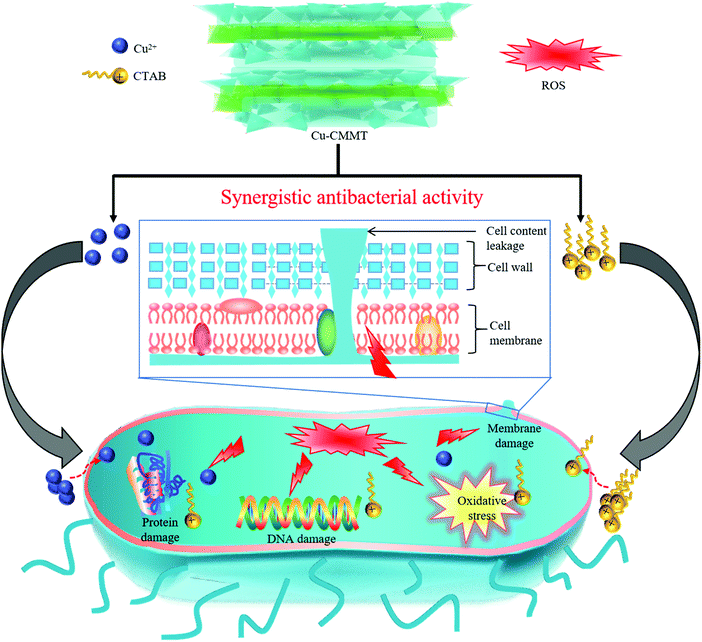 |
| Fig. 8 Schematic to illustrate the synergistic antibacterial activity of Cu2+ and CTAB towards E. coli. | |
3. Experimental
3.1. Materials
PGA was supplied by Shenzhen Polymtek Biomaterial Co., Ltd, China. MMT was obtained from Feiyu Biological Technology Co., Ltd, China. CTAB was purchased from Clamar Co., Ltd, China. CuSO4 was provided by Aladdin Co., Ltd, China.
3.2. Preparation of modified MMT
Cu2+ modified MMT (Cu-MMT) was prepared through a cation-exchange process. Firstly, MMT (5.0 g) was dispersed in 50 mL distilled water by vigorous stirring for 12 h at room temperature to obtain a stable suspended solution. Afterwards, CuSO4 (5.0 mmol) was added to the suspended solution and the mixed solution was stirred vigorously at 65 °C for 5 h. The mixed solution was filtered and washed repeatedly using distilled water three times. At last, the collected powder was dried in an oven at 80 °C for 8 h. CTAB modified MMT (CMMT) was prepared through an intercalation method similar to precious modification. Firstly, MMT (5.0 g) was dispersed in 50 mL distilled water with vigorous mechanical stirring for 12 h at room temperature. Afterwards, CTAB (2.5 mmol) was added to the suspended solution of MMT, and the mixed solution was stirred vigorously at 65 °C for 5 h. The mixed solution was filtered and washed using distilled water three times. Finally, the collected powder was dried in an oven at 80 °C for 8 h. The preparation of CTAB modified Cu-MMT (Cu-CMMT) was consistent with the above methods but replace MMT with Cu-MMT.
