DOI:
10.1039/D2QI01956F
(Research Article)
Inorg. Chem. Front., 2022,
9, 6527-6533
An indium-based microporous metal–organic framework with unique three-way rod-shaped secondary building units for efficient methane and hydrogen storage†
Received
10th September 2022
, Accepted 24th October 2022
First published on 24th October 2022
Abstract
A novel microporous indium-based MOF material with unique 3-way rod-shaped secondary building units (SBUs), UTSA-22, was reported and exhibited high methane (CH4) and hydrogen (H2) storage. At 298 K and 65 bar, the total CH4 volumetric uptake of UTSA-22 is 174 cm3(STP) cm−3. Moreover, UTSA-22 shows a high CH4 working capacity of 146 cm3(STP) cm−3 in a pressure range of 5–65 bar at 298 K. In addition, UTSA-22 shows a high H2 gravimetric storage capacity (1.2 wt%) at 298 K and 100 bar.
As a primary greenhouse gas, carbon dioxide has been released globally to reach a record high level as fossil fuel demand is growing tremendously. Methane (CH4), a primary component in natural gas, is considered a potential alternative to liquid fossil fuels since it is clean, abundant, and renewable on earth.1 However, low energy densities have limited its practical applications. In this regard, to utilize methane as a transportation fuel, a suitable adsorbent with high CH4 storage capacity at low pressures will be required (when methane is used as a transportation fuel stored by an adsorbent).2 Therefore, significant interest has been shown in adsorbed natural gas systems to overcome these problems, including filling the tank with porous materials for storing high-density methane at moderate pressures. According to the guidelines of the department of energy (DOE) in the U.S.,3 ambitious targets for volumetric and gravimetric capacities for CH4 storage are up to 350 cm3(STP) cm−3 and 0.5 g/g, respectively, at room temperature (R.T.) for the next generation of clean energy automobiles when considering the ignored loss of the packing adsorbent. Thus, comprehensive research efforts are being devoted to developing novel adsorbent materials with high CH4 storage capacity to achieve these challenging storage goals.4,5
Owing to their high Brunauer–Emmett–Teller (BET) surface areas and tunable pore functions, metal–organic frameworks (MOFs) are emerging as a new generation of crystalline materials that are able to outperform conventionally used activated carbon, zeolites, and silica gels in a multitude of different physico-chemical aspects.6–15 Large numbers of MOFs have been demonstrated to be promising for CH4 storage, considering both volumetric and gravimetric CH4 uptake and storage.16–24 It is worth noting that the CH4 volumetric working capacity (also known as deliverable capacity) is considered to be a much more important parameter to assess the performance of these absorptive materials for practical applications due to the limitations of gas tanks in vehicles, which reflects the actual driving range using natural gas.25–27 At present, to achieve a high working capacity, it is necessary to maximize the amount of methane stored at high pressure and minimize methane storage at low pressure (around 5 bar).28–30 Several strategies have been proven to improve the CH4 working capacity, such as optimizing pore structure, tuning the framework's flexibility, and incorporating strong binding sites.31–35 However, it is still challenging to optimize the pore structure with an appropriate CH4 binding affinity for balancing the trade-off of methane adsorption between low and high pressures to therefore obtain a superior volumetric working capacity.36–39
Herein, a three-dimensional microporous metal–organic framework [In5(TTETA)11/3(OH)4(H2O)·30H2O·19DMF] (UTSA-22, H3TTETA = 4,4′,4′′-((2,4,6-trimethylbenzene-1,3,5-triyl)tris(ethyne-2,1-diyl)) tribenzoic acid), with unique 3-way rod-shaped SBUs, was synthesized under solvothermal conditions. It was found that the activated UTSA-22 shows a high methane uptake of 174 cm3(STP) cm−3 at 298 K and 65 bar, which is higher than those of DUT-4 (164 cm3(STP) cm−3),3 Fe-NDC (160 cm3(STP) cm−3)40 and VNU-22 (155 cm3(STP) cm−3).40 Moreover, this uptake value is comparable to some of the top performing materials when considering the significantly low surface area of UTSA-22 (2173 m2 g−1), such as MOF-205 (183 cm3(STP) cm−3),41 FJI-H23 (179 cm3(STP) cm−3),42 and BUT-22 (182 cm3(STP) cm−3).16 Additionally, the H2 storage capacity of UTSA-22 can reach 1.2 wt% (8.45 g L−1) at 100 bar and 298 K.
