DOI:
10.1039/D2QI00023G
(Research Article)
Inorg. Chem. Front., 2022,
9, 1504-1513
Temperature- and solvent-induced reversible single-crystal-to-single-crystal transformations of TbIII-based MOFs with excellent stabilities and fluorescence sensing properties toward drug molecules†
Received
4th January 2022
, Accepted 9th February 2022
First published on 9th February 2022
Abstract
Recently, single-crystal-to-single-crystal conversion has been a hot topic in the field of metal–organic framework (MOF) materials, which could improve the stability and properties due to structural changes. A new TbIII-based MOF ([Tb4(BTDI)3(DMF)4]n, JXUST-12) has been designed and synthesized by the incorporation of TbIII ions and H4BTDI ligands (5,5′-(benzo[c][1,2,5]thiadiazole-4,7-diyl)diisophthalic acid) featuring a fluorescent unit. Very interestingly, the reversible single-crystal-to-single-crystal transformation between JXUST-12 and {[Tb4(BTDI)3(H2O)4]·4H2O·solvents}n (JXUST-12a) occurred, and thus the enhancement of water and pH stabilities has been achieved. Because of the presence of TbIII ions and the fluorescent organic ligand, JXUST-12 and JXUST-12a can be used as luminescent sensors for the detection of drugs (NFT = nitrofurantoin, NZF = nitrofurazone, and DCN = 2,6-dichloro-4-nitroaniline). Furthermore, theoretical caculations and experimental studies suggest that the luminescence quenching of JXUST-12 and JXUST-12a for the detection of NFT, NZF and DCN may be attributed to a competitive energy absorption and photoinduced electron transfer mechanism. Remarkably, fluorescent test papers and composite films based on JXUST-12 and JXUST-12a have been developed to simply detect drugs through the visual change of the luminescence colour.
Introduction
Metal–organic frameworks (MOFs) have potential application prospects in the fields of sensing,1,2 catalysis,3 magnetism,4 proton conduction5 and gas adsorption and separation6,7 due to the abundant topological networks,8 high specific surface area9 and adjustable optical properties.10 Single-crystal-to-single-crystal (SCSC) conversion is an effective way to reconstruct the structures of MOFs11 accompanied by the changes of coordination bonds, dimensions and porosity.12–15 Interestingly, SCSC conversion can improve the stability16,17 and luminescence performance18 of MOFs. Luminescent metal–organic frameworks (LMOFs) have been proven to be one of the most promising luminescence sensing materials because of the expected luminescence features.1,19–21 In addition, the aqueous stability of LMOFs is important for exploring their sensing properties.17
Drugs have brought us great advantages in the prevention and treatment of human, animal and plant infectious diseases, but the abuse of drugs can cause harm to the environment and human health.22–26 For example, nitrofuran drugs including nitrofurantoin (NFT) and nitrofurazone (NZF), and 2,6-dichloro-4-nitroaniline (DCN) in organochlorine pesticides are highly toxic and non-degradable, increasing the risk of human cell mutation and carcinogenicity.27–30 In addition, ofloxacin (OFX) and fleroxacin (FO) in fluoroquinolone drugs can affect the stomach, skin and central nervous system of the human body.31–33 The traditional detection methods (high performance liquid chromatography-mass spectrometry and spectrophotometry) are complicated and of high cost.22,34,35 Therefore, there is an urgent need for simple, rapid and efficient detection of the drugs. LMOF sensing can be used as a method to detect drugs due to their advantages in terms of definite fluorescence spectra, visible signals by the naked eye, high sensitivity and selectivity, and simplicity in operation.36–38
2,1,3-Benzothiadiazole (BTD) is a chromophore and an electron withdrawing group, with rich photophysical and photochemical properties, and its derivatives have broad prospects in the field of molecular luminescent probes.39–42 Herein, 5,5′-(benzo[c][1,2,5]thiadiazole-4,7-diyl)diisophthalic acid (H4BTDI) was chosen as the primary ligand and a three-dimensional (3D) TbIII-based MOF ({[Tb4(BTDI)3(H2O)4]·4H2O·solvents}n, JXUST-12a) was obtained by the reversible conversion of [Tb4(BTDI)3(DMF)4]n (JXUST-12) under the heating conditions of 410 °C. In this work, the enhancement of water stability of the LMOF has been achieved by the SCSC transformation and the reversible conversion of the LMOF makes it more convenient and less expensive to sense harmful substances. JXUST-12 and JXUST-12a were used as luminescent probes to detect drug molecules. Notably, JXUST-12a exhibits excellent water and pH stabilities as well as fluorescence sensing properties toward NFT, NZF, DCN, OFX and FO. The limits of detection (LODs) of JXUST-12a for the detection of NFT and NZF are lower than that of JXUST-12. In addition, the sensing mechanisms of JXUST-12 and JXUST-12a were also investigated in detail.