3.3. Preparation of the composite scaffold
PGA-based composite powders with 10 wt% MMT and modified MMT were prepared, taking PGA/Cu-CMMT for example: (a) putting 4.5 g PGA into a beaker with anhydrous ethanol under ultrasonic dispersion for 0.5 h; (b) putting 0.5 g Cu-CMMT into a beaker with anhydrous ethanol under ultrasonic dispersion for 0.5 h; (c) mixing MMT suspension and PGA suspension together, and then magnetically stirring the mixed suspension for 1 h; (d) dispersing the mixed suspension under ultrasonic dispersion for 1 h; (e) filtering the suspension to obtain PGA/Cu-CMMT precipitate, and then drying the precipitate at 80 °C. Four types of PGA-based composite powders were prepared using MMT, Cu-MMT, CMMT and Cu-CMMT, which were named PGA/MMT, PGA/Cu-MMT, PGA/CMMT and PGA/Cu-CMMT, respectively. Scaffolds were prepared via SLS using the above composite powders.58,59
3.4. Microstructure and mechanical properties
The phase composition of MMT and modified MMT powders was determined using an X-ray diffractometer (XRD) at 4° min−1 using Ni-filtered Cu-Kα radiation (λ = 0.154056 nm). The functional group of MMT and modified MMT powders was characterized by Fourier Transform Infrared Spectroscopy (FTIR). Surface morphology and element composition of the powders and scaffolds were characterized using a scanning electron microscope (SEM) equipped with energy disperse spectroscopy (EDS). Compressive tests were conducted according to the American Society for Testing and Materials International (ASTM) standards D695 Type 2a.60,61 An Instron universal material testing system (model 5567) was used to perform the testing at room temperature. Compressive specimens (with the size 5 mm × 5 mm × 10 mm) were compressed between two steel plates at the rate of 0.5 mm min−1, and the load was applied until the specimen was compressed to approximately 50% of its original length.62,63 Compressive strength and modulus were determined from stress–strain curves, and the values of strength and modulus were the average results of five tests.
3.5. Wettability and degradable properties
A water contact angle (WCA) test was carried out using an Attension Theta Lite optical tensiometer. During the test, a droplet of 4 μL deionized water was slowly dripped on the surface of the specimens, and the process was recorded by video. Degradable properties were tested by immersing specimens in phosphate buffered saline (PBS). Briefly speaking, specimens were weighed before immersing, and after that they were placed into a tube with PBS respectively. And then, specimens in tubes were shaken constantly and incubated in water at 37 °C. After incubation for 28 days, specimens were taken out from the tubes and placed in a dried oven. After drying, the extracted specimens were weighed to calculate the weight loss according to the following equation:
Weight loss (%) = (W0 − W1)/W0 × 100 |
where W0 represents the initial weight and W1 represents the weight after immersion for 28 days. Degradation morphologies after 28 days immersion were further evaluated under SEM.
3.6. Bacterial adsorption experiments
Adsorption percentage of bacterial on the specimens was conducted as a function of time. Before the experiment, E. coli was cultured in a log phase at 37 °C, and the weight of total bacteria (W1) was measured after centrifugation. And then, specimens were added to the suspension of bacterial, and the mixture was shaken in an orbital shaker at 150 rmp min−1 for different time periods (30, 60, 90, 120 and 150 min). Afterwards, 1.5 mL sucrose (60 wt%) solution was injected into the bottom of the suspension. As sucrose had a greater density than the bacteria suspended in solution, but was less dense than the specimens, the specimens with adsorbed bacteria sunk to the bottom of the tube while un-adsorbed bacteria as well as the aqueous solution would remain in the sucrose layer. And so the un-adsorbed bacterial part was extracted using a pipette. Next, the weight of un-adsorbed bacteria (W2) was measured after centrifugation. At last, adsorption percentage was calculated using the following formula:64
Adsorption percentage (%) = W1 − W2/W1 × 100 |
3.7. Antibacterial properties
Antibacterial properties of the scaffolds were evaluated using an inhibition zone method, turbidimetry method and bacterial morphology characterization. Specimens were sterilized by ultraviolet light exposure before tests. E. coli (ATCC25922) was cultured at 37 °C in Luria-Bertani (LB) medium for 24 h to be used in further experiments. According to the Agar disk diffusion method65,66 used to examine the antibacterial effect of the scaffolds, a bacterial suspension was taken out from the Luria-Bertani agar medium after culture and then mixed with saline solution to obtain the concentration of 1 × 107 CFU mL−1. Afterwards, the bacterial suspension was mixed with an agar medium to be diluted at different density of approximately 1 × 105 CFU mL−1 and 1 × 106 CFU mL−1 and be evenly spread over an agar plate. Specimens were carefully placed into the agar plate. After culture for 24 h, the plate was photographed and the bacterial inhibition zone size was measured. According to the turbidimetry method to detect the bacterial inhibition rate, bacterial/culture medium mixing suspensions at 1 × 106 CFU mL−1 in transparent tubes were used to test the antibacterial effect of the specimens. Besides, a transparent tube with the culture medium but without bacteria and specimens was used as blank control, while a transparent tube with bacterial/culture medium but without specimens was selected as the negative control. All tubes were incubated in a rotary shaker at 37 °C for 24 h. And then, the above six types of tubes after incubation were photographed. Absorbance of suspensions was measured using a microplate reader at 600 nm, and the antibacterial rates were calculated according to the equation:
Antibacterial rate (%) = (A1 − A0)/A1 × 100 |
where A0 and A1 are the absorbance of the bacterial suspensions immersing with and without scaffolds, respectively.