Solvothermal reactions of H3TTETA with In(NO3)3·6H2O and nitric acid yielded single crystals of UTSA-22. Single-crystal X-ray diffraction analysis revealed that UTSA-22 crystallizes in the trigonal system, space group R
c. Three independent In3+ atoms, 11/6 TTETA3− ligands, and one μ2-OH− group were observed in the asymmetric unit of UTSA-22. Both In3+ atoms are coordinated with four carboxylate O atoms coming from four different TTETA3− ligands in the equatorial positions and two μ2-OH− groups in the apical positions. The lengths of In–O and In–OH bonds are in the ranges of 2.050(4)–2.209(6) and 2.026(6)–2.094(6) Å, respectively (Table S2†). For the TTETA3− ligand, two carboxyl groups coordinate with two adjacent In3+ atoms in a bi-monodentate coordination, and the remaining carboxyl group coordinates with the In3+ atom in a monodentate mode (Fig. 1). The uncoordinated carboxylate O atom (O12) can form hydrogen bonding interactions with a μ2-OH− group (O2, the distance is 2.6 Å, Fig. S1†). The connection of In3+ atoms with carboxylate and μ2-OH− groups in the order of “In1–In2–In3–In2–In1” results in infinite rod-shaped secondary building units (SBUs). The SBUs are bridged by TTETA3− ligands making a three-dimensional structure with one type of double-wall disordered octahedral cage (Fig. S2†). The diameter of the octahedral cage is about 18 Å. In addition, two types of pore walls with thicknesses of 3.6 and 7.7 Å, respectively, are observed in UTSA-22 (Fig. S3†). Interestingly, the 1D chains in UTSA-22 are arranged in a three-way model, which differs from the commonly observed one-way or two-way models (Fig. 1).43–46 Similar SBUs have been reported in a recently published work.47 It should be pointed out that, while preparing this manuscript, the single crystal structure of UTSA-22 was reported by Li and co-workers, and this MOF was used for the detection of selective antibiotics in water.48 The total potential solvent accessible void volume of the framework is 65% of the whole structure as estimated by PLATON.49
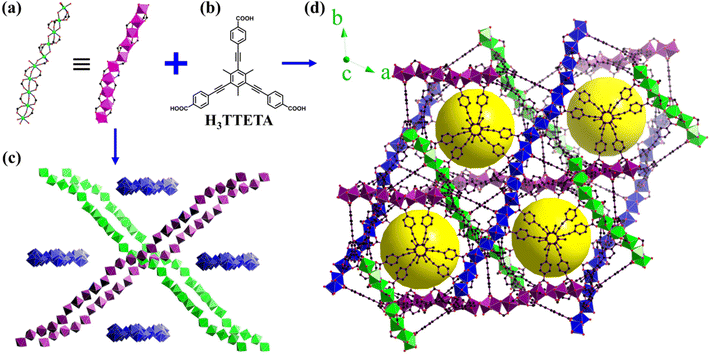 |
| Fig. 1 (a) Infinite 1D rod-shaped SBUs, (b) structural formula of the ligand, H3TTETA, (c) the packing of the SBUs, and (d) a three-dimensional framework structure of UTSA-22 viewed along the crystallographic c-axis (color code: In, turquoise; C, black; and O, red; an octahedral geometry constituted by In and O; and hydrogen atoms are omitted for clarity). | |
The phase purity of UTSA-22 was examined by powder X-ray diffraction (PXRD) measurements (Fig. S5†). The PXRD peaks of the as-synthesized sample match with those of simulated ones obtained from the single-crystal data, proving the high phase purity of UTSA-22. The crystal structure remains intact after activation. In the thermogravimetric analysis (TGA) curve, two steps of weight loss were clearly observed: one is in the temperature range of 21–69 °C with a weight loss of 12%, and the other one is in the temperature range of 69–137 °C with a weight loss of 30% (Fig. S6†). By considering that in the synthesis of UTSA-22, only water, DMF, and HNO3 (10 μL, 16 M) are used, and the trace HNO3 will decompose to NO2 at high temperature, it is thus believed that the two steps of weight loss belong to that of water and DMF in the pores of UTSA-22, respectively. The calculated amount of water and DMF molecules in the pores of UTSA-22 are 30 and 19, respectively, which is reasonable considering the large cell parameters (a = b = 51.504(3) Å, c = 50.096(4) Å; α = β = 90°, γ = 120°; and v = 115
083(17) Å3) of the host framework. The framework of UTSA-22 is stable up to ∼400 °C, followed by its decomposition (Fig. S6†). Besides, in the FT-IR spectra, a slight red shift of the characteristic peaks belonging to the carbonyl group in UTSA-22 was observed compared with those of the H3TTETA ligand, demonstrating the coordination between the carboxylate groups and metals (Fig. S7†). At 77 K, UTSA-22 was examined by N2 adsorption to achieve permanent porosity (Fig. 2a). The saturated N2 uptake of UTSA-22 is 581 cm3(STP) g−1, corresponding to 2173 m2 g−1 as the BET surface area (Fig. S8 and S9†). Therefore, the experimental total pore volume of UTSA-22 is 0.90 cm3 g−1, which is close to the theoretical value of 0.93 cm3 g−1 by PLATON calculation.
