DOI:
10.1039/D2QI00338D
(Research Article)
Inorg. Chem. Front., 2022,
9, 2880-2894
Structural investigation of the efficient capture of Cs+ and Sr2+ by a microporous Cd–Sn–Se ion exchanger constructed from mono-lacunary supertetrahedral clusters†
Received
14th February 2022
, Accepted 15th April 2022
First published on 20th April 2022
Abstract
Visualization of the ion exchange mechanism for 137Cs and 90Sr decontamination is important for safe radioactive liquid waste reprocessing and emergency response improvement in the event of a nuclear accident. Here, the remediation of Cs+ and Sr2+ was achieved through ion exchange using a cadmium selenidostannate, [CH3NH3]3[NH4]3Cd4Sn3Se13·3H2O (CdSnSe-1), with rapid exchange kinetics, high β/γ radiation resistances, broad pH durability and facile elution. The framework constructed from mono-lacunary supertetrahedral clusters features a great negative charge density of 3.27 × 10−3 that accounts for the superhigh exchange capacities of 371.4 (Cs+) and 128.4 mg g−1 (Sr2+). Single-crystal structural analysis on the exchanger during the “pristine–ion exchange–elution” cycle supplies instructive information to elucidate the uptake and recycle mechanism for Cs+ and Sr2+. The broken symmetry of the cluster caused by a vacant site, combined with the co-templating effects of mixed methylammonium/ammonium, contributes to the formation of voids I and II that show adsorption activity for both Cs+ and K+ ions. In comparison, the divalent Sr2+ ions with higher hydration degree exchange with (alkyl)ammonium cations in a 1
:
2 molar ratio, resulting in its location at a new void (III) closer to the framework and thus a higher binding strength. The energy variation during the adsorption process based on a DFT calculation illustrates the high efficiency of CdSnSe-1 for capture of both Cs+ and Sr2+. This “visualized” ion exchange underlines the robustness and flexibility of CdSnSe-1 as a Cs+ and Sr2+ trapper, and reveals the deeper structure–function relationship from a new surface interaction viewpoint.
Introduction
The rapid growth of the nuclear energy industry has brought about an increasing amount of hazardous radionuclides that can cause significant damage to the environment and public health.1,2137Cs and 90Sr, with half lives of around 30 years, are two primary fission products from 235U, and they can emit β or γ radiation and exhibit high levels of toxicity for human bodies, especially for people affected by nuclear accidents.3,4 For example, the Fukushima nuclear leakage accident in 2011 resulted in the release of large quantities of 137Cs+ and 90Sr2+ ions into the surrounding environment. With a high migration ability, they are enriched in water,5 soil,6 forest vegetation7 and other organisms.8 Upon ingestion, 137Cs+ can accumulate in body fluid/soft tissues and cause damage or death to cells,9 while 90Sr2+ is preferentially fixed in bone mass and increases the risk of illnesses like osteoporosis, leukemia, or even bone cancer.10,11 On the other hand, 137Cs+ and 90Sr2+ ions generate large amounts of heat during their decay processes, which pose a requirement for greater storage room and better encapsulation technology in geological repositories.12 This leads to a great risk for the storage of nuclear liquid waste over a long period. In this context, the efficient removal of 137Cs+ and 90Sr2+ can not only improve the emergency response capability in the event of a nuclear accident, but also reduce the cost and burden of safer nuclear liquid waste reprocessing. The recovered 137Cs and 90Sr radionuclides can be further purified as excellent heating or radiation sources for nuclear batteries, thermoelectric generators and cancer radiotherapy, providing feasible ways for their recycling and comprehensive utilization.13–15
Hitherto, Cs+ and Sr2+ pollutants have been eliminated through a variety of technologies, like precipitation,16,17 solvent extraction,18,19 membrane filtration,20,21 biological treatment,22,23 and ion exchange.24,25 However, there are some specific disadvantages with these methods. For example, precipitation cannot sufficiently reduce the ion concentration below the limit level to meet the relevant water quality standards, and moreover, it introduces large quantities of salt ions or solution pH deviation, which necessitates complex secondary treatment. The application of solvent extraction is subject to the use of toxic organic extractants. Membrane filtration suffers from membrane fouling, low recovery rate and high energy consumption. Moreover, biological treatment is usually sensitive to pH, temperature, nutrient availability, etc. and thus the microbes could be readily inhibited or poisoned as they encounter a sudden change of environment. In comparison, ion exchange emerges as a promising method for Cs+ and Sr2+ removal owing to its high efficiency, facile operation, and simple device, to name several advantages. In most cases, the ion exchange method will produce high-quality treated effluent, and it will not cause secondary pollution due to the safe landfill disposal of used adsorbents. Inorganic porous materials with high radiation and thermal stabilities have been extensively employed for the removal of radioactive Cs+ and Sr2+, as confirmed by the great development made in research on zeolites,26,27 clays,28,29 titanium silicates,30,31 phosphates,32,33 molybdates,34etc. However, these oxide exchangers usually lose their activities in acidic/alkaline or highly salty environments, and thus cannot be used in the extreme conditions in the practical treatment of nuclear liquid wastes. Therefore, developing new advanced materials for the effective removal of Cs+ and Sr2+ from aqueous solutions has recently received extensive public attention.
Metal chalcogenide materials with labile extra framework cations are a type of prospective ion exchanger because they possess soft chalcogen atoms (e.g. S, Se) that show a strong affinity to softer metal cations against harder H+, Na+ or Ca2+. Hitherto, important progress has been obtained on the uptake of Cs+ and Sr2+ by metal sulfides, e.g. KMS-1,35 KMS-2,36 KTS-3,37 FJSM-SnS,38 ZnSnS-1,39 InS-1,40 InS-2,41 KZTS,42 NaTS,43 SbS-1K,44 oxysulfides as InSnOS materials,45,46 and selenides like K14Cd15Sn12Se46,47 CuGeSe-1,48 and AgSnSe-1.49 These ion exchangers exhibit fast exchange kinetics, superior capacities, excellent selectivity and good acid/base and radiation resistances. However, there are still serious unresolved issues that hinder the design and preparation of new advanced exchangers. One of the issues is the uncertainty of the exact adsorption site for Cs+ and Sr2+ in most cases due to the lack of good crystallinity on the exchange process, as limited to recent examples including InSnS-1,50 FJSM-SbS,51 ZnSnS-1,39 CuGeSe-1,48 and AgSnSe-1.49 In addition, the effects of the chemical environment on adsorption selectivity are not well understood, especially for cases where the intercalation of larger hydrated ions is inhibited to some extent. This context proposes higher requests for the study of the ion exchange mechanism at a molecular level, which relies on the building of a robust chalcogenide framework with versatile tunnels/voids for cation encapsulation.
Highlighted here is a porous material construction strategy using lacunary supertetrahedral clusters as building units, which is proposed primarily for the following three reasons. (a) With large size and remarkable interlinking capability, the supertetrahedral clusters prove to be effective building units for constructing microporous frameworks with an open tunnel system, which is a critical premise for the transfer of metal ions. (b) The formation of vacant sites in the cluster would cause molecular symmetry breaking and thus enrich the specific voids (i.e. adsorption sites) with different chemical environments to detect their distinction in metal uptake. (c) As illustrated previously,40 the employment of lacunary clusters as building units can effectively increase the negative charge density of the framework, which is beneficial for elevating the theoretical exchange capacity of ion exchangers. In the present work, this hypothesis was achieved by the deep eutectic solvothermal preparation of a cadmium selenidostannate, namely [CH3NH3]3[NH4]3Cd4Sn3Se13·3H2O (CdSnSe-1), which is constructed from the interlinkage of mono-lacunary P1-[Cd4Sn3Se16]12− clusters. CdSnSe-1 exhibits high ion exchange capacities for both Cs+ (371.4 mg g−1) and Sr2+ (128.4 mg g−1), together with rapid kinetics, wide pH durability, high radiation resistance, facile elution and good selectivity against various alkali/alkaline earth metal cations. Impressively, the structural distinction of adsorption sites caused by cluster symmetry breaking and its effect on the adsorption selectivity for Cs+ and Sr2+ was first revealed on the basis of the successful collection of single-crystal structures for pristine, Cs+ and Sr2+ exchanged (CdSnSe-1Cs and CdSnSe-1Sr) and K+ elution products (CdSnSe-1Cs-K and CdSnSe-1Sr-K). This work visualizes the intercalation and elution of Cs+ and Sr2+ at the molecular level, not only opening up a new perspective clarifying the ion exchange mechanism, but also underlining the metal chalcogenides constructed from lacunary clusters as advanced ion exchangers for radionuclide decontamination (Fig. 1).
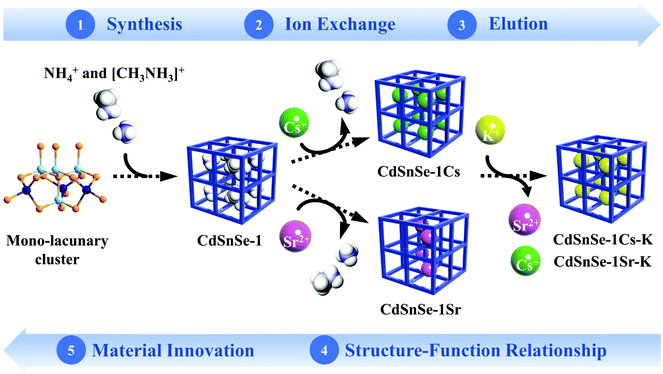 |
| Fig. 1 Schematic representation for the construction of CdSnSe-1 and the following Cs+, Sr2+ ion exchange and K+-elution processes, during which a significant structure–function relationship was revealed for optimizing the design and synthesis of materials. | |
Experimental section
Materials
CdCl2·2.5H2O (99%, Sinopharm Chemical Reagent Co. Ltd), Sn (99.99% Aladdin Reagent Co. Ltd), Se (99.99%, Aladdin Reagent Co. Ltd), diethylamine hydrochloride (99%, Aladdin Reagent Co. Ltd), N,N′-dimethylurea (98%, Aladdin Reagent Co. Ltd), hydrazine hydrate (>98%, Aladdin Reagent Co. Ltd), CsCl (99.9%, Aladdin Reagent Co. Ltd), SrCl2·6H2O (99.5%, Shanghai Macklin Biochemical Co. Ltd), NaCl (GR, Sinopharm Chemical Reagent Co. Ltd), KCl (GR, Aladdin Reagent Co. Ltd), MgCl2·6H2O (99%, Shanghai Macklin Biochemical Co. Ltd), CaCl2·2H2O (98%, Beijing J&K Scientific Co. Ltd), hydrochloric acid (AR, Sinopharm Chemical Reagent Co. Ltd) and NaOH (AR, Sinopharm Chemical Reagent Co. Ltd) were commercially available reagents and used without additional purification.