Experimental section
Synthesis of [Tb4(BTDI)3(DMF)4]n (JXUST-12)
JXUST-12 was solvothermally synthesized based on a mixture of Tb(NO3)3·6H2O (22.7 mg, 0.05 mmol), H4BTDI (11.6 mg, 0.025 mmol), 4,4′-bipyridine (15.6 mg, 0.1 mmol), N,N′-dimethylformamide (DMF, 7 mL), formic acid (500 μL) and water (3 mL). The mixture was stirred for 30 minutes and put into a 25 mL Teflon-lined autoclave, which was heated to 160 °C for 72 h. After cooling to room temperature, yellow block crystals were collected with 65.71% yield based on H4BTDI. Elemental analysis (%) calcd for C78H52N10S3Tb4O28: C, 40.57; H, 2.27; N, 6.07; S, 4.17. Found: C, 39.85; H, 2.38; N, 5.69; S, 3.81. IR (KBr, cm−1): 3061w, 1662s, 1617s, 1534s, 1447s, 1395s, 1114w, 845m, 782m, 754m, 713m, 674w, 529w, 434w (Fig. S2, ESI†).
Reversible transformations between JXUST-12 and {[Tb4(BTDI)3(H2O)4]·4H2O·solvents}n (JXUST-12a)
When the TG-DTA analyzer was heated at 410 °C for 15 minutes, JXUST-12 transformed to JXUST-12a by replacing two coordinated DMF molecules with two water molecules without losing crystallinity. Remarkably, the block crystals of JXUST-12a could gradually change to JXUST-12′ (the same structure of JXUST-12) without losing crystallinity when JXUST-12a was heated at 160 °C to reflux in 10 mL DMF solution for 3 days (Fig. 1). Elemental analysis (%) calcd for C78H52N10S3Tb4O28: C, 37.31; H, 1.71; N, 3.96; S, 4.53. Found: C, 35.11; H, 2.42; N, 4.01; S, 4.56. IR (KBr pellets, cm−1): 3612w, 3420w, 3063w, 1640s, 1530s, 1451s, 1397s, 1118w, 853w, 757m, 715m, 533w, 437w (Fig. S2, ESI†).