Morphologies of the bacteria on the surface of specimens were observed. Specimens were incubated in bacterial/culture medium mixing suspensions at 1 × 106 CFU mL−1 for 24 h. And then, specimens with bacteria were fixed by 2.5% glutaraldehyde for 4 h before washing with PBS. Afterwards, they were dehydrated with a graded series of alcohol (30%, 50%, 70%, 80%, 90%, 100%) for 15 min. Finally, dehydrated specimens were dried for further SEM observation.
3.8 ROS level assay
Intracellular production of ROS was evaluated using 2,7-dichlorofluorescein diacetate (DCFH-DA) provided by Shanghai Macklin Biochemical Co., Ltd, China. In the study, the bacterial cells of 1 × 108 CFU mL−1 were incubated with specimens for 2 h. And then, 5 μM DCFH-DA was added to the treated bacteria solution. The solution was incubated in an orbital shaker (200 rpm) at 37 °C for 45 min. Afterwards, the bacterial suspension was collected by centrifugation and washed with PBS to remove the residual DCFH-DA outside the cell. Finally, the fluorescence signal was captured using a fluorescence microscope and the ROS level was evaluated with a microplate reader. Electron Spin Resonance (ESR) spectra were determined using a Bruker A300 spectrometer. In the experiments, 5,5-dimethyl-1-pyrroline N-oxide (DMPO) acts as a spin-trapping reagent. The measurements were carried out as follows: catalyst (2 mg) was suspended in 500 μL water or 500 μL chromatographic pure methanol containing 50 μL DMPO (the DMPO was prepared by adding 400 μL UP water into 40 μL commercial DMPO) to detect ˙OH or ˙O2− within a Pyrex glass tube (capacity 1.5 mL), and the tube was sealed with a rubber septum cap. Following this, ultrasonication (10 min), the detection of ˙O2− in air, and the detection of ˙OH in an anaerobic environment with Ar bubbling (5 min) were carried out. To initiate each reaction, 2 M hydrogen peroxide was added to a solution containing the PGA/Cu-CMMT powder (3 mg mL−1).
3.9. Statistical analysis
Data were expressed as the mean ± standard deviation. The statistical difference was assessed by SPSS using a Student's t-test, where labels *, ** and *** represent P < 0.05, P < 0.01 and P < 0.001, respectively.
4. Conclusions
In summary, a synergistic antibacterial system was constructed by modification of MMT with Cu2+ and CTAB and then incorporated into the PGA scaffold to endow the scaffold with excellent antibacterial activities. In the synergistic antibacterial system, cation exchange of Cu2+ facilitated the intercalation of CTAB, and simultaneously the intercalation of CTAB contributed to the bacterial killing effect of Cu2+. From the results, the zeta potential of the MMT powder was transformed from negative (−26.1 mV) to positive (19.7 mV) after modification, resulting in the bacterial adsorption rate increasing by 76.5% compared to MMT. Moreover, the PGA/Cu-CMMT scaffold displayed the best antibacterial effect against E. coli with an antibacterial rate of 98.1%. Besides, the mechanism of the antibacterial activity was studied through the formation of ROS and the highest level of ROS production was observed in the PGA/Cu-CMMT scaffold.
Author contributions
Conceptualization, writing–reviewing and editing, Li Yu and Tiantian He; conceptualization, Jia Yao; methodology, Wendi Xu; data curation, writing–original draft preparation, Shuping Peng; visualization, investigation, validation, Pei Feng and Cijun Shuai.