Characterizations and irradiation studies
Energy-dispersive spectroscopy (EDS) and elemental distribution maps were recorded on an FEI Quanta FEG 250 scanning electron microscope. FTIR spectra were obtained on a PerkinElmer Frontier Mid-IR FTIR spectrometer using KBr pellets. UV–vis reflectance of the solid-state samples (BaSO4 for 100% reflectance) was recorded on a PerkinElmer Lambda 750 UV–vis spectrophotometer. The absorption spectra were transformed from reflectance spectra according to the Kubelka–Munk function: F(R) = (1 − R)2/2R, where F(R) and R represent the absorption and reflectance, respectively.52 Powder X-ray diffraction (XRD, Cu-Kα, λ = 1.54178 Å) was performed on a Rigaku SmartLab 9 kW diffractometer. X-ray photoelectron spectroscopy (XPS) was performed on a Thermo Scientific ESCALAB 250Xi spectrometer. H, C, and N elemental analyses of solid-state samples were performed using an Elementar Vario EL cube instrument. 1H and 13C NMR of the samples was performed on a Bruker Avance III 400 instrument at room temperature, and mixed N2H4·H2O (98%)/D2O was used as the solvent. The metal ion concentrations were measured using a Vista-MPX Varian inductively coupled plasma optical emission spectrometer (ICP-OES) and a ThermoFisher iCAP RQ inductively coupled plasma mass spectrometer (ICP-MS). CdSnSe-1 single crystals were irradiated using γ ray for 100 kGy (1.2 kGy h−1) and β ray for 100 and 200 kGy (50 kGy h−1). The γ irradiation was conducted using a 60Co source that was provided by Suzhou CNNC Huadong Radiation Co. Ltd, Jiangsu Province, China. The β irradiation was performed using 10 MeV electron beams that were generated using an electron accelerator from CGN Dasheng Electron Accelerator Co. Ltd., Jiangsu Province, China.
Synthesis of CdSnSe-1
A mixture of CdCl2·2.5H2O (0.305 g, 1.33 mmol), Sn (0.119 g, 1 mmol), Se (0.42 g, 5.32 mmol), diethylamine hydrochloride (0.384 g, 3.5 mmol), N,N′-dimethylurea (0.617 g, 7 mmol) and N2H4·H2O (0.8 mL, 16.5 mmol) was sealed in a 25 mL Teflon liner encapsulated in a stainless steel reactor and heated at 160 °C for 3 days. After natural cooling to room temperature, the product was washed using deionized water and then dried in air. Pure orange block-like crystals of CdSnSe-1 were collected in a mass of 0.48 g, with a yield of 70.7% based on Sn. Anal. calcd for C3H36N6O3Cd4Sn3Se13 (CdSnSe-1, FM = 2036.53): C, 1.769; H, 1.782; N, 4.127. Found: C, 1.791; H, 1.980; N, 4.151.
Ion exchange experiments
Ion exchange experiments were conducted using nonradioactive Cs and Sr isotopes in a typical batch method at a V/m ratio of 1000 mL g−1. A quantity of 20 mg of CdSnSe-1 was added to a covered vial containing 20 mL of CsCl or SrCl2 aqueous solution with predetermined Cs+ or Sr2+ concentrations, and the mixture was constantly stirred at room temperature (∼20 °C) for 24 h to reach the adsorption equilibrium. Thereafter, the exchanged product was collected by filtration (Whatman 102 paper) and washed using deionized water (DW) and then dried in air. It was characterized using powder XRD, XPS, FTIR, UV–vis, EDS, elemental distribution maps, etc. The exchange-saturated products were obtained from 0.1 M CsCl and 0.1 M SrCl2 solutions, and their formulas were defined as Cs5.6[CH3NH3]0.2[NH4]0.2Cd4Sn3Se13·3.5H2O (CdSnSe-1Cs) and Sr2.85[CH3NH3]0.15[NH4]0.15Cd4Sn3Se13·6.5H2O (CdSnSe-1Sr), respectively. Anal. calcd for C0.2H9Cd4Cs5.6N0.4O3.5Se13Sn3 (CdSnSe-1Cs, FM = 2649.52): N, 0.212. Found: N, 0.096. Anal. calcd for C0.15H14.5Cd4N0.3O6.5Se13Sn3Sr2.85 (CdSnSe-1Sr, FM = 2206.49): N, 0.190. Found: N, 0.182. The Cs+ and Sr2+ metal ions in the filtrate need to be diluted to a concentration range below 200 ppb for ICP-MS characterization.
The individual Cs+ and Sr2+ (∼6 ppm) ion exchange kinetics were studied in a contact time range of 0–2880 min. The removal rate R (%), distribution coefficient Kd (mL g−1) and equilibrium adsorption amount qe (mg g−1) were calculated using eqn (1)–(3),
| 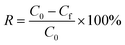 | (1) |
| 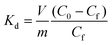 | (2) |
| 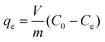 | (3) |
where
C0 (ppm),
Cf (ppm) and
Ce (ppm) represent the initial, final and equilibrium concentrations of Cs
+ and Sr
2+ in aqueous solution,
m (g) is the mass of the ion exchanger, and
V (mL) is the volume of the test solution. The fitting of the experimental data was conducted using a pseudo-second-order kinetic model as described by
eqn (4),
|  | (4) |
where
k2 (g mg
−1 min
−1) and
qt (mg g
−1) represent the rate constant and exchange capacity at time
t (min), respectively.
The individual Cs+ (∼3–824 ppm) and Sr2+ (∼3–741 ppm) ion exchange isotherms were recorded at room temperature for a 24 h contact time. The equilibrium curves were fitted by Langmuir (eqn (5)) and Langmuir–Freundlich (eqn (6)) isotherm models,
|  | (5) |
| 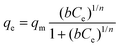 | (6) |
where
b (L mg
−1) and
qm (mg g
−1) account for the Langmuir constant and maximum exchange capacity, respectively, and
n is a parameter reflecting the heterogeneity of the system.
The pH effects on the individual Sr2+ and Cs+ (∼6 ppm) ion exchange were studied in solutions with the required pH (0–14) that were prepared by diluting Cs+ or Sr2+ from 600 ppm to ∼6 ppm using accurately concentrated HCl and NaOH. The contact time for ion exchange was 24 h, and the final pH after the experiment was also measured. The solid product after the ion exchange experiments was checked using powder XRD.
By analogy, the effects of Na+, K+, Mg2+, Ca2+ cations (0–100 mmol L−1) on the individual Cs+ and Sr2+ (∼6 ppm) ion exchange were studied using a similar method, just replacing the pH adjustment with the dissolution of the required amount of NaCl, KCl, MgCl2, CaCl2 in the solution. In addition, deionized water (DW), mineral water (MW, from Nongfu spring), tap water (TW, Xiqing District, Tianjin), and lake water (LW, Tianjin University of Technology, Tianjin) were taken as exchange media to assess the adsorption of Cs+ or Sr2+ in actual environments.
For elution, the Cs+ and Sr2+ exchange-saturated products, i.e., CdSnSe-1Cs and CdSnSe-1Sr (20 mg for each), were treated with 1 M KCl solution (20 mL) with constant stirring for a contact time of 24 h. The solid products were separated using filtration (Whatman 102 paper), washed and dried in air. The products were characterized using powder XRD, TG, EDS and elemental mapping, and finally defined by the formulas K6Cd4Sn3Se13·5H2O (CdSnSe-1Cs-K) and K5.3Sr0.35Cd4Sn3Se13·6.5H2O (CdSnSe-1Sr-K).
Crystallography
The indexing and data collection of CdSnSe-1, CdSnSe-1Cs, CdSnSe-1Sr, CdSnSe-1Cs-K and CdSnSe-1Sr-K single crystals were conducted at 293 K using a Rigaku XtaLab PRO diffractometer with graphite-monochromated Cu Kα radiation (λ = 1.54178 Å). A multi-scan technique was adopted for the absorption corrections. The Cd, Sn and Se atoms in the structure were solved using a direct method (SHELXS-97), and the remaining atoms were revealed by successive Fourier syntheses (SHELXL2014).53 Refinements were performed for all data using full matrix least squares against |F|2. All of the Cd, Sn, Se, Cs, Sr, K, O, C and N atoms were anisotropically refined, while the hydrogen atoms around the O, C and N atoms were generated with idealized geometries. The details for crystallographic data and relevant structure refinement are listed in Tables S1 and S2.†
Density functional theory (DFT) calculations
The Vienna Ab initio Simulation Package (VASP) with projector augmented wave (PAW) potentials was used for the first principles calculations.54,55 The Perdew–Burke–Ernzerhof (PBE) functional of the generalized gradient approximation (GGA) was adopted as the exchange correlation function.56 The threshold of 10−4 eV and the plane-wave cutoff energy of 400 eV were set for the self-consistent-field convergence of the total electronic energy. The adsorption energy (ΔEad) was calculated as: | ΔEad = E(fram + ad) − E(fram) − E(ad) | (7) |
where E(fram + ad) is the energy of the framework with one adsorbed Cs+ or Sr2+ ion, and E(fram), and E(ad) are the energies of the framework and one Cs+ or Sr2+ ion, respectively.