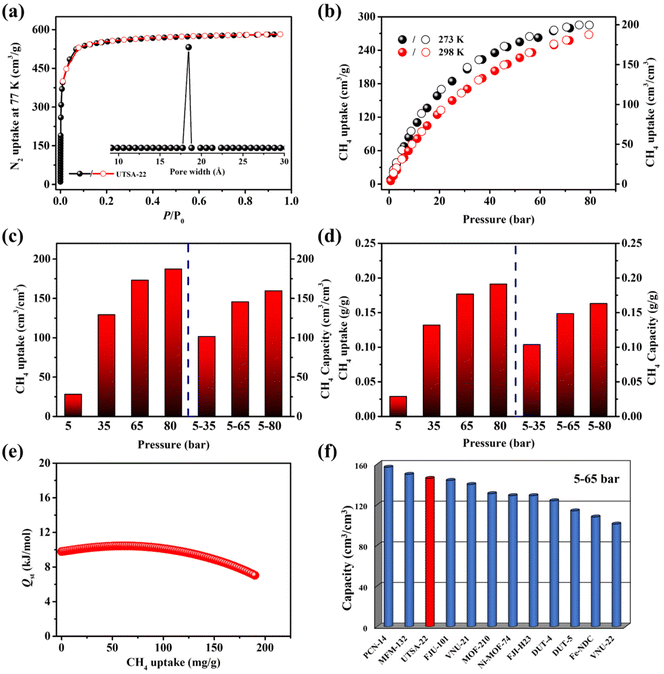 |
| Fig. 2 (a) N2 adsorption/desorption isotherms for UTSA-22 at 77K; (b) CH4 isotherms at 273 and 298 K for UTSA-22 up to 80 bar. Solid symbols: adsorption and open symbols: desorption. (c) Volumetric and (d) gravimetric CH4 uptake (at 5, 35, 65, and 80 bar, respectively)/working capacities (in the pressure ranges of 35–5, 65–5, and 80–5 bar, respectively) of UTSA-22 at 298 K. (e) Qst of CH4 for UTSA-22 obtained using the virial method; and (f) comparison of the volumetric CH4 working capacities (5–65 bar) of UTSA-22 with some benchmark MOF materials. | |
The CH4 storage capacity of UTSA-22 was explored from the beginning accordingly. At 273 and 298 K, CH4 adsorption isotherms were measured from 0 to 80 bar, respectively. As shown in Fig. 2b, at 35 bar and 298 K, the total gravimetric CH4 uptake of UTSA-22 is 183 cm3(STP) g−1, exceeding the DOE's previous goal (180 cm3(STP) g−1), without regard for packing density loss. At 65 and 80 bar, the total gravimetric CH4 uptake rates of UTSA-22 are 249 and 268 cm3(STP) g−1 at 298 K, which correspond to 0.179 and 0.192 g/g, respectively, which are much higher than those of some benchmark MOFs such as Ni-MOF-74 (210 (223) cm3(STP) g−1),50 VNU-22 (132 (140) cm3(STP) g−1),40 and Cu-tbo-MOF-5 (208 (225) cm3(STP) g−1)50 under identical conditions (Table S3†). In addition, at 80 bar and 298 K, the volumetric CH4 uptake is 188 cm3(STP) cm−3, comparable to those of VNU-21 (194 cm3(STP) cm−3),40 BUT-22 (202 cm3(STP) cm−3),16 and MFM-132 (213 cm3(STP) cm−3).34
The working capacity is another important factor that needs to be considered while assessing porous materials for practical methane storage. The working capacity is the difference in the total adsorption from 5 to 80 (or 65) bar. As shown in Table S3,† at 298 K, the CH4 volumetric working capacity (65–5 bar) for UTSA-22 is 146 cm3(STP) cm−3, which is comparable or higher than those widely explored MOFs like Ni-MOF-74 (129 cm3(STP) cm−3),50 FJU-101 (144 cm3(STP) cm−3),17 DUT-4 (124 cm3(STP) cm−3),3 and VNU-22 (101 cm3(STP) cm−3).40 When the temperature is reduced to 273 K, the CH4 volumetric working capacity (65–5 bar) increases to 157 cm3(STP) cm−3, which is higher than some well-known microporous MOFs such as NiMOF-74 (106 cm3(STP) cm−3),51 ZJU-70 (134 cm3(STP) cm−3),52 MOF-505 (112 cm3(STP) cm−3),53 and PCN-14 (153 cm3(STP) cm−3).51 Additionally, the adsorption enthalpy (Qst) of UTSA-22 is 9.8 kJ mol−1 (Fig. 2e), which is lower than that found for most reported MOFs (Table S3†). Such a low adsorption enthalpy involving host–guest interactions is significantly important when CH4 gas is released from the gas tank.