Conflicts of interest
The authors declare that they have no conflict of interest.
Acknowledgements
This work was supported by the following funds: (1) The Natural Science Foundation of China (51905553, 51935014, 82072084, and 81871498); (2) Hunan Provincial Natural Science Foundation of China (2021JJ20061, 2020JJ3047, and 2019JJ50588); (3) The Provincial Key R & D Projects of Jiangxi (20201BBE51012); (4) The Project of State Key Laboratory of High Performance Complex Manufacturing; (5) Fundamental Research Funds for the Central Universities of Central South University (2021zzts0143).
References
- Y. Yang, Y. Cheng, F. Deng, L. Shen, Z. Zhao, S. Peng and C. Shuai, A bifunctional bone scaffold combines osteogenesis and antibacterial activity via in situ grown hydroxyapatite and silver nanoparticles, Bio. Des. Manuf., 2021, 4, 1–17 CrossRef.
- Z. Yang, X. Hao, X. Chen, Z. Ma, W. Wang, C. Wang, L. Yu, H. Sun, Q. Shao, V. Murugadoss and Z. Guo, Long-term antibacterial stable reduced graphene oxide nanocomposites loaded with cuprous oxide nanoparticles, J. Colloid Interface Sci., 2019, 533, 13–23 CrossRef CAS.
- L. Shi, P. Tang, W. Zhang, Y. Zhao, L. Zhang and H. Zhang, Green synthesis of CuO nanoparticles using Cassia auriculata leaf extract and in vitro evaluation of their biocompatibility with rheumatoid arthritis macrophages (RAW 264.7), Trop. J. Pharm. Res., 2017, 16, 185–192 CrossRef CAS.
- M. Appleby, P. Walker, D. Pritchard, S. van Meurs, C. Booth, C. Robertson, M. Ward, D. Kelly and J. Weinstein, Cu(I) diimine complexes as immobilised antibacterial photosensitisers operating in water under visible light, Mater. Adv., 2020, 1, 3417–3427 RSC.
- K. Djoko, M. Goytia, P. Donnelly, M. Schembri, W. Shafer and A. McEwan, Copper II – bis(thiosemicarbazona) to complexes as antibacterial agents: insights into their mode of action and potential as therapeutics, Antimicrob. Agents Chemother., 2015, 59, 6444–6453 CrossRef CAS.
- T. Bai, K. Bai, Z. Lu, X. Liu, Z. Lin, M. Cheng, Z. Li, D. Zhu and L. Zhang, Simple fabrication of Cu2+ doped calcium alginate hydrogel filtration membrane with excellent anti-fouling and antibacterial properties, Chin. Chem. Lett., 2021, 32, 1051–1054 CrossRef CAS.
- Z. Zhu, Q. Gao, Z. Long, Q. Huo, Y. Ge, N. Vianney, N. Daliko, Y. Meng, J. Qu, H. Chen and B. Wang, Polydopamine/poly (sulfobetaine methacrylate) Co-deposition coatings triggered by CuSO4/H2O2 on implants for improved surface hemocompatibility and antibacterial activity, Bioact. Mater., 2021, 6, 2546–2556 CrossRef CAS PubMed.
- E. Krakor, S. Saniternik, I. Gessner, R. Frohnhoven, M. Wilhelm, M. Drexelius, N. Tosun, I. Neundorf and S. Mathur, Hollow mesoporous silica capsules loaded with copper, silver, and zinc oxide nanoclusters for sustained antibacterial efficacy, J. Am. Ceram. Soc., 2021 DOI:10.1111/jace.18002.
- B. Cao, Y. Zheng, T. Xi, C. Zhang, W. Song, K. Burugapalli, H. Yang and Y. Ma, Concentration-dependent cytotoxicity of copper ions on mouse fibroblasts in vitro: effects of copper ion release from TCu380A vs. TCu220C intra-uterine devices, Biomed. Microdevices, 2012, 14, 709–720 CrossRef CAS PubMed.