Results and discussion
Synthesis
CdSnSe-1 was prepared by reacting CdCl2·2.5H2O, Sn and Se in a deep eutectic solvent (DES) consisting of diethylamine hydrochloride and N,N′-dimethylurea in a molar ratio of 1
:
2. According to our previous studies, DESs prove to be excellent media for the preparation of metal chalcogenides owing to their bifunctional role as both a solvent and a source of templates.48,49,57–61 For example, the DES employed here provides a unique ionic environment that facilitates faster dissolution of reagents and crystal growth of the product, resulting in a relatively shorter reaction time (3 days) and higher yield (70.7%) for CdSnSe-1. In addition, N,N′-dimethylurea underwent in situ hydrolysis during the reaction and the generated methylammonium cations were important charge-compensating and structure-directing agents to create appropriate chemical environments for metal adsorption. It is noticeable that N2H4·H2O (98%) was added to the reaction system and the N2H4·H2O
:
N,N′-dimethylurea molar ratio needed to be tuned to 2.36
:
1 for isolation of the optimal CdSnSe-1 product. The decomposition of N2H4·H2O gives ammonium species that perform as co-templates with the methylammonium cations. Such a co-templating mechanism integrates well with the broken symmetry of the cluster due to the introduction of a vacant site, which accounts for the formation of voids with distinct chemical environments and adsorption selectivity.
Structural description
CdSnSe-1 crystallizes in the trigonal R3m space group, featuring an anionic framework of [Cd4Sn3Se13]n6n− templated by mixed methylammonium and ammonium cations. Isomorphic frameworks were also observed in compounds K6Cd4Sn3Se13 and K3Rb3Zn4Sn3Se13 where alkaline metal ions were present as counter cations.62,63 The [Cd4Sn3Se16]12−-constructing block for the 3D framework appears to be a mono-lacunary derivative from the plenary P1-[Cd4Sn4Se17]10− cluster upon removing one [SnSe]2+ group at the terminal position (Fig. 2a). Each lacunary [Cd4Sn3Se16]12− cluster interconnects with six adjacent ones via the apical or impending Se2− ligands (Se(2)) to form an anionic [Cd4Sn3Se13]n6n− open framework (Fig. 2b and c). The framework possesses intersecting micropores, whose trigonal {Cd5Sn4Se9} cross-section is large enough (11.82 × 11.15 Å2) for the transfer of hydrated Cs+ (rH = 3.29 Å) and Sr2+ (rH = 4.12 Å) ions (Fig. 2d and Fig. S1†).64 Equivalent amounts of mixed ammonium and methylammonium were filled in the void spaces as charge-compensating agents. These cations exhibit relatively weak electrostatic interactions and hydrogen bonds with the framework, endowing them with promising mobilities that would undoubtedly facilitate the Cs+ and Sr2+ ion exchange process.
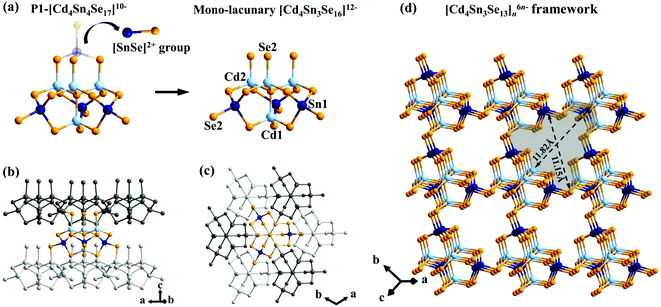 |
| Fig. 2 (a) Structural derivation from a plenary P1-[Cd4Sn4Se17]10− cluster to a mono-lacunary [Cd4Sn3Se16]12− cluster. (b and c) Side and top view of the interconnection between the central [Cd4Sn3Se16]12− cluster (shown in color) and six surrounding ones (shown in pale and dark gray) in CdSnSe-1. (d) Representation of the [Cd4Sn3Se13]n6n− framework of CdSnSe-1. The gray-highlighted moiety is the {Cd5Sn4Se9} window. | |
Characterization of the exchange-saturated products
The Cs+ and Sr2+ exchange-saturated products, i.e. Cs5.6[CH3NH3]0.2[NH4]0.2Cd4Sn3Se13·3.5H2O (CdSnSe-1Cs) and Sr2.85[CH3NH3]0.15[NH4]0.15Cd4Sn3Se13·6.5H2O (CdSnSe-1Sr), keep the block-like crystal morphology very like that of the CdSnSe-1 exchanger, apart from the color change from orange to red and the fracture-caused rough surfaces (Fig. 3a). As shown in Fig. 3b, the compounds are shown to be direct semiconductors as the photon energy (hν) and the band-gap energy (Eg) have a simple relation as αhν = const. × (hν − Eg)1/2, where α can be computed using the Kubelka–Munk function α/S = F(R) = (1 − R)2/2R.52 It is clear that the ion exchange leads to a red shift of optical band gaps from 2.14 eV (CdSnSe-1) to 1.66 eV (CdSnSe-1Cs) or 1.64 eV (CdSnSe-1Sr), in consistent with the color change of appearance. EDS analysis and element mapping images illustrate that Cs+ and Sr2+ ions enter the exchanger and exhibit a homogeneous distribution in the exchanged samples (Fig. 3c and Fig. S2–S4†). Elemental analysis suggests a significant decrease in N components in the solid product upon the Cs+ and Sr2+ uptake, reconfirming the ion exchange mechanism for the adsorption process. All the pristine and exchanged materials were characterized by powder XRD (Fig. 3d). They show similarity in diffraction patterns but distinction in 2θ values, peak numbers and intensities, suggesting that the ion exchange is an isotactic process. The retention of the framework is further illustrated by single-crystal structures (as will be discussed below in detail), whose simulated powder XRD patterns match well with the experimental ones. In addition, 200 kGy β or 100 kGy γ radiation would not cause structural and crystal damage to CdSnSe-1 (Fig. S5†), illustrating the good radiolytic stability of the exchanger for capturing radioactive isotopes.
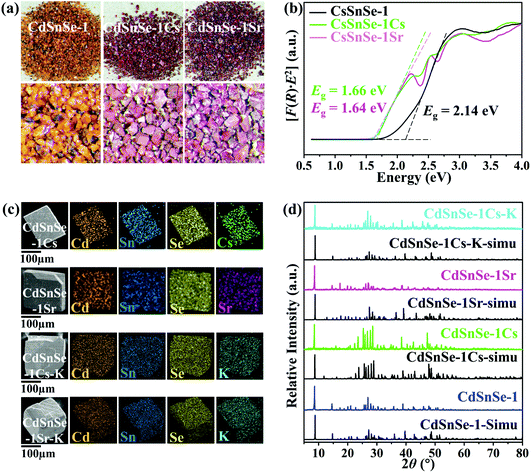 |
| Fig. 3 (a) Exterior appearances, (b) Kubelka–Munk spectra, (c) SEM and elemental mapping images and (d) powder XRD patterns of pristine CdSnSe-1 and ion-exchanged products CdSnSe-1Cs and CdSnSe-1Sr. Eluted products CdSnSe-1Cs-K and CdSnSe-1Sr-K are also included in (c) and (d). | |
High-resolution XPS of CdSnSe-1, CdSnSe-1Cs and CdSnSe-1Sr show characteristic peaks for Cd and Sn elements (Fig. 4a). The binding energies of 495.3 (Sn 3d3/2) and 486.9 eV (Sn 3d5/2) demonstrate the +IV oxidation state for Sn during the ion exchange process, without any chemical reduction being detected. The characteristic peaks for the adsorbed Cs+, Sr2+ and K+ ions are assigned to binding energies of 738.1 (Cs 3d3/2), 724.2 (Cs 3d5/2), 135.9 (Sr 3d3/2), 133.8 (Sr 3d5/2), 295.5 (K 2p1) and 293.0 eV (K 2p3) (Fig. 4b). Notably, the binding energy for Se 3d3/2 (54.3 eV) is raised to higher values upon ion exchange (54.6 eV for Cs+ adsorption, 54.7 eV for Sr2+ adsorption and elution by K+), which implies a decrease in the negative charge density of Se2− owing to the electron transfer to Cs+, Sr2+ and K+ ions (Fig. 4c). This implies that the adsorbed metal ions interact more intensely with the Se2− ions compared with the original ammonium and methylammonium ions. All these characteristic peaks are presented in the survey spectra (Fig. 4d).
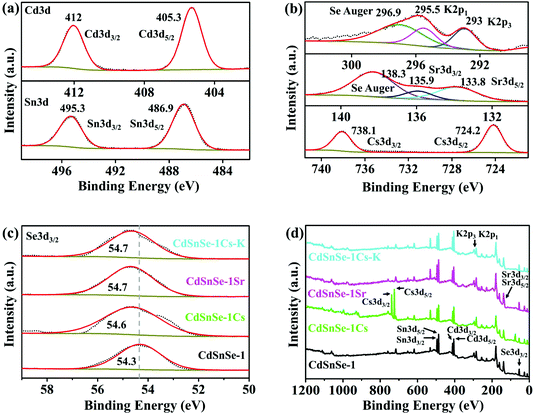 |
| Fig. 4 High-resolution XPS spectra of (a) Cd 3d, Sn 3d, (b) Cs 3d, Sr 3d, K 2p, (c) Se 3d and (d) survey XPS spectra before and after ion exchange with Cs+, Sr2+, and eluted by K+. The overall fitting and experimental curves are shown in red solid and black dotted lines, respectively. | |
Ion exchange kinetics and isotherms
The uptake of Cs+ and Sr2+ ions by CdSnSe-1 single crystals with increasing contact time was investigated (Fig. 5a and b). The removal rates of Cs+ and Sr2+ were 71.06% and 74.45% within 4 h and remained almost unchanged after 24 h. At equilibrium (48 h for example), the removal rate of Sr2+ (94.85%) was slightly higher than that of Cs+ (91.72%), implying an intrinsically stronger affinity of CdSnSe-1 to Sr2+. The plots of t/qtvs. t were well fitted with the pseudo-second-order model, giving high correlation coefficients R2 of 0.99982 for Cs+ and 0.99922 for Sr2+ (insets of Fig. 5a and b). The adsorption rate constants k2 of CdSnSe-1 were determined to be 0.00212 g mg−1 min−1 for Cs+ and 0.00373 g mg−1 min−1 for Sr2+, which are comparable with the values for some other metal selenides, e.g., CuGeSe-1 (0.00635 g mg−1 min−1 for Cs+)48 and AgSnSe-1 (0.00895 g mg−1 min−1 for Cs+).49 The calculated values of qe were 5.79 mg g−1 for Cs+ and 5.85 mg g−1 for Sr2+, in line with the practical ones (5.61 mg g−1 for Cs+ and 5.75 mg g−1 for Sr2+). These reconfirm the superior fit of the pseudo-second-order kinetic model to experimental data, suggesting that the chemical adsorption is the rate-determining step in the Cs+ or Sr2+ adsorption process.