The H2 isotherms of UTSA-22 were recorded up to 100 bar at 273 and 298 K. As shown in Fig. 3, the gravimetric H2 of UTSA-22 at 298 K and 100 bar is 1.2 wt%, which is higher than the values for most reported MOFs, such as Co2(BDC)2(dabco) (0.32 wt%),54 Cu2(BDC)2(dabco) (0.42 wt%),54 JUC-48 (1.1 wt%),55 Mg2(dobdc) (0.8 wt%),56 and Cu(peip) (0.46 wt%)57 under identical conditions. Besides, UTSA-22 shows a remarkably high H2 volumetric uptake of 8.45 g L−1 at 298 K and 100 bar, which is higher than some famous MOFs such as NU-1501-Al (8.40 g L−1)58 and Mg2(dobdc) (7.50 g L−1).56 The Qst of H2 for UTSA-22 is 12.3 kJ mol−1 at zero bar based on the isotherms obtained at 298 and 273 K (Fig. S12†).
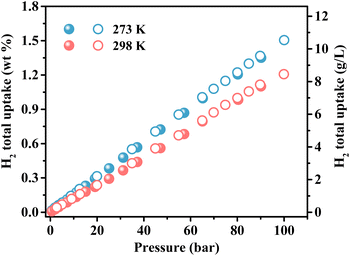 |
| Fig. 3 High-pressure hydrogen adsorption isotherms for UTSA-22 at 273 and 298 K. | |
Conclusions
A novel microporous In-based MOF with unique three-way rod-shaped SBUs, named UTSA-22, has been designed and synthesized for efficient CH4 and H2 storage. Owing to its cage-type structure, UTSA-22 has a modest BET surface area of 2173 m2 g−1 and is stable up to 400 °C. UTSA-22 shows a high CH4 gravimetric storage capacity of 268 cm3(STP) g−1 (0.192 g/g) at 80 bar and 298 K. The CH4 volumetric delivery capacity (65–5 bar) of UTSA-22 is 146 cm3(STP) cm−3 at 298 K, comparable to or higher than some benchmark MOF materials. Furthermore, the H2 gravimetric uptake is 1.2 wt% for UTSA-22 at 298 K and 100 bar. Therefore UTSA-22 can be potentially used in CH4 and H2 storage applications.
Conflicts of interest
There are no conflicts to declare.
Acknowledgements
This work was supported by the Welch Foundation (AX-1730). The authors extend their appreciation to the Deputyship for Research & Innovation, Ministry of Education, in Saudi Arabia for funding this research work through the project number DRI-KSU-572. R.-B. L. wants to thank the NSFC (22101307) for its support. X-ray diffraction studies at the Molecular Foundry and Advanced Light Source was supported by the Office of Science, Office of Basic Energy Sciences, of the U.S. Department of Energy (DE-AC02-05CH11231). The authors would like to express their sincere appreciation to Dr. Kaijun Lu from the Marine Science Institute at the University of Texas at Austin for assistance with the thermogravimetric analysis.
References
- J. Jiang, H. Furukawa, Y.-B. Zhang and O. M. Yaghi, High Methane Storage Working Capacity in Metal–Organic Frameworks with Acrylate Links, J. Am. Chem. Soc., 2016, 138, 10244–10251 CrossRef CAS PubMed.
- T. A. Makal, J.-R. Li, W. Lu and H.-C. Zhou, Methane storage in advanced porous materials, Chem. Soc. Rev., 2012, 41, 7761–7779 RSC.
- Y. He, W. Zhou, G. Qian and B. Chen, Methane storage in metal–organic frameworks, Chem. Soc. Rev., 2014, 43, 5657–5678 RSC.
- Z. Chen, M. R. Mian, S.-J. Lee, H. Chen, X. Zhang, K. O. Kirlikovali, S. Shulda, P. Melix, A. S. Rosen, P. A. Parilla, T. Gennett, R. Q. Snurr, T. Islamoglu, T. Yildirim and O. K. Farha, Fine-Tuning a Robust Metal–Organic Framework toward Enhanced Clean Energy Gas Storage, J. Am. Chem. Soc., 2021, 143, 18838–18843 CrossRef CAS.