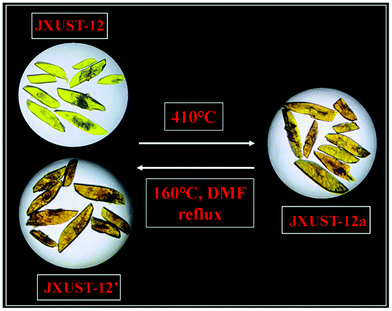 |
| Fig. 1 Photographs of the reversible transformations between JXUST-12 and JXUST-12a in natural light. | |
Results and discussion
Structural description of JXUST-12 and JXUST-12a
Single-crystal X-ray diffraction studies suggest that JXUST-12 and JXUST-12a crystallize in the triclinic system with the P
space group. The asymmetric unit of JXUST-12 contains two crystallographically independent TbIII ions, one and a half BTDI4− ligands and two DMF molecules (Fig. 2a). The asymmetric unit of JXUST-12a is similar to that of JXUST-12 except for two coordinated and two lattice H2O molecules (Fig. 2b). In JXUST-12, seven-coordinate Tb1 and Tb2 present a capped trigonal prism coordination configuration calculated using the SHAPE 2.143 software (Table S4 and Fig. S1c, ESI†). The coordination configuration of Tb2 in JXUST-12a is the same as that of Tb2 in JXUST-12 (Table S5 and Fig. S1d, ESI†), while the coordination configuration of eight-coordinate Tb1 in JXUST-12a is square antiprism (Table S5 and Fig. S1d, ESI†). The coordination modes of carboxylate groups in JXUST-12 are syn–syn-μ2-η1:η1, syn-anti-μ2-η1:η1 and μ-η1:η1 (Fig. S1a, ESI†), while the coordination modes of carboxylate groups in JXUST-12a are similar to those of JXUST-12 except that syn–anti-μ2-η1:η1 is replaced by syn–anti-μ2-η1:η2 (Fig. S1b, ESI†). As shown in Fig. 2a and b, Tb1 of JXUST-12 and JXUST-12a is surrounded by six or seven O atoms from the carboxylate groups of five different BTDI4− ligands and one O atom from DMF or H2O, while Tb2 is coordinated with six O atoms from five distinct BTDI4− ligands and one O atom from DMF or H2O. The adjacent Tb1 and Tb2 are bridged by carboxylate O atoms from BTDI4− ligands to constitute a [Tb2(COO)2] secondary building unit, which is further linked by BTDI4− ligands to form a three-dimensional structure (Fig. 2c and d).
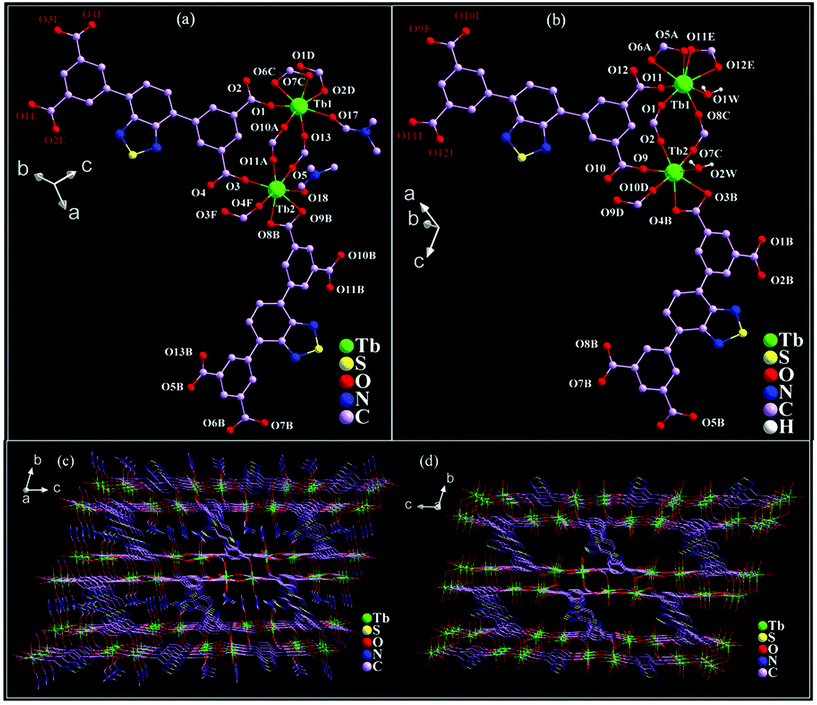 |
| Fig. 2 (a) Ball-and-stick view of the coordination environments of TbIII in JXUST-12 (symmetry codes: A, x, y, z − 1; B, x + 1, y, z − 1; C, x − 1, y, z; D, −x, −y + 1, −z + 1; E, −x + 1, −y + 1, −z). (b) Ball-and-stick view of the coordination environments of TbIII in JXUST-12a (symmetry codes: A, x, y, z − 1; B, x − 1, y, z; C, x − 1, y, z − 1; D, −x, −y + 1, −z; E, −x, −y + 1, −z − 1; F, −x + 1, −y + 2, −z). (c) The 3D structure of JXUST-12. (d) The 3D structure of JXUST-12a. | |
Stability of JXUST-12 and JXUST-12a