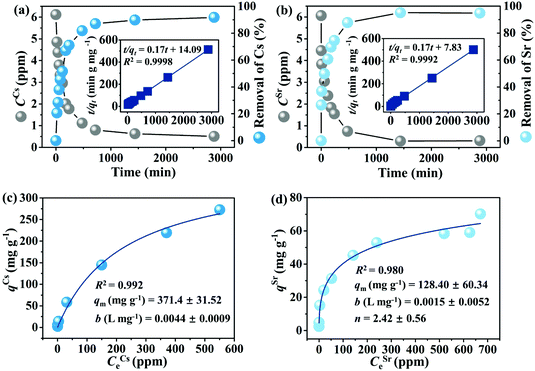 |
| Fig. 5 (a and b) Variation of Cs+ and Sr2+ concentration and their removal rates with contact time t (min). Insert: plot of t/qt (min g mg−1) vs. t according to the kinetic data (C0 ∼ 6 ppm, pH = 6, V/m = 1000 mL g−1, room temperature). (c and d) Equilibrium curves of Cs+ and Sr2+ adsorption by CdSnSe-1 (CCs0 ∼ 3–824 ppm, CSr0 ∼ 3–741 ppm). | |
The ion exchange isotherms of CdSnSe-1 were recorded for Cs+ (C0 ∼ 3–824 ppm) and Sr2+ (C0 ∼ 3–741 ppm) at room temperature. Fig. 5c and d shows that the exchange capacity of Cs+ or Sr2+ increases with the equilibrium concentration. The equilibrium curve of Cs+ ion exchange can be fitted well by the Langmuir model (R2 = 0.992), giving a maximum exchange capacity qCsm of 371.4 mg g−1 that accounts for 94.9% of the theoretical capacity (391.5 mg g−1) (Fig. 5c). The qCsm is 1.8–8 times higher than the capacities of commonly used natural or artificially prepared Cs+ adsorbents like clay (46.3 mg g−1),65 Zeolite A (207.5 mg g−1),66 titanium silicate TAM-5 (191.8 mg g−1),67 polyoxometalates I-as (134 mg g−1),68 and HKUST-1 (153 mg g−1),69 and is comparable with those of some best metal chalcogenide materials such as KMS-2 (531.7 mg g−1),36 InSnOS (537.7 mg g−1)46 and FJSM-SnS (408.9 mg g−1) (Tables S7 and 8†).38 As for Sr2+ exchange, the Langmuir–Freundlich isotherm model fits better with the equilibrium curve (R2 = 0.980) than the Langmuir one (R2 = 0.939) (Fig. 5d). The higher Langmuir–Freundlich constant (n = 2.42) indicates that the adsorption deviates from the Langmuir isotherm model (n = 1),37,70 which is reasonable because the number of adsorbed Sr2+ ions is only half that of the exchanged ammonium and methylammonium cations and thereby results in a lower surface coverage. The maximum exchange capacity qSrm was determined to be 128.4 mg g−1, which is ∼99.5% of the theoretical capacity (129.1 mg g−1). A complete ionic substitution is rarely reported for metal chalcogenide Sr2+ exchangers, whose cation-accessible space is commonly insufficient for the accommodation of large hydrated Sr2+ (e.g. [Sr(H2O)6]2+) ions in a full stoichiometric number.38,71 In contrast, the qSrm of CdSnSe-1 exceeds those of the oxide adsorbents, e.g. clay (39.7 mg g−1),65 Na2V6O16·3H2O (96.4 mg g−1),72 SZ-4 (117.9 mg g−1),73 and FJSM-CA (21.3 mg g−1),74 and even ranks CdSnSe-1 ahead of some of the best metal chalcogenides like KMS-1 (77 mg g−1),35 KMS-2 (86.89 mg g−1),36 KTS-3 (102 mg g−1),37 NaTS (80 mg g−1),43 InS-1 (105.35 mg g−1),40 and ZnSnS-1 (124.2 mg g−1),39 and very close to FJSM-SnS-4 (141.22 mg g−1)75 and the record-holder InS-2 (143.29 mg g−1).41
The excellent Cs+ and Sr2+ exchange capacities stem from the high negative charge density of the [Cd4Sn3Se13]n6n− framework, which was calculated to be 3.27 × 10−3 according to the equation ρ = z/Mr.40 For each building block, the introduction of one vacant site into the plenary P1-[Cd4Sn4Se17]10− cluster brings about a reduced molecular weight (from 2266.8 to 2069.1) and increased negative charge number (from −10 to −12), synergistically enhancing the negative charge density of the newly formed lacunary cluster. This pronounced ρ value exceeds those of other selenide ion exchangers like [NH4]4In12Se20 (1.35 × 10−3),76 CuGeSe-1 (1.93 × 10−3)48 and AgSnSe-1 (1.53 × 10−3),49 and it compares well with those of extensively studied metal sulfides such as KMS-1 (1.94 to 3.89 × 10−3),71 KMS-2 (1.99 to 4.40 × 10−3)36 and FJSM-SnS (3.44 × 10−3).38 Consequently, the rich negative charges of the [Cd4Sn3Se13]n6n− framework need to be compensated by a great quantity of ammonium and methylammonium cations, endowing CdSnSe-1 with a high theoretical exchange capacity for Cs+ and Sr2+.
Elution
The elution of Cs+ and Sr2+ ions can be easily achieved by exposing Cs+- and Sr2+-laden products to highly concentrated K+ (1 M KCl). EDS analysis and elemental mapping indicate that 100% of Cs+ and 88.3% of Sr2+ were replaced by K+ (Fig. 3c and Fig. S10, S11†), giving the analogous potassium salts of cadmium selenidostannate, i.e. K6Cd4Sn3Se13·5H2O (CdSnSe-1Cs-K) and K5.3Sr0.35Cd4Sn3Se13·6.5H2O (CdSnSe-1Sr-K). Once eluted, the original block-like morphology of the exchanger was well retained, with no obvious cracks or fractures. The powder XRD patterns shown in Fig. S12† indicate the retention of the framework upon elution treatment, reflecting an excellent mechanical resistance for the alternating transfer of cations. Moreover, the eluted products can be further used for adsorption towards Cs+ and Sr2+ (Fig. S13 and S14†), whose removal rates were 64.97% and 61.54% within 4 h and remained almost unchanged after 24 h. The equilibrium removal rates of Cs+ and Sr2+ were determined to be 92.64% and 98.01%, comparable with those of the pristine exchanger. The retention of the framework structure and exchange performance reveals a recycling potential of the material for industry practice.
Structural and theoretical investigation of the ion exchange
Accurate structural investigations on the Cs+ and Sr2+ exchange-saturated and eluted products were performed by means of single-crystal XRD to give illuminating insights into the ion exchange mechanism. All four samples, i.e.CdSnSe-1Cs, CdSnSe-1Sr, CdSnSe-1Cs-K and CdSnSe-1Sr-K, crystallize in the same trigonal R3m space group as that of CdSnSe-1, featuring the same parent framework architecture. Upon the uptake of Cs+, the unit cell volume expands by 2.5%, from 3097.30(6) to 3176.24(11) Å3, as the length of c axis is increased from 16.0233(2) to 16.4222(4) Å. This stems probably from the steric interactions from larger Cs+ ions to the framework, which is mirrored by an enlargement of the Cd(2)–Se(2)–Sn(1) bond angle from 97.35 to 99.23°, as well as a broadening of the {Cd5Sn4Se9} window from 11.82 × 11.15 to 12.01 × 11.15 Å2. Once the Cs+ (structural ionic radius rs: 1.69 Å) is eluted by the smaller K+ (rs:1.33 Å),64 the a/b and c axes are shortened to 14.8258(2) and 16.0744(4) Å, respectively, resulting in a unit cell contraction back to 3059.86 (11) Å3 that is 1.2% smaller even than that of the pristine exchanger. In comparison, although the growth of the c axis was also observed for the incorporation of Sr2+, the a and b axes were contrarily shortened from 14.94000(10) to 14.7181(2) Å due to the fact that the (alkyl)ammonium ions were replaced by Sr2+ in a half quantity owing to the +2 charge on the latter being twice as high as that on the former. This contributes to an overall retention of the unit cell volume upon Sr2+ exchange. The framework flexibility of materials bears importance for some specific functions such as separation, purification, and molecular/ion recognition.77–80 Nevertheless, the dynamic response has rarely been investigated for metal selenide exchangers, being limited to examples like K14−xHxCd15Sn12Se46 (x ≈ 7),47 [NH4]4In12Se20,76 CuGeSe-1,48 and AgSnSe-1,49 hampering the deeper understanding of the selective adsorption mechanism.