- J. A. Mason, J. Oktawiec, M. K. Taylor, M. R. Hudson, J. Rodriguez, J. E. Bachman, M. I. Gonzalez, A. Cervellino, A. Guagliardi, C. M. Brown, P. L. Llewellyn, N. Masciocchi and J. R. Long, Methane storage in flexible metal–organic frameworks with intrinsic thermal management, Nature, 2015, 527, 357–361 CrossRef CAS PubMed.
- H. Cui, Y. Ye, T. Liu, Z. A. Alothman, O. Alduhaish, R.-B. Lin and B. Chen, Isoreticular Microporous Metal–Organic Frameworks for Carbon Dioxide Capture, Inorg. Chem., 2020, 59, 17143–17148 CrossRef CAS.
- H. Cui, Y. Xie, Y. Ye, Y. Shi, B. Liang and B. Chen, An Ultramicroporous Metal-Organic Framework with Record High Selectivity for Inverse CO2/C2H2 Separation, Bull. Chem. Soc. Jpn., 2021, 94, 2698–2701 CrossRef CAS.
- H. Cui, Y. Ye, H. Arman, Z. Li, A. Alsalme, R.-B. Lin and B. Chen, Microporous Copper Isophthalate Framework of mot Topology for C2H2/CO2 Separation, Cryst. Growth Des., 2019, 19, 5829–5835 CrossRef CAS.
- X. Zhang, R.-B. Lin, J. Wang, B. Wang, B. Liang, T. Yildirim, J. Zhang, W. Zhou and B. Chen, Optimization of the Pore Structures of MOFs for Record High Hydrogen Volumetric Working Capacity, Adv. Mater., 2020, 32, 1907995 CrossRef CAS PubMed.
- R.-B. Lin, S. Xiang, B. Li, Y. Cui, G. Qian, W. Zhou and B. Chen, Our journey of developing multifunctional metal-organic frameworks, Coord. Chem. Rev., 2019, 384, 21–36 CrossRef CAS.
- W. Gong, H. Cui, Y. Xie, Y. Li, X. Tang, Y. Liu, Y. Cui and B. Chen, Efficient C2H2/CO2 Separation in Ultramicroporous Metal–Organic Frameworks with Record C2H2 Storage Density, J. Am. Chem. Soc., 2021, 143, 14869–14876 CrossRef CAS PubMed.
- Y. Xie, Y. Shi, H. Cui, R.-B. Lin and B. Chen, Efficient Separation of Propylene from Propane in an Ultramicroporous Cyanide-Based Compound with Open Metal Sites, Small Struct., 2022, 3, 2100125 CrossRef CAS.
- Y. Shi, Y. Xie, H. Cui, Z. A. Alothman, O. Alduhaish, R.-B. Lin and B. Chen, An ultramicroporous metal–organic framework with dual functionalities for high sieving separation of CO2 from CH4 and N2, Chem. Eng. J., 2022, 446, 137101 CrossRef CAS.
- D. Zhao, X. Wang, L. Yue, Y. He and B. Chen, Porous metal–organic frameworks for hydrogen storage, Chem. Commun., 2022, 58, 11059–11078 RSC.
- D. Zhao, K. Yu, X. Han, Y. He and B. Chen, Recent progress on porous MOFs for process-efficient hydrocarbon separation, luminescent sensing, and information encryption, Chem. Commun., 2022, 58, 747–770 RSC.
- B. Wang, X. Zhang, H. Huang, Z. Zhang, T. Yildirim, W. Zhou, S. Xiang and B. Chen, A microporous aluminum-based metal-organic framework for high methane, hydrogen, and carbon dioxide storage, Nano Res., 2021, 14, 507–511 CrossRef CAS.
- Y. Ye, R.-B. Lin, H. Cui, A. Alsalme, W. Zhou, T. Yildirim, Z. Zhang, S. Xiang and B. Chen, A microporous metal–organic framework with naphthalene diimide groups for high methane storage, Dalton Trans., 2020, 49, 3658–3661 RSC.
- H.-X. Li, Z.-H. Zhang, H. Fang, D.-X. Xue and J. Bai, Synthesis, structure and high methane storage of pure D6R Yb(Y) nonanuclear cluster-based zeolite-like metal–organic frameworks, J. Mater. Chem. A, 2022, 10, 14795–14798 RSC.