- H. Geng, Y. Zhao, J. Liu, Y. Cui, Y. Wang, Q. Zhao and S. Wang, Hollow mesoporous silica as a high drug loading carrier for regulation insoluble drug release, Int. J. Pharmaceut., 2016, 510, 184–194 CrossRef CAS PubMed.
- M. Mostafa, M. El-Meligy, M. Sharaf, A. Soliman and M. AbuKhadra, Insight into chitosan/zeolite-A nanocomposite as an advanced carrier for levofloxacin and its anti-inflammatory properties; loading, release, and anti-inflammatory studies, Int. J. Biol. Macromol., 2021, 179, 206–216 CrossRef CAS PubMed.
- J. Poostforooshan, S. Belbekhouche, M. Shaban, V. Alphonse, D. Habert, N. Bousserrhine, J. Courty and A. Weber, Aerosol-assisted synthesis of tailor-made hollow mesoporous silica microspheres for controlled release of antibacterial and anticancer agents, ACS Appl. Mater. Interfaces, 2020, 12, 6885–6898 CrossRef CAS.
- Z. Jia, Z. Gao, D. Lan, Y. Cheng, G. Wu and H. Wu, Effects of filler loading and surface modification on electrical and thermal properties of epoxy/montmorillonite composite, Chin. Phys. B, 2018, 27, 117806 CrossRef.
- H. Yan, P. Zhang, X. Chen, C. Bao, R. Zhao, J. Hu, C. Liu and Q. Lin, Preparation and characterization of octyl phenyl polyoxyethylene ether modified organo-montmorillonite for ibuprofen controlled release, Appl. Clay Sci., 2020, 189, 105519 CrossRef CAS.
- L. Zhang, J. Chen, W. Yu, Q. Zhao and J. Liu, Antimicrobial nanocomposites prepared from montmorillonite/Ag+/quaternary ammonium nitrate, J. Nanomater., 2018, 2018, 6190251 Search PubMed.
- G. Tong, M. Yulong, G. Peng and X. Zirong, Antibacterial effects of the Cu II-exchanged montmorillonite on Escherichia coli K88 and Salmonella choleraesuis, Vet. Microbiol., 2005, 105, 113–122 CrossRef CAS.
- A. Abduraimova, A. Molkenova, A. Duisembekova, T. Mulikova, D. Kanayeva and T. S. Atabaev, Cetyltrimethylammonium bromide CTAB-loaded SiO2-Ag mesoporous nanocomposite as an efficient antibacterial agent, Nanomaterials, 2021, 11, 477 CrossRef CAS PubMed.
- J. Harrison, R. Turner, D. Joo, M. Stan, C. Chan, N. Allan, H. Vrionis, M. Olson and H. Ceri, Copper and quaternary ammonium cations exert synergistic bactericidal and antibiofilm activity against Pseudomonas aeruginosa, Antimicrob. Agents Chemother., 2008, 52, 2870–2881 CrossRef CAS.
- B. Dizman, J. Badger, M. Elasri and L. Mathias, Antibacterial fluoromicas: a novel delivery medium, Appl. Clay Sci., 2007, 38, 57–63 CrossRef CAS.
- G. Chen, Z. Li, X. Wang, L. Xie, Q. Qi and W. Fang, Preparation of CTAB-loaded magnetic nanospheres for rapid bacterial capture and decontamination, Mater. Lett., 2014, 134, 290–294 CrossRef CAS.
- N. Hayeemasae, K. Waesateh, A. Masa and H. Ismail, Halloysite nanotubes filled natural rubber composites: functionality, crystallinity and thermal studies, J. Eng. Sci., 2019, 15, 1–10 CrossRef.
- C. Medcraft, S. Zinn, M. Schnell, A. Poblotzki, J. Altnöder, M. Heger, M. A. Suhm, D. Bernhard, A. Stamm and F. Dietrich, Aromatic embedding wins over classical hydrogen bonding-a multi-spectroscopic approach for the diphenyl ether–methanol complex, Phys. Chem. Chem. Phys., 2016, 18, 25975–25983 RSC.
- M. Lamyaa, Discrimination of pork content in mixtures with raw minced camel and buffalo meat using FTIR spectroscopic technique, Int. Food Res. J., 2013, 20, 1389 CAS.