The introduction of a vacant site reduces the cluster symmetry from Td to C3v, with a 3-fold rotation axis passing through the vacant site and the center of three apical vertices of the cluster. This configuration gives rise to an obvious distinction in chemical environment between the space voids that are positioned around the vacant site (void I) of the cluster and that at the hollow between two apical vertices (void II), respectively (Fig. 6a and b). Void I is occupied by one methylammonium cation with the methyl group pointing outwards from the cluster, while void II is filled by an inner ammonium cation together with an outer water molecule. Consequently, for each cluster, there are three methylammonium cations evenly distributed around the truncated vertex, and three extra ammonium cations located at the edge centers of the bottom plane generated by the other three vertices (Fig. 6c–e). According to the elemental analysis, 93.3% of the ammonium and methylammonium ions are substituted by Cs+ ions, which are located at both voids I (Cs(1)) and II (Cs(2)) with equivalent occupancies (Fig. 6f). Both Cs(1)+ and Cs(2)+ are surrounded by six Se2− ions and the Cs⋯Se distance ranges from 3.6270(15) to 3.9320(17) Å. A bridging aqua ligand (O(1W), ∼50% occupancy) is located between adjacent Cs(1)+ and Cs(2)+ ions, and there is another extra water molecule (O(2W), ∼50% occupancy) detected close to Cs(2)+ (Fig. 6g and h). The case becomes different for the Sr2+-laden product, where more than 95% (based on elemental analysis) ammonium and methylammonium ions are replaced. Distinct from the two-positional distribution of Cs+, all the Sr2+ ions are located at a new site (denoted as void III) that remains in parallel with the plane generated by two impending Se(2)2− ions around the vacant site and two bridging Se(4)2− ions from two adjacent clusters (Fig. 6i). In this case, each Sr2+ interacts with the overall four Se2− ions of the framework, featuring a shorter Sr⋯Se distance range of 3.212(4)–3.275(3) Å compared with Cs⋯Se interaction. The remaining space of the Sr2+ coordination sphere is filled with approximately two aqua ligands that are distributed at four positions (O(1W), O(2W), O(3W) and O(4W)), forming a {(H2O)2Sr} plane that is perpendicular to the {Se4Sr} plane (Fig. 6j and k). The higher hydration degree of the adsorbed Sr2+ (Sr2+
:
H2O ≈ 1
:
2 in molar ratio) compared with Cs+ (Cs+
:
H2O ≈ 2
:
1 in molar ratio) is in good agreement with the hydrated radius order of rSrH (4.12 Å) > rCsH (3.29 Å) in aqueous solution. Upon elution, both Cs+ and Sr2+ adsorbed in the exchanger would be substituted by K+ ions, which occupy almost the same positions as the Cs+ because of their common alkaline metal nature (Fig. 6l). The hydration degree of the K+ salt analog is detected to be higher than the Cs+-laden product, in accordance with the similarity of Cs+ and K+ in hydrated radii (Cs+, 3.29 Å; K+, 3.31 Å), but distinction in structural radii (Cs+, 1.69 Å; K+, 1.33 Å) (Fig. 6m and n). That is to say, the hydration tendency of Sr2+ > K+ > Cs+ is well kept in the crystal lattice, where the Sr2+, K+ and Cs+ metal centers are coupled with approximately two, one and one-half water molecules, respectively.
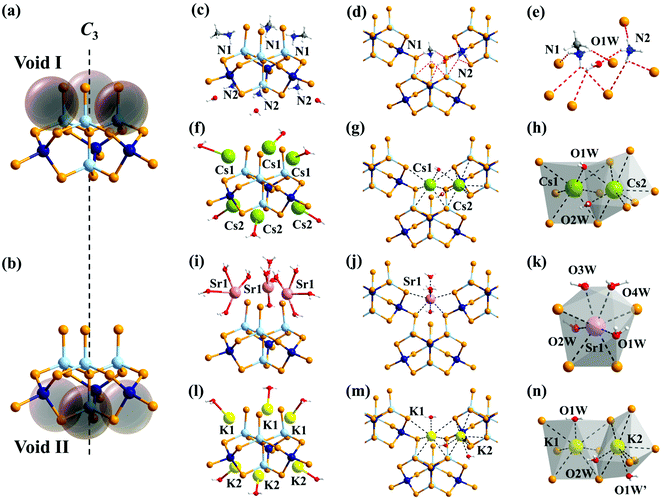 |
| Fig. 6 (a and b) Representations of voids I and II around the mono-lacunary [Cd4Sn3Se16]12− cluster. Positions and coordination environments of ammonium/methylammonium, Cs+, Sr2+ and K+ in (c–e) CdSnSe-1, (f––h) CdSnSe-1Cs, (i–k) CdSnSe-1Sr and (l–n) CdSnSe-1Cs-K/CdSnSe-1Sr-K. | |
Density functional theory (DFT) calculations were further conducted on the crystal structures of exchanged products to explore the efficiency of voids for Cs+ and Sr2+ capture (Fig. 7 and Table S9†). The adsorption energy of each Cs+ at voids I and II was calculated to be −51.95 and −51.79 kcal mol−1, respectively, which indicates a favorable thermodynamic process for the capture of Cs+ by both two nonequivalent sites (Fig. 7b and c). This agrees well with the almost complete substitution of ammonium or methylammonium by Cs+ and the equivalent distribution in quantity over these two sites that is revealed by elemental analysis, TG curves (Fig. S15†) and single-crystal refinement. In comparison, the adsorption energy of Sr2+ at void III was determined to be −160.87 kcal mol−1 (Fig. 7d), which is even higher than the values recorded for Cs+ adsorption. This reveals a stronger affinity of the CdSnSe-1 framework to Sr2+ than Cs+, which certificates the conclusion drawn from the aforementioned exchange kinetic study. It is further reflected by the absence of Cs+ but the small amount of residual Sr2+ in the exchanger upon elution with concentrated K+. Calculations were also conducted on the hypothetical adsorption of Sr2+ in void I or II, but the results cannot converge reasonably, or instead give the fragmentation of the molecules. This suggests the invalidity of original sites occupied by ammonium or methylammonium for the capture of Sr2+, which is probably caused by the stereochemical constraints of the larger hydrated Sr2+ compared with Cs+ and/or the unbalanced charge distribution over a crystal lattice that is not beneficial for stabilization. In one word, the exiting “visualization” of the complete Cs+ and Sr2+ adsorption and K+-elution processes through well-refined single-crystal structures not only underlines the robust and flexible framework of the CdSnSe-1 exchanger, but also supplies important information for the recognition of ionic species varying in size, charge and other physicochemical properties.
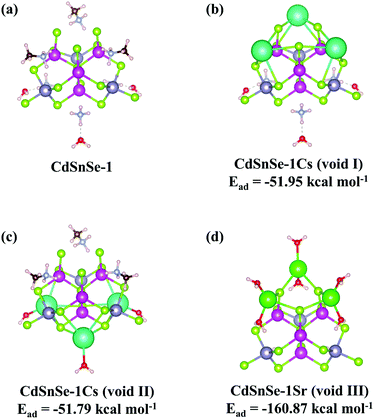 |
| Fig. 7 DFT-modified structures of (a) CdSnSe-1, (b) CdSnSe-1Cs (void I), (c) CdSnSe-1Cs (void II) and (d) CdSnSe-1Sr (void III) showing the position of ammonium/methylammonium, Cs+ and Sr2+ related to the mono-lacunary cluster and the corresponding coordination environments. Extra Cs⋯Se and Sr⋯Se interactions with adjacent clusters are not shown for clarity. | |
Effects of pH on the ion exchange
Because the radioactive Cs+- and Sr2+-contaminated water would be acidic or alkaline, it is highly desirable to investigate the influence of solution pH on the performance of CdSnSe-1. The ion exchange features a bimodal-shape variation of Kd with pH, where the peak values are positioned at pH 5 (1.11 × 104 mL g−1) and 11 (1.42 × 104 mL g−1) for Cs+, and pH 6 (1.96 × 104 mL g−1) and 11 (1.50 × 104 mL g−1) for Sr2+ (Fig. 8a and b). In general, a Kd larger than 104 mL g−1 is evaluated as good for adsorbents, and CdSnSe-1 compares well with some outstanding bi-functional Cs+ and Sr2+ exchangers with Kd values ranging from 103 to 105 mL g−1, such as KMS-1 (KCsd = 2 × 104 mL g−1; KSrd = 1.52 × 105 mL g−1),35,71 KMS-2 (KCsd = 4.56 × 103 mL g−1; KSrd = 1.45 × 105 mL g−1),36 KTS-3 (KCsd = 5.5 × 104 mL g−1; KSrd = 3.9 × 105 mL g−1),37 and FJSM-SnS (KCsd = 2.36 × 103 mL g−1; KSrd = 8.89 × 104 mL g−1).38 The higher Kd values that occur at weakly acidic and alkaline conditions probably stem from the increase in ionic strength. Actually, both KCsd and KSrd remain at a level higher than 3.70 × 103 mL g−1 over pH 4–12, indicating a remarkable acid/base resistance of exchange performance on CdSnSe-1. Especially, CdSnSe-1 still exhibits superior removal efficiencies for Sr2+ at pH 3 (KSrd = 1.18 × 103 mL g−1) and pH 13 (KSrd = 7.46 × 102 mL g−1), outperforming most metal chalcogenide Sr2+ exchangers under highly acidic and alkaline conditions. Powder XRD measurements reveal a good structural stability of CdSnSe-1 at pH 4–13 for Cs+ adsorption and pH 3–13 for Sr2+ adsorption (Fig. S17†). Stronger acidic (pH 0–2) or alkaline (pH 14) environments result in the collapse of the exchanger framework and give black residues (Fig. S18 and S19†), and thus give rise to worse adsorption performances with R < 35.54% for both ions. In addition, the initial solutions at pH 4–10 were finally neutralized to pH 8.5–10.1 for Cs+ and pH 7.9–9.4 for Sr2+, suggesting a buffering role of CdSnSe-1 in accepting or donating protons during the ion exchange processes (Fig. 8a and b).