- D. Alezi, Y. Belmabkhout, M. Suyetin, P. M. Bhatt, Ł. J. Weseliński, V. Solovyeva, K. Adil, I. Spanopoulos, P. N. Trikalitis, A.-H. Emwas and M. Eddaoudi, MOF Crystal Chemistry Paving the Way to Gas Storage Needs: Aluminum-Based soc-MOF for CH4, O2, and CO2 Storage, J. Am. Chem. Soc., 2015, 137, 13308–13318 CrossRef CAS PubMed.
- C. Song, Y. Ling, Y. Feng, W. Zhou, T. Yildirim and Y. He, A NbO-type metal–organic framework exhibiting high deliverable capacity for methane storage, Chem. Commun., 2015, 51, 8508–8511 RSC.
- Q.-Y. Yang, P. Lama, S. Sen, M. Lusi, K.-J. Chen, W.-Y. Gao, M. Shivanna, T. Pham, N. Hosono, S. Kusaka, J. J. Perry IV, S. Ma, B. Space, L. J. Barbour, S. Kitagawa and M. J. Zaworotko, Reversible Switching between Highly Porous and Nonporous Phases of an Interpenetrated Diamondoid Coordination Network That Exhibits Gate-Opening at Methane Storage Pressures, Angew. Chem., Int. Ed., 2018, 57, 5684–5689 CrossRef CAS PubMed.
- T. Kundu, B. B. Shah, L. Bolinois and D. Zhao, Functionalization-Induced Breathing Control in Metal–Organic Frameworks for Methane Storage with High Deliverable Capacity, Chem. Mater., 2019, 31, 2842–2847 CrossRef CAS.
- Y. Yan, M. Juríček, F.-X. Coudert, N. A. Vermeulen, S. Grunder, A. Dailly, W. Lewis, A. J. Blake, J. F. Stoddart and M. Schröder, Non-Interpenetrated Metal–Organic Frameworks Based on Copper(II) Paddlewheel and Oligoparaxylene-Isophthalate Linkers: Synthesis, Structure, and Gas Adsorption, J. Am. Chem. Soc., 2016, 138, 3371–3381 CrossRef CAS PubMed.
- C.-C. Liang, Z.-L. Shi, C.-T. He, J. Tan, H.-D. Zhou, H.-L. Zhou, Y. Lee and Y.-B. Zhang, Engineering of Pore Geometry for Ultrahigh Capacity Methane Storage in Mesoporous Metal–Organic Frameworks, J. Am. Chem. Soc., 2017, 139, 13300–13303 CrossRef CAS.
- K. A. Forrest, G. Verma, Y. Ye, J. Ren, S. Ma, T. Pham and B. Space, Methane storage in flexible and dynamical metal–organic frameworks, Chem. Phys. Rev., 2022, 3, 021308 CrossRef CAS.
- Y. Fang, S. Banerjee, E. A. Joseph, G. S. Day, M. Bosch, J. Li, Q. Wang, H. Drake, O. K. Ozdemir, J. M. Ornstein, Y. Wang, T.-B. Lu and H.-C. Zhou, Incorporating Heavy Alkanes in Metal–Organic Frameworks for Optimizing Adsorbed Natural Gas Capacity, Chem. – Eur. J., 2018, 24, 16977–16982 CrossRef CAS PubMed.
- W. Zhou, H. Wu, M. R. Hartman and T. Yildirim, Hydrogen and Methane Adsorption in Metal−Organic Frameworks: A High-Pressure Volumetric Study, J. Phys. Chem. C, 2007, 111, 16131–16137 CrossRef CAS.
- H. Li, L. Li, R.-B. Lin, W. Zhou, Z. Zhang, S. Xiang and B. Chen, Porous metal-organic frameworks for gas storage and separation: Status and challenges, EnergyChem, 2019, 1, 100006 CrossRef.
- C.-X. Chen, Z.-W. Wei, J.-J. Jiang, S.-P. Zheng, H.-P. Wang, Q.-F. Qiu, C.-C. Cao, D. Fenske and C.-Y. Su, Dynamic Spacer Installation for Multirole Metal–Organic Frameworks: A New Direction toward Multifunctional MOFs Achieving Ultrahigh Methane Storage Working Capacity, J. Am. Chem. Soc., 2017, 139, 6034–6037 CrossRef CAS.
- T. Kundu, M. Wahiduzzaman, B. B. Shah, G. Maurin and D. Zhao, Solvent-Induced Control over Breathing Behavior in Flexible Metal–Organic Frameworks for Natural-Gas Delivery, Angew. Chem., Int. Ed., 2019, 58, 8073–8077 CrossRef CAS PubMed.