- L. Júnior, D. Silva, M. Aguiar, C. Melo and K. Alves, Preparation and characterization of polypyrrole/organophilic montmorillonite nanofibers obtained by electrospinning, J. Mol. Liq., 2019, 275, 452–462 CrossRef.
- S. Bhagath, A. Vivek, V. Krishna, S. Mittal and M. Balachandran, Synthesis and characteristics of MMT reinforced chitosan nanocomposite, Mater. Today: Proc., 2021, 46, 4487–4492 CAS.
- C. Shuai, C. He, S. Peng, F. Qi, G. Wang, A. Min, W. Yang and W. Wang, Mechanical alloying of immiscible metallic systems: process, microstructure, and mechanism, Adv. Eng. Mater., 2021, 23, 2001098 CrossRef CAS.
- P. Liang, S. Yang, X. Ouyang, H. Hong and Y. Jiao, Improvement in mechanical properties of NBR/AO60/MMT composites by surface hydrogen bond, Polym. Composit., 2020, 41, 2169–2180 CrossRef CAS.
- S. Strand, K. Vårum and K. Østgaard, Interactions between chitosans and bacterial suspensions: adsorption and flocculation, Colloids
Surf., B, 2003, 27, 71–81 CrossRef CAS.
- T. Guo, S. Cao, R. Su, Z. Li, P. Hu and Z. Xu, Adsorptive property of Cu2+-loaded montmorillonite clays for Escherichia coli K88 in vitro, J. Environ. Sci., 2011, 23, 1808–1815 CrossRef CAS.
- C. Gao, Z. Zeng, S. Peng and C. Shuai, Magnetostrictive alloys: promising materials for biomedical applications, Bioact. Mater., 2021, 8, 177–195 CrossRef.
- T. Tierney, K. Bodnár, Å. Rasmuson and S. Hudson, Carrier particle design for stabilization and isolation of drug nanoparticles, Int. J. Pharmaceut., 2017, 518, 111–118 CrossRef CAS.
- J. Liu, Y. Wang, X. Fan, H. Liu, J. Li, X. He, A. Hui and A. Wang, The high-efficiency synergistic and broad-spectrum antibacterial effect of cobalt doped zinc oxide quantum dots Co-ZnO QDs loaded cetyltributylphosphonium bromide (CTPB) modified MMT C-MMT nanocomposites, Colloids Surf., A, 2021, 613, 126059 CrossRef CAS.
- C. Shuai, B. Peng, P. Feng, L. Yu, R. Lai and A. Min, In situ synthesis of hydroxyapatite nanorods on graphene oxide nanosheets and their reinforcement in biopolymer scaffold, J. Adv. Res., 2021 DOI:10.1016/j.jare.2021.03.009.
- P. Feng, Y. Kong, M. Liu, S. Peng and C. Shuai, Dispersion strategies for low-dimensional nanomaterials and their application in biopolymer implants, Mater. Today Nano, 2021, 15, 100127 CrossRef CAS.
- Y. Yang, M. Yang, C. He, F. Qi, D. Wang, S. Peng and C. Shuai, Rare earth improves strength and creep resistance of additively manufactured Zn implants, Composite, Part B, 2021, 216, 108882 CrossRef CAS.
- K. Mahesh, H. Murthy, B. Kumaraswamy, N. Raghavendra, R. Sridhar, M. Krishna, N. Pattar, R. Pal and B. Sherigara, Synthesis and characterization of organomodified Na-MMT using cation and anion surfactants, Front. Chem. China, 2011, 6, 153–158 CrossRef.
- J. Xiong, Y. Liu, X. Yang and X. Wang, Thermal and mechanical properties of polyurethane/montmorillonite nanocomposites based on a novel reactive modifier, Polym. Degrad. Stab., 2004, 86, 549–555 CrossRef CAS.
- G. Qian, L. Zhang, G. Wang, Z. Zhao, S. Peng and C. Shuai, 3D printed Zn-doped mesoporous silica-incorporated poly-L-lactic acid scaffolds for bone repair, Int. J. Bioprint., 2021, 7, 346 CAS.