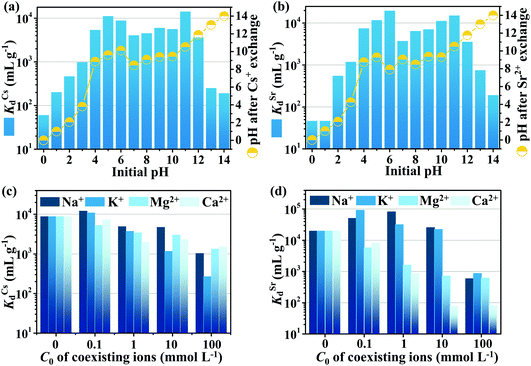 |
| Fig. 8 Variation of Kd with (a and b) solution pH and (c and d) increasing concentrations of coexisting ions (pH ∼6) for Cs+ and Sr2+ ion exchange by CdSnSe-1. The end pH values after exchange are shown as yellow scatters in (a) and (b). Note: C0 ~ 6 ppm, 25 °C, V/m = 1000 mL g−1, contact time = 24 h. | |
Effects of coexisting ions on the ion exchange
The waste solution may contain Na+, K+, Mg2+ and Ca2+ ions that would influence the Cs+, Sr2+ exchange. Fig. 8c and d shows the variation of KCsd and KSrd with the concentration (0.1, 1, 10 and 100 mmol L−1) of each individual coexisting ion. Na+, Mg2+ and Ca2+ exhibit a marginal influence on the Cs+ adsorption, where the KCsd values are kept at higher than 103 mL g−1 even when the concentrations of these ions are increased to 100 mmol L−1. In contrast, the adverse impact of K+ is more significant, as evidenced by the decrease in KCsd to 2.68 × 102 mL g−1 in the presence of K+ (100 mmol L−1). This arises from the comparability of Cs+ and K+ in both hydrated (Cs+, 3.29 Å; K+, 3.31 Å) and structural ionic radii (Cs+, 1.69 Å; K+, 1.33 Å). For Sr2+ adsorption, less concentrated (0.1–10 mmol L−1) Na and K+ ions would not reduce but would raise the KSrd up to a level higher than 2.22 × 104 mL g−1. Especially, the KSrd can even reach 9.11 × 104 and 8.17 × 104 mL g−1 in the presence of 0.1 mmol L−1 K+ and 1 mmol L−1 Na+, respectively. This may be ascribed to the increase in overall ionic strength that is analogous to the observation in the pH effect study. Another possible reason is the charge distinction as Sr2+ is a divalent cation while Na+ and K+ are monovalent cations. In comparison, the uptake of Sr2+ is more sensitively affected by Mg2+ and Ca2+ ions, whose hydrated ionic radii (Mg2+, 4.28 Å; Ca2+, 4.12 Å) are closer to that of Sr2+ (4.12 Å) than those of Na+ (3.58 Å) and K+ (3.31 Å). The KSrd decreases from 1.96 × 104 (blank) to 7.18 × 102 mL g−1 as the concentration of Mg2+ is increased to 10 mmol L−1, and if the Mg2+ is replaced by Ca2+, the KSrd value will decline more sharply to 76.53 mL g−1. The more severe effect of Ca2+ than Mg2+ can be ascribed to its higher similarity to Sr2+ in both structural ionic radius (Mg2+, 0.65 Å; Ca2+, 0.99 Å; Sr2+, 1.13 Å) and chemical properties.
Ion exchange in actual water environments
The Cs+ and Sr2+ (C0 ∼ 6 ppm for each) ion exchange performances of CdSnSe-1 were also explored in mineral water (MW), tap water (TW) and lake water (LW). The experiments performed in deionized water (DW) were adopted as a control (Fig. 9 and Table S14†). CdSnSe-1 displays superior Cs+ adsorption in MW and TW, with corresponding Kd values of 3.43 × 103 mL g−1 (R = 77.41%) and 3.83 × 103 mL g−1 (R = 79.31%), respectively. Even in LW, the Kd value is still kept higher than 1.20 × 103 mL g−1 (R = 54.62%), which can be ascribed to the good resistance of CdSnSe-1 to relatively highly concentrated Na+ (683.05 ppm), Mg2+ (102.80 ppm) and Ca2+ (81.20 ppm). In comparison, the Sr2+ exchange is influenced more significantly by the actual environment. Based on the analysis of water quality, the Ca2+ concentration follows the order of MW (9.88 ppm) < TW (36.3 ppm) < LW (81.20 ppm). 47.81% and 38.83% of Sr2+ can be eliminated from MW and TW, and the removal further drops to 17.05% in LW. This contributes to lower Kd values of 9.16 × 102 (MW), 6.35 × 102 (TW) and 2.06 × 102 mL g−1 (LW) compared with those for Cs+ adsorption.
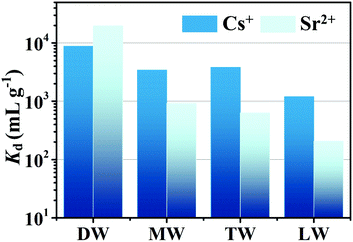 |
| Fig. 9
K
d for Cs+ and Sr2+ ion exchange by CdSnSe-1 performed in DW, MW, TW and LW. | |
Conclusion
Efficient remediation of Cs+ and Sr2+ through the ion exchange method was achieved using a cadmium selenidostannate CdSnSe-1, whose unique open framework constructed from mono-lacunary supertetrahedral clusters facilitates the systematic investigation of capture and elution mechanism at the molecular level. CdSnSe-1 exhibits rapid ion exchange kinetics, wide pH durability, high exchange selectivity and β and γ radiation resistance. Particularly, the [Cd4Sn3Se13]n6n− framework features a large negative charge density of 3.27 × 10−3 that accounts for the superhigh exchange capacities of 371.4 mg g−1 for Cs+ and 128.4 mg g−1 for Sr2+. Moreover, the facile elution of adsorbed Cs+ and Sr2+ ions by K+ endows this material with a promising potential for recycling. Single-crystal structural analysis on the solid at different stages in the “pristine–exchange–elution” cycle supplies instructive information to clarify the uptake and recycle mechanism for Cs+ and Sr2+. The co-templating effects of mixed methylammonium/ammonium and the broken symmetry of the cluster caused by the vacant site contribute to the formation of two types of voids (I and II) that show adsorption activity for both monovalent Cs+ and K+ ions. In comparison, the divalent Sr2+ ions with a higher hydration degree exchange with the (alkyl)ammonium cations in a 1
:
2 molar ratio, resulting in their location at a new void (III) closer to the framework and thus higher binding strength. DFT calculations performed on the well-refined crystal structures illustrate the efficiency of CdSnSe-1 for Cs+ and Sr2+ capture according to the energy variation during the adsorption process. In one word, the “visualized” mechanism research presented here sheds light on a deeper understanding of the structure–function relationship for materials, which is of great significance for methodological innovation in radionuclide remediation.
Conflicts of interest
There are no conflicts to declare.
Acknowledgements
This work was financially supported by the National Natural Science Foundation of China (21701123), the Foundation Enhancement Program (2021-JCJQ-JJ-1061), the Fund of the State Key Laboratory of Structural Chemistry (20190009), and the Fund of the National Engineering Research Center for Optoelectronic Crystalline Materials (OCM-2020-02).
Notes and references
- M. Aoyagi, The impact of the Fukushima accident on nuclear power policy in Japan, Nat. Energy, 2021, 6, 326–328 CrossRef.
- T. Ohba, K. Tanigawa and L. Liutsko, Evacuation after a nuclear accident: Critical reviews of past nuclear accidents and proposal for future planning, Environ. Int., 2021, 148, 106379 CrossRef CAS PubMed.
- P. C. Burns, R. C. Ewing and A. Navrotsky, Nuclear fuel in a reactor accident, Science, 2012, 335, 1184–1188 CrossRef CAS PubMed.
- H. Tazoe, T. Yamagata, K. Tsujita, H. Nagai, H. Obata, D. Tsumune, J. Kanda and M. Yamada, Observation of dispersion in the Japanese coastal area of released 90Sr, 134Cs, and 137Cs from the Fukushima Daiichi nuclear power plant to the sea in 2013, Int. J. Environ. Res. Public Health, 2019, 16, 4094 CrossRef CAS PubMed.
- A. Konoplev, Y. Wakiyama, T. Wada, C. Udy, V. Kanivets, M. M. Ivanov, M. Komissarov, T. Takase, A. Goto and K. Nanba, Radiocesium distribution and mid-term dynamics in the ponds of the Fukushima Dai-ichi nuclear power plant exclusion zone in 2015–2019, Chemosphere, 2021, 265, 129058 CrossRef CAS PubMed.
- N. Kinoshita, K. Sueki, K. Sasa, J.-I. Kitagawa, S. Ikarashi, T. Nishimura, Y.-S. Wong, Y. Satou, K. Handa, T. Takahashi, M. Sato and T. Yamagata, Assessment of individual radionuclide distributions from the Fukushima nuclear accident covering central-east Japan, Proc. Natl. Acad. Sci. U. S. A., 2011, 108, 19526–19529 CrossRef CAS PubMed.
- K. Sakuma, K. Yoshimura and T. Nakanishi, Leaching characteristics of 137Cs for forest floor affected by the Fukushima nuclear accident: A litterbag experiment, Chemosphere, 2021, 264, 128480 CrossRef CAS PubMed.
- W. Men, F. Wang, W. Yu, J. He, F. Lin, F. Deng, T. Yu, H. Ma and Z. Zeng, Radioactive impacts of the Fukushima Dai-ichi nuclear power plant accident on blue sharks in the Northwest Pacific, Chemosphere, 2021, 285, 131537 CrossRef CAS PubMed.
- D. Alby, C. Charnay, M. Heran, B. Prelot and J. Zajac, Recent developments in nanostructured inorganic materials for sorption of cesium and strontium: Synthesis and shaping, sorption capacity, mechanisms, and selectivity – A review, J. Hazard. Mater., 2018, 344, 511–530 CrossRef CAS PubMed.
- B. Ding, Z. Wang, X. Wang, W. Yang, S. Wang, C. Li, H. Dai and S. Tao, Sr2+ adsorbents produced by microfluidics, Colloids Surf., A, 2021, 613, 126072 CrossRef CAS.
- M. Castrillejo, N. Casacuberta, C. F. Breier, S. M. Pike, P. Masqué and K. O. Buesseler, Reassessment of 90Sr, 137Cs, and 134Cs in the coast off Japan derived from the Fukushima Dai-ichi nuclear accident, Environ. Sci. Technol., 2016, 50, 173–180 CrossRef CAS.
- X.-Y. Zhou, D. A. Sun, Y. Tan and A. Zhou, Canister spacing in high-level radioactive nuclear waste repository, Ann. Nucl. Energy, 2020, 141, 107335 CrossRef CAS.
- O. Artun, A study of nuclear structure for 244Cm, 241Am, 238Pu, 210Po, 147Pm, 137Cs, 90Sr and 63Ni nuclei used in nuclear battery, Mod. Phys. Lett. A, 2017, 32, 1750117 CrossRef CAS.
- H. Yoshikawa, S. Sunada, H. Hirakawa, A. Fujimori, S. Elmegerhi, D. Leary and T. A. Kato, Radiobiological characterization of canine malignant melanoma cell lines with different types of ionizing radiation and efficacy evaluation with cytotoxic agents, Int. J. Mol. Sci., 2019, 20, 841 CrossRef CAS.
- M. Huang, R. G. Hill and S. C. F. Rawlinson, Strontium (Sr) elicits odontogenic differentiation of human dental pulp stem cells (hDPSCs): A therapeutic role for Sr in dentine repair?, Acta Biomater., 2016, 38, 201–211 CrossRef CAS PubMed.