- H.-M. Wen, K. Shao, W. Zhou, B. Li and B. Chen, A novel expanded metal–organic framework for balancing volumetric and gravimetric methane storage working capacities, Chem. Commun., 2020, 56, 13117–13120 RSC.
- M. Zhang, C. Chen, Z. Shi, K. Huang, W. Fu and W. Zhou, Inserting Amide into NOTT-101 to Sharply Enhance Volumetric and Gravimetric Methane Storage Working Capacity, Inorg. Chem., 2019, 58, 13782–13787 CrossRef CAS PubMed.
- J.-M. Lin, C.-T. He, Y. Liu, P.-Q. Liao, D.-D. Zhou, J.-P. Zhang and X.-M. Chen, A Metal–Organic Framework with a Pore Size/Shape Suitable for Strong Binding and Close Packing of Methane, Angew. Chem., Int. Ed., 2016, 55, 4674–4678 CrossRef CAS.
- Y. Yan, D. I. Kolokolov, I. da Silva, A. G. Stepanov, A. J. Blake, A. Dailly, P. Manuel, C. C. Tang, S. Yang and M. Schröder, Porous Metal–Organic Polyhedral Frameworks with Optimal Molecular Dynamics and Pore Geometry for Methane Storage, J. Am. Chem. Soc., 2017, 139, 13349–13360 CrossRef CAS PubMed.
- M. Zhang, W. Zhou, T. Pham, K. A. Forrest, W. Liu, Y. He, H. Wu, T. Yildirim, B. Chen, B. Space, Y. Pan, M. J. Zaworotko and J. Bai, Fine Tuning of MOF-505 Analogues To Reduce Low-Pressure Methane Uptake and Enhance Methane Working Capacity, Angew. Chem., Int. Ed., 2017, 56, 11426–11430 CrossRef CAS PubMed.
- J. A. Mason, M. Veenstra and J. R. Long, Evaluating metal–organic frameworks for natural gas storage, Chem. Sci., 2014, 5, 32–51 RSC.
- D. A. Gómez-Gualdrón, T. C. Wang, P. García-Holley, R. M. Sawelewa, E. Argueta, R. Q. Snurr, J. T. Hupp, T. Yildirim and O. K. Farha, Understanding Volumetric and Gravimetric Hydrogen Adsorption Trade-off in Metal–Organic Frameworks, ACS Appl. Mater. Interfaces, 2017, 9, 33419–33428 CrossRef PubMed.
- C. Song, H. Liu, J. Jiao, D. Bai, W. Zhou, T. Yildirim and Y. He, High methane storage and working capacities in a NbO-type metal–organic framework, Dalton Trans., 2016, 45, 7559–7562 RSC.
- Y. He, F. Chen, B. Li, G. Qian, W. Zhou and B. Chen, Porous metal–organic frameworks for fuel storage, Coord. Chem. Rev., 2018, 373, 167–198 CrossRef CAS.
- T. N. Tu, H. T. D. Nguyen and N. T. Tran, Tailoring the pore size and shape of the one-dimensional channels in iron-based MOFs for enhancing the methane storage capacity, Inorg. Chem. Front., 2019, 6, 2441–2447 RSC.
- H. Furukawa, N. Ko, Y. B. Go, N. Aratani, S. B. Choi, E. Choi, A. Ö. Yazaydin, R. Q. Snurr, M. O'Keeffe, J. Kim and O. M. Yaghi, Ultrahigh Porosity in Metal-Organic Frameworks, Science, 2010, 329, 424–428 CrossRef CAS PubMed.
- P. Huang, C. Chen, Z. Hong, J. Pang, M. Wu, F. Jiang and M. Hong, Azobenzene Decorated NbO-Type Metal–Organic Framework for High-Capacity Storage of Energy Gases, Inorg. Chem., 2019, 58, 11983–11987 CrossRef CAS PubMed.
- M. Krüger, A. K. Inge, H. Reinsch, Y.-H. Li, M. Wahiduzzaman, C.-H. Lin, S.-L. Wang, G. Maurin and N. Stock, Polymorphous Al-MOFs Based on V-Shaped Linker Molecules: Synthesis, Properties, and in Situ Investigation of Their Crystallization, Inorg. Chem., 2017, 56, 5851–5862 CrossRef PubMed.
- L. Li, S. Wang, T. Chen, Z. Sun, J. Luo and M. Hong, Solvent-Dependent Formation of Cd(II) Coordination Polymers Based on a C2-Symmetric Tricarboxylate Linker, Cryst. Growth Des., 2012, 12, 4109–4115 CrossRef CAS.
- A. Schoedel, M. Li, D. Li, M. O'Keeffe and O. M. Yaghi, Structures of Metal–Organic Frameworks with Rod Secondary Building Units, Chem. Rev., 2016, 116, 12466–12535 CrossRef CAS PubMed.