- H. Yi, F. Jia, Y. Zhao, W. Wang, S. Song, H. Li and C. Liu, Surface wettability of montmorillonite 0 0 1 surface as affected by surface charge and exchangeable cations: a molecular dynamic study, Appl. Surf. Sci., 2018, 459, 148–154 CrossRef CAS.
- W. Xiao, M. Zhan and Z. Li, Organically modifying and modeling analysis of montmorillonites, Mater. Des., 2003, 24, 455–462 CrossRef CAS.
- K. Shah, M. Mishra, A. Shukla, T. Imae and D. Shah, Controlling wettability and hydrophobicity of organoclays modified with quaternary ammonium surfactants, J. Colloid Interface Sci., 2013, 407, 493–499 CrossRef CAS PubMed.
- R. Revati, M. Majid, M. Ridzuan, K. Basaruddina, M. Rahman, E. Cheng and A. Gibson,
In vitro degradation of a 3D porous Pennisetum purpureum/PLA biocomposite scaffold, J. Mech. Behav. Biomed. Mater., 2017, 74, 383–391 CrossRef CAS PubMed.
- M. Fang, J. Chen, X. Xu, P. Yang and H. Hildebrand, Antibacterial activities of inorganic agents on six bacteria associated with oral infections by two susceptibility tests, Int. J. Antimicrob. Agents, 2006, 27, 513–517 CrossRef CAS.
- Z. Zhang, Y. Wu, Z. Wang, X. Zhang, Y. Zhao and L. Sun, Electrospinning of Ag Nanowires/polyvinyl alcohol hybrid nanofibers for their antibacterial properties, Mater. Sci. Eng., C, 2017, 78, 706–714 CrossRef CAS.
- Y. Alshammari, F. Yang and L. Bolzoni, Low-cost powder metallurgy Ti–Cu alloys as a potential antibacterial material, J. Mech. Behav. Biomed. Mater., 2019, 95, 232–239 CrossRef CAS.
- H. Yu, Y. Zhang, J. Zhang, H. Zhang and J. Liu, Preparation and antibacterial property of SiO2-Ag/PES hybrid ultrafiltration membranes, Desalin. Water Treat., 2013, 51, 3584–3590 CrossRef CAS.
- N. Pal, P. Dubey, P. Gopinath and K. Pal, Combined effect of cellulose nanocrystal and reduced graphene oxide into poly-lactic acid matrix nanocomposite as a scaffold and its anti-bacterial activity, Int. J. Biol. Macromol., 2017, 95, 94–105 CrossRef CAS PubMed.
- J. Yang, C. Liu, Y. Ding, T. Sun, X. Bai, Z. Cao, S. Ramakrishna, J. Zhang and Y. Long, Synergistic antibacterial polyacrylonitrile/gelatin nanofibers coated with metal-organic frameworks for accelerating wound repair, Int. J. Biol. Macromol., 2021, 189, 698–704 CrossRef CAS.
- M. Ahmad, S. Aslam, F. Mustafa and U. Arshad, Synergistic antibacterial activity of surfactant free Ag–GO nanocomposites, Sci. Rep., 2021, 11, 1–9 CrossRef PubMed.
- Y. Yan, C. Li, H. Wu, J. Du, J. Feng, J. Zhang, L. Huang, S. Tan and Q. Shi, Montmorillonite-modified reduced graphene oxide stabilizes copper nanoparticles and enhances bacterial adsorption and antibacterial activity, ACS Appl. Bio Mater., 2019, 2, 1842–1849 CrossRef CAS.
- R. Rastogi, S. Singh, D. Häder and R. Sinha, Detection of reactive oxygen species ROS by the oxidant-sensing probe 2′,7′-dichlorodihydrofluorescein diacetate in the cyanobacterium Anabaena variabilis PCC 7937, Biochem. Biophys. Res. Commun., 2010, 397, 603–607 CrossRef CAS PubMed.
- X. Li, T. Fu, B. Li, P. Yan and Y. Wu, Riboflavin-protected ultrasmall silver nanoclusters with enhanced antibacterial activity and the mechanisms, RSC Adv., 2019, 9, 13275–13282 RSC.