- S.-M. Kim, I.-H. Yoon, I. Kim, J.-H. Kim and S.-J. Park, Hydrothermal desorption of Cs with oxalic acid from hydrobiotite and wastewater treatment by chemical precipitation, Energies, 2020, 13, 3284 CrossRef CAS.
- D. Fang, Y. Wang, H. Liu, H. Zhang, X. Ye, Q. Li, J. Li and Z. Wu, Efficient extraction of Rb+ and Cs+ by a precipitation flotation process with ammonium phosphowolframate as precipitant, Colloids Surf., A, 2021, 608, 125581 CrossRef CAS.
- P. Vaňura, P. Selucký and Z. Asfari, Towards selective separations of monovalent cations by solvent extraction of their calix[4]crown complexes in water–nitrobenzene systems, J. Mol. Liq., 2021, 336, 116357 CrossRef.
- K. Patra, B. Sadhu, A. Sengupta, C. B. Patil, R. K. Mishra and C. P. Kaushik, Achieving highly efficient and selective cesium extraction using 1,3-di-octyloxycalix[4]arene-crown-6 in n-octanol based solvent system: experimental and DFT investigation, RSC Adv., 2021, 11, 21323–21331 RSC.
- F. Ma, Z. Li, H. Zhao, Y. Geng, W. Zhou, Q. Li and L. Zhang, Potential application of graphene oxide membranes for removal of Cs(I) and Sr(II) from high level-liquid waste, Sep. Purif. Technol., 2017, 188, 523–529 CrossRef CAS.
- R. K. Vishwakarma, P. K. Narayanam, R. Umamaheswari and K. Sundararajan, Surface modified and functionalized graphene oxide membranes for separation of strontium from aqueous solutions, J. Environ. Manage., 2021, 298, 113443 CrossRef CAS PubMed.
- S. Ghorbanzadeh Mashkani and P. Tajer Mohammad Ghazvini, Biotechnological potential of Azolla filiculoides for biosorption of Cs and Sr: Application of micro-PIXE for measurement of biosorption, Bioresour. Technol., 2009, 100, 1915–1921 CrossRef PubMed.
- Y. Hu, X. Guo and J. Wang, Biosorption of Sr2+ and Cs+ onto Undaria pinnatifida: Isothermal titration calorimetry and molecular dynamics simulation, J. Mol. Liq., 2020, 319, 114146 CrossRef CAS.
- Y. Sun, D. Shao, C. Chen, S. Yang and X. Wang, Highly efficient enrichment of radionuclides on graphene oxide-supported polyaniline, Environ. Sci. Technol., 2013, 47, 9904–9910 CrossRef CAS.
- K. Jin, B. Lee and J. Park, Metal-organic frameworks as a versatile platform for radionuclide management, Coord. Chem. Rev., 2021, 427, 213473 CrossRef CAS.
- C. W. Kim, N. H. Heo and K. Seff, Framework sites preferred by aluminum in zeolite ZSM-5. Structure of a fully dehydrated, fully Cs+-exchanged ZSM-5 crystal (MFI, Si/Al=24), J. Phys. Chem. C, 2011, 115, 24823–24838 CrossRef CAS.
- Y. Li, A. O. Simon, C. Jiao, M. Zhang, W. Yan, H. Rao, J. Liu and J. Zhang, Rapid removal of Sr2+, Cs+ and UO22+ from solution with surfactant and amino acid modified zeolite Y, Microporous Mesoporous Mater., 2020, 302, 110244 CrossRef CAS.
- Y. Kim, Y. K. Kim, J. H. Kim, M.-S. Yim, D. Harbottle and J. W. Lee, Synthesis of functionalized porous montmorillonite via solid-state NaOH treatment for efficient removal of cesium and strontium ions, Appl. Surf. Sci., 2018, 450, 404–412 CrossRef CAS.
- A. S. Semenkova, M. V. Evsiunina, P. K. Verma, P. K. Mohapatra, V. G. Petrov, I. F. Seregina, M. A. Bolshov, V. V. Krupskaya, A. Y. Romanchuk and S. N. Kalmykov, Cs+ sorption onto Kutch clays: Influence of competing ions, Appl. Clay Sci., 2018, 166, 88–93 CrossRef CAS.
- N. Döbelin and T. Armbruster, Microporous titanosilicate AM-2: Ion-exchange and thermal stability, Microporous Mesoporous Mater., 2007, 99, 279–287 CrossRef.
- D. Ding, K. Li, D. Fang, X. Ye, Y. Hu, X. Tan, H. Liu and Z. Wu, Novel biomass-derived adsorbents grafted sodium titanium silicate with high adsorption capacity for Rb+ and Cs+ in the Brine, ChemistrySelect, 2019, 4, 13630–13637 CrossRef CAS.
- A. J. Cramer and J. M. Cole, Topological analysis of void space in phosphate frameworks: Assessing storage properties for the environmentally important guest molecules and ions: CO2, H2O, UO2, PuO2, U, Pu, Sr2+, Cs+, CH4, and H2, ACS Sustainable Chem. Eng., 2016, 4, 4094–4112 CrossRef CAS.
- A. Ivanets, V. Milyutin, I. Shashkova, N. Kitikova, N. Nekrasova and A. Radkevich, Sorption of stable and radioactive Cs(I), Sr(II), Co(II) ions on Ti–Ca–Mg phosphates, J. Radioanal. Nucl. Chem., 2020, 324, 1115–1123 CrossRef CAS.
- A. L. Hames, P. Tkac, A. Paulenova, J. L. Willit and M. A. Williamson, Investigation of molybdate melts as an alternative method of reprocessing used nuclear fuel, J. Nucl. Mater., 2017, 486, 158–166 CrossRef CAS.
- M. J. Manos and M. G. Kanatzidis, Highly efficient and rapid Cs+ uptake by the layered metal sulfide K2xMnxSn3−xS6 (KMS-1), J. Am. Chem. Soc., 2009, 131, 6599–6607 CrossRef CAS.
- J. L. Mertz, Z. H. Fard, C. D. Malliakas, M. J. Manos and M. G. Kanatzidis, Selective removal of Cs+, Sr2+, and Ni2+ by K2xMgxSn3–xS6 (x=0.5–1) (KMS-2) relevant to nuclear waste remediation, Chem. Mater., 2013, 25, 2116–2127 CrossRef CAS.
- D. Sarma, C. D. Malliakas, K. S. Subrahmanyam, S. M. Islam and M. G. Kanatzidis, K2xSn4−xS8−x (x=0.65–1): A new metal sulfide for rapid and selective removal of Cs+, Sr2+ and UO22+ ions, Chem. Sci., 2016, 7, 1121–1132 RSC.
- X.-H. Qi, K.-Z. Du, M.-L. Feng, J.-R. Li, C.-F. Du, B. Zhang and X.-Y. Huang, A two-dimensionally microporous thiostannate with superior Cs+ and Sr2+ ion-exchange property, J. Mater. Chem. A, 2015, 3, 5665–5673 RSC.
- K.-Y. Wang, D. Ding, M. Sun, L. Cheng and C. Wang, Effective and rapid adsorption of Sr2+ ions by a hydrated pentasodium cluster templated zinc thiostannate, Inorg. Chem., 2019, 58, 10184–10193 CrossRef CAS PubMed.
- K.-Y. Wang, M. Sun, D. Ding, H.-W. Liu, L. Cheng and C. Wang, Di-lacunary [In6S15]12− cluster: Building block of a highly negative charged framework for superior Sr2+ adsorption capacities, Chem. Commun., 2020, 56, 3409–3412 RSC.
- M. Sun, K.-Y. Wang, D. Ding, J.-Y. Zhu, Y.-M. Zhao, L. Cheng and C. Wang, Removal of Sr2+ ions by a high-capacity indium sulfide exchanger containing permeable layers with large pores, Inorg. Chem., 2020, 59, 13822–13826 CrossRef CAS PubMed.
- M. Zhang, P. Gu, Z. Zhang, J. Liu, L. Dong and G. Zhang, Effective, rapid and selective adsorption of radioactive Sr2+ from aqueous solution by a novel metal sulfide adsorbent, Chem. Eng. J., 2018, 351, 668–677 CrossRef CAS.
- Z. Zhang, P. Gu, M. Zhang, S. Yan, L. Dong and G. Zhang, Synthesis of a robust layered metal sulfide for rapid and effective removal of Sr2+ from aqueous solutions, Chem. Eng. J., 2019, 372, 1205–1215 CrossRef CAS.
- Y.-M. Zhao, L. Cheng, K.-Y. Wang, X. Hao, J. Wang, J.-Y. Zhu, M. Sun and C. Wang, pH-Controlled switch over coadsorption and separation for mixed Cs+ and Sr2+ by an acid-resistant potassium thioantimonate, Adv. Funct. Mater., 2022, 2112717 CrossRef.
- X.-M. Zhang, D. Sarma, Y.-Q. Wu, L. Wang, Z.-X. Ning, F.-Q. Zhang and M. G. Kanatzidis, Open-framework oxysulfide based on the supertetrahedral [In4Sn16O10S34]12– cluster and efficient sequestration of heavy metals, J. Am. Chem. Soc., 2016, 138, 5543–5546 CrossRef CAS PubMed.
- L. Wang, H. Pei, D. Sarma, X.-M. Zhang, K. MacRenaris, C. D. Malliakas and M. G. Kanatzidis, Highly selective radioactive 137Cs+ capture in an open-framework oxysulfide based on supertetrahedral cluster, Chem. Mater., 2019, 31, 1628–1634 CrossRef CAS.
- N. Ding and M. G. Kanatzidis, Acid-induced conversions in open-framework semiconductors: From [Cd4Sn3Se13]6− to [Cd15Sn12Se46]14−, a remarkable disassembly/reassembly process, Angew. Chem., Int. Ed., 2006, 45, 1397–1401 CrossRef CAS PubMed.
- H.-W. Liu, K.-Y. Wang, D. Ding, M. Sun, L. Cheng and C. Wang, Deep eutectic solvothermal synthesis of an open framework copper selenidogermanate with pH-resistant Cs+ ion exchange properties, Chem. Commun., 2019, 55, 13884–13887 RSC.