- V. Colombo, S. Galli, H. J. Choi, G. D. Han, A. Maspero, G. Palmisano, N. Masciocchi and J. R. Long, High thermal and chemical stability in pyrazolate-bridged metal–organic frameworks with exposed metal sites, Chem. Sci., 2011, 2, 1311–1319 RSC.
- Y.-F. Zhang, Z.-H. Zhang, L. Ritter, H. Fang, Q. Wang, B. Space, Y.-B. Zhang, D.-X. Xue and J. Bai, New Reticular Chemistry of the Rod Secondary Building Unit: Synthesis, Structure, and Natural Gas Storage of a Series of Three-Way Rod Amide-Functionalized Metal–Organic Frameworks, J. Am. Chem. Soc., 2021, 143, 12202–12211 CrossRef CAS PubMed.
- Y.-L. Zhao, Q. Chen, J. Lv, M.-M. Xu, X. Zhang and J.-R. Li, Specific sensing of antibiotics with metal-organic frameworks based dual sensor system, Nano Res., 2022, 15, 6430–6437 CrossRef CAS.
- A. Spek, Single-crystal structure validation with the program PLATON, J. Appl. Crystallogr., 2003, 36, 7–13 CrossRef CAS.
- F. Gándara, H. Furukawa, S. Lee and O. M. Yaghi, High Methane Storage Capacity in Aluminum Metal–Organic Frameworks, J. Am. Chem. Soc., 2014, 136, 5271–5274 CrossRef.
- Y. Peng, V. Krungleviciute, I. Eryazici, J. T. Hupp, O. K. Farha and T. Yildirim, Methane Storage in Metal–Organic Frameworks: Current Records, Surprise Findings, and Challenges, J. Am. Chem. Soc., 2013, 135, 11887–11894 CrossRef CAS PubMed.
- X. Duan, C. Wu, S. Xiang, W. Zhou, T. Yildirim, Y. Cui, Y. Yang, B. Chen and G. Qian, Novel Microporous Metal–Organic Framework Exhibiting High Acetylene and Methane Storage Capacities, Inorg. Chem., 2015, 54, 4377–4381 CrossRef CAS PubMed.
- Y. He, W. Zhou, T. Yildirim and B. Chen, A series of metal–organic frameworks with high methane uptake and an empirical equation for predicting methane storage capacity, Energy Environ. Sci., 2013, 6, 2735–2744 RSC.
- T. Takei, J. Kawashima, T. Ii, A. Maeda, M. Hasegawa, T. Kitagawa, T. Ohmura, M. Ichikawa, M. Hosoe, I. Kanoya and W. Mori, Hydrogen Adsorption Properties of Lantern-Type Dinuclear M(BDC)(DABCO)1/2, Bull. Chem. Soc. Jpn., 2008, 81, 847–856 CrossRef CAS.
- Q.-R. Fang, G.-S. Zhu, Z. Jin, Y.-Y. Ji, J.-W. Ye, M. Xue, H. Yang, Y. Wang and S.-L. Qiu, Mesoporous Metal–Organic Framework with Rare etb Topology for Hydrogen Storage and Dye Assembly, Angew. Chem., Int. Ed., 2007, 46, 6638–6642 CrossRef CAS.
- K. Sumida, C. M. Brown, Z. R. Herm, S. Chavan, S. Bordiga and J. R. Long, Hydrogen storage properties and neutron scattering studies of Mg2(dobdc)—a metal–organic framework with open Mg2+ adsorption sites, Chem. Commun., 2011, 47, 1157–1159 RSC.
- X. Liu, M. Oh and M. S. Lah, Size- and Shape-Selective Isostructural Microporous Metal–Organic Frameworks with Different Effective Aperture Sizes, Inorg. Chem., 2011, 50, 5044–5053 CrossRef CAS PubMed.
- Z. Chen, P. Li, R. Anderson, X. Wang, X. Zhang, L. Robison, L. R. Redfern, S. Moribe, T. Islamoglu, D. A. Gómez-Gualdrón, T. Yildirim, J. F. Stoddart and O. K. Farha, Balancing volumetric and gravimetric uptake in highly porous materials for clean energy, Science, 2020, 368, 297–303 CrossRef CAS PubMed.
Footnotes |
† Electronic supplementary information (ESI) available. CCDC 2204487. For ESI and crystallographic data in CIF or other electronic format see DOI: https://doi.org/10.1039/d2qi01956f |
‡ These authors contributed equally to this work. |
|
This journal is © the Partner Organisations 2022 |