- I. Dror, L. Fink, L. Weiner and B. Berkowitz, Elucidating the catalytic degradation of enrofloxacin by copper oxide nanoparticles through the identification of the reactive oxygen species, Chemosphere, 2020, 258, 127266 CrossRef CAS PubMed.
- L. Wang, Y. Sha, D. Wu, Q. Wei, D. Chen, S. Yang, F. Jia, Q. Yuan, X. Han and J. Wang, Surfactant induces ROS-mediated cell membrane permeabilization for the enhancement of mannatide production, Process Biochem., 2020, 91, 172–180 CrossRef CAS.
- X. Li, S. Hao, A. Han, Y. Yang, G. Fang, J. Liu and S. Wang, Intracellular Fenton reaction based on mitochondria-targeted copper(II) – peptide complex for induced apoptosis, J. Mater. Chem. B, 2019, 7, 4008–4016 RSC.
- G. Luo, W. Chen, S. Hong, Q. Cheng, W. Qiu and X. Zhang, A self-transformable pH-driven membrane-anchoring photosensitizer for effective photodynamic therapy to inhibit tumor growth and metastasis, Adv. Funct. Mater., 2017, 27, 1702122 CrossRef.
- S. Prasad, M. Tiwari, A. Pandey, T. Shrivastav and S. Chaube, Impact of stress on oocyte quality and reproductive outcome, J. Biomed. Sci., 2016, 23, 1–5 CrossRef.
- Y. Yang, C. Lu, L. Shen, Z. Zhao, S. Peng and C. Shuai,
In situ deposition of apatite layer to protect Mg-based composite fabricated via laser additive manufacturing, J. Magnesium Alloys, 2021 DOI:10.1016/j.jma.2021.04.009.
- F. Qi, Z. Zeng, J. Yao, W. Cai, Z. Zhao, S. Peng and C. Shuai, Constructing core-shell structured BaTiO3@ carbon boosts piezoelectric activity and cell response of polymer scaffolds, Mater. Sci. Eng., C, 2021, 126, 112129 CrossRef CAS PubMed.
- P. Singh, A. Tripathy, A. Kainthola, B. Mahanta, V. Singh and T. Singh, Indirect estimation of compressive and shear strength from simple index tests, Eng. Comput. Germany, 2017, 33, 1–11 CrossRef.
- R. Havaldar, S. Pilli and B. Putti, Insights into the effects of tensile and compressive loadings on human femur bone, Adv. Biomed. Res., 2014, 3, 101 CrossRef.
- L. Pan, X. Pei, R. He, Q. Wan and J. Wang, Multiwall carbon nanotubes/polycaprolactone composites for bone tissue engineering application, Colloids Surf. B, 2012, 93, 226–234 CrossRef CAS PubMed.
- S. Mondal, U. Pal and A. Dey, Natural origin hydroxyapatite scaffold as potential bone tissue engineering substitute, Ceram Int., 2016, 42, 18338–18346 CrossRef CAS.
- Y. Ma, B. Yang and L. Xie, Adsorptive property of Cu2+-ZnO/cetylpyridinium-montmorillonite complexes for pathogenic bacterium in vitro, Colloids Surf., B, 2010, 79, 390–396 CrossRef CAS PubMed.
- R. Mahmoudi, S. Aghaei, Z. Salehpour, A. Mousavizadeh, S. Khoramrooz, M. Taheripour Sisakht, G. Christiansen, M. Baneshi, B. Karimi and H. Bardania, Antibacterial and antioxidant properties of phyto-synthesized silver nanop's using Lavandula stoechas extract, Appl. Organomet. Chem., 2020, 34, e5394 CAS.
- P. Weldrick, M. Hardman and V. Paunov, Smart active antibiotic nanocarriers with protease surface functionality can overcome biofilms of resistant bacteria, Mater. Chem. Front., 2021, 5, 961–972 RSC.
Footnote |
† These authors contributed equally to this work. |
|
This journal is © the Partner Organisations 2022 |