- D. Ding, L. Cheng, K.-Y. Wang, H.-W. Liu, M. Sun and C. Wang, Efficient Cs+–Sr2+ separation over a microporous silver selenidostannate synthesized in deep eutectic solvent, Inorg. Chem., 2020, 59, 9638–9647 CrossRef CAS PubMed.
- J.-H. Tang, J.-C. Jin, W.-A. Li, X. Zeng, W. Ma, J.-L. Li, T.-T. Lv, Y.-C. Peng, M.-L. Feng and X.-Y. Huang, Highly selective cesium(I) capture under acidic conditions by a layered sulfide, Nat. Commun., 2022, 13, 658 CrossRef CAS PubMed.
- Y.-Y. Liao, J.-R. Li, B. Zhang, H.-Y. Sun, W. Ma, J.-C. Jin, M.-L. Feng and X.-Y. Huang, Robust and flexible thioantimonate materials for Cs+ remediation with distinctive structural transformation: A clear insight into the ion-exchange mechanism, ACS Appl. Mater. Interfaces, 2021, 13, 5275–5283 CrossRef CAS PubMed.
-
Reflectance Spectroscopy, ed. W. M. Wendlandt and H. G. Hecht, Interscience, New York, 1966 Search PubMed.
- G. M. Sheldrick, A short history of SHELX, Acta Crystallogr., Sect. A: Found. Crystallogr., 2008, 64, 112–122 CrossRef CAS PubMed.
- G. Kresse and J. Furthmüller, Efficient iterative schemes for ab initio total-energy calculations using a plane-wave basis set, Phys. Rev. B: Condens. Matter Mater. Phys., 1996, 54, 11169–11186 CrossRef CAS PubMed.
- G. Kresse and D. Joubert, From ultrasoft pseudopotentials to the projector augmented-wave method, Phys. Rev. B: Condens. Matter Mater. Phys., 1999, 59, 1758–1775 CrossRef CAS.
- J. P. Perdew, K. Burke and M. Ernzerhof, Generalized gradient approximation made simple, Phys. Rev. Lett., 1996, 77, 3865–3868 CrossRef CAS PubMed.
- K.-Y. Wang, D. Ding, S. Zhang, Y. Wang, W. Liu, S. Wang, S.-H. Wang, D. Liu and C. Wang, Preparation of thermochromic selenidostannates in deep eutectic solvents, Chem. Commun., 2018, 54, 4806–4809 RSC.
- K.-Y. Wang, H.-W. Liu, S. Zhang, D. Ding, L. Cheng and C. Wang, Selenidostannates and a silver selenidostannate synthesized in deep eutectic solvents: crystal structures and thermochromic study, Inorg. Chem., 2019, 58, 2942–2953 CrossRef CAS PubMed.
- K.-Y. Wang, S. Zhang, H.-W. Liu, L. Cheng and C. Wang, Stepwise conversion from GeO2 to [MGe4S10]n3n– (M=Cu, Ag) polymer via isolatable [Ge2S6]4– and [Ge4S10]4– anions by virtue of templating technique, Inorg. Chem., 2019, 58, 12832–12842 CrossRef CAS PubMed.
- M. Sun, S. Zhang, K.-Y. Wang, J. Wang, L. Cheng, J.-Y. Zhu, Y.-M. Zhao and C. Wang, Mixed solvothermal synthesis of tn cluster-based indium and gallium sulfides using versatile ammonia or amine structure-directing agents, Inorg. Chem., 2021, 60, 7115–7127 CrossRef CAS PubMed.
- S. Zhang, M. Sun, K.-Y. Wang, L. Cheng, S. Zhang and C. Wang, Conversion of organically directed selenidostannate into porous SnO2 exhibiting effective electrochemical reduction of CO2 to C1 products, ACS Sustainable Chem. Eng., 2021, 9, 2358–2366 CrossRef CAS.
- N. Ding, D.-Y. Chung and M. G. Kanatzidis, K6Cd4Sn3Se13: A polar open-framework compound based on the partially destroyed supertetrahedral [Cd4Sn4Se17]10− cluster, Chem. Commun., 2004, 1170–1171 RSC.
- M. Wu, W. Su, N. Jasutkar, X. Huang and J. Li, An open-framework bimetallic chalcogenide structure K3Rb3Zn4Sn3Se13 built on a unique [Zn4Sn3Se16]12− cluster: Synthesis, crystal structure, ion exchange and optical properties, Mater. Res. Bull., 2005, 40, 21–27 CrossRef CAS.
- E. R. Nightingale, Phenomenological theory of ion solvation. Effective radii of hydrated ions, J. Phys. Chem., 1959, 63, 1381–1387 CrossRef CAS.
- A.-A. M. Abdel-Karim, A. A. Zaki, W. Elwan, M. R. El-Naggar and M. M. Gouda, Experimental and modeling investigations of cesium and strontium adsorption onto clay of radioactive waste disposal, Appl. Clay Sci., 2016, 132–133, 391–401 CrossRef CAS.
- A. M. El-Kamash, Evaluation of zeolite A for the sorptive removal of Cs+ and Sr2+ ions from aqueous solutions using batch and fixed bed column operations, J. Hazard. Mater., 2008, 151, 432–445 CrossRef CAS PubMed.
- Z. Zheng, C. V. Philip, R. G. Anthony, J. L. Krumhansl, D. E. Trudell and J. E. Miller, Ion exchange of group I metals by hydrous crystalline silicotitanates, Ind. Eng. Chem. Res., 1996, 35, 4246–4256 CrossRef CAS.
- S. Seino, R. Kawahara, Y. Ogasawara, N. Mizuno and S. Uchida, Reduction-induced highly selective uptake of cesium ions by an ionic crystal based on silicododecamolybdate, Angew. Chem., Int. Ed., 2016, 55, 3987–3991 CrossRef CAS PubMed.
- S. Naeimi and H. Faghihian, Performance of novel adsorbent prepared by magnetic metal-organic framework (MOF) modified by potassium nickel hexacyanoferrate for removal of Cs+ from aqueous solution, Sep. Purif. Technol., 2017, 175, 255–265 CrossRef CAS.
- M. Arshadi, M. J. Amiri and S. Mousavi, Kinetic, equilibrium and thermodynamic investigations of Ni(II), Cd(II), Cu(II) and Co(II) adsorption on barley straw ash, Water Resour. Ind., 2014, 6, 1–17 CrossRef.
- M. J. Manos, N. Ding and M. G. Kanatzidis, Layered metal sulfides: Exceptionally selective agents for radioactive strontium removal, Proc. Natl. Acad. Sci. U. S. A., 2008, 105, 3696 CrossRef CAS PubMed.
- S. Sarina, A. Bo, D. Liu, H. Liu, D. Yang, C. Zhou, N. Maes, S. Komarneni and H. Zhu, Separate or simultaneous removal of radioactive cations and anions from water by layered sodium vanadate-based sorbents, Chem. Mater., 2014, 26, 4788–4795 CrossRef CAS.
- J. Zhang, L. Chen, X. Dai, L. Zhu, C. Xiao, L. Xu, Z. Zhang, E. V. Alekseev, Y. Wang, C. Zhang, H. Zhang, Y. Wang, J. Diwu, Z. Chai and S. Wang, Distinctive two-step intercalation of Sr2+ into a coordination polymer with record high 90Sr uptake capabilities, Chem, 2019, 5, 977–994 CAS.
- W. Ma, B. Hu, J.-L. Li, Z.-Z. Zhang, X. Zeng, J. Jin, Z. Li, S.-T. Zheng, M.-L. Feng and X.-Y. Huang, The uptake of hazardous metal ions into a high-nuclearity cluster-based compound with structural transformation and proton conduction, ACS Appl. Mater. Interfaces, 2020, 12, 26222–26231 CrossRef CAS PubMed.
- J. Li, J. Jin, T. Zhang, W. Ma, X. Zeng, H. Sun, M. Cheng, M. Feng and X. Huang, Rapid and selective uptake of Cs+ and Sr2+ ions by a layered thiostannate with acid–base and irradiation resistances, ACS ES&T Water, 2021, 1, 2440–2449 Search PubMed.
- M. J. Manos, C. D. Malliakas and M. G. Kanatzidis, Heavy-metal-ion capture, ion-exchange, and exceptional acid stability of the open-framework chalcogenide [NH4]4In12Se20, Chem. – Eur. J., 2007, 13, 51–58 CrossRef CAS PubMed.
- M. J. Manos, K. Chrissafis and M. G. Kanatzidis, Unique pore selectivity for Cs+ and exceptionally high NH4+ exchange capacity of the chalcogenide material K6Sn[Zn4Sn4S17], J. Am. Chem. Soc., 2006, 128, 8875–8883 CrossRef CAS PubMed.
- K.-Y. Wang, M.-L. Feng, J.-R. Li and X.-Y. Huang, [NH3CH3]4[In4SbS9SH]: A novel methylamine-directed indium thioantimonate with Rb+ ion-exchange property, J. Mater. Chem. A, 2013, 1, 1709–1715 RSC.
- N. Ding and M. G. Kanatzidis, Selective incarceration of caesium ions by Venus flytrap action of a flexible framework sulfide, Nat. Chem., 2010, 2, 187–191 CrossRef CAS PubMed.
- Y.-M. Zhao, M. Sun, L. Cheng, K.-Y. Wang, Y. Liu, J.-Y. Zhu, S. Zhang and C. Wang, Efficient removal of Ba2+, Co2+ and Ni2+ by an ethylammonium-templated indium sulfide ion exchanger, J. Hazard. Mater., 2022, 425, 128007 CrossRef CAS PubMed.
Footnotes |
† Electronic supplementary information (ESI) available: Powder XRD patterns, FTIR, EDS, mapping, UV–vis reflectance spectra and TG analysis on the pristine, exchanged and eluted products. CCDC 2133519–2133523 (for CdSnSe-1, CdSnSe-1Cs, CdSnSe-1Sr, CdSnSe-1Cs-K and CdSnSe-1Sr-K). For the ESI and crystallographic data in CIF or another electronic format see DOI: https://doi.org/10.1039/d2qi00338d |
‡ These authors contributed equally to this work. |
|
This journal is © the Partner Organisations 2022 |