As presented in Fig. S3a and S3c (ESI†), the peaks in the PXRD patterns of the as-synthesized samples of JXUST-12 and JXUST-12a are in good agreement with the simulated ones. Both JXUST-12 and JXUST-12a exhibit excellent solvent stability toward common solvents (Fig. S3b and S3d, ESI†). Notably, JXUST-12a exhibits excellent water and pH stabilities (Fig. S3c and S3e, ESI†). When the crystal sample of JXUST-12a was immersed in water at room temperature for a week and stored in boiling water for 24 h, the framework still remained stable. The PXRD patterns of JXUST-12a after soaking in aqueous solutions with pH values of 1–12 are in good agreement with the simulated one, indicating that JXUST-12a has excellent pH stability. The size and morphology of JXUST-12 and JXUST-12a were characterized by the scanning electron microscopy method (Fig. S5, ESI†). The results show a rectangular block structure with an average particle size of 0.32 mm and 0.23 mm for JXUST-12 and JXUST-12a, respectively.
TGA was performed from room temperature to 900 °C (or 1000 °C) under a N2 atmosphere to explore the thermal stabilities of JXUST-12 and JXUST-12a. As displayed in Fig. S4a (ESI†), JXUST-12 remained stable before 285 °C, and the weight loss of 13.8% between 285 °C and 540 °C was due to the loss of the coordinated DMF molecules. Subsequently, the framework began to collapse after 540 °C, demonstrating that JXUST-12 displayed a relatively good thermal stability. Similarly, the mass loss of 13.6% for JXUST-12a was due to the removal of free H2O molecules in the channel and coordination of H2O molecules before reaching 540 °C (Fig. S4b, ESI†).
Fluorescence behaviors
Generally, MOFs constructed by rare earth ions and conjugated BTD-based organic ligands can display excellent luminescence properties.1,11,44 The emission spectra of H4BTDI, JXUST-12 and JXUST-12a were investigated in the solid state at room temperature. As shown in Fig. S6a (ESI†), the emission peaks of H4BTDI ligand, JXUST-12 and JXUST-12a occurred at 515, 510 and 520 nm upon excitation at 370 nm, respectively. The emission of H4BTDI ligand might be ascribed to the intraligand π* → π or π* → n transitions.45 In addition, JXUST-12 and JXUST-12a exhibited emission peaks at 499 and 504 nm in EtOH solution, respectively. The quantum efficiencies of JXUST-12 and JXUST-12a are 16.07% and 1.93%, respectively. The emission peaks at 505 nm in aqueous solution were observed for JXUST-12a (Fig. S6b, ESI†). The emission peaks of JXUST-12 and JXUST-12a in solvents were different from those in the solid state, which was influenced by the solvent effect.
Selective sensing of drug molecules
The luminescence performance of JXUST-12 and JXUST-12a prompted us to investigate the sensing properties toward drugs for antibiotics (NFT, NZF, FO, OFX, ornidazole (ODZ), metronidazole (MDZ), sulfamethazine (SMZ), sulfadiazine (SDZ), florfenicol (FFC), chloramphenicol (CAP) and 3-nitropropionic (3-NPA)) and non-antibiotics (DCN and 4-nitroimidazole (4-ND)). All fluorescence experiments were performed at room temperature. The stable suspension was prepared by immersing 0.5 mg finely ground sample in 2 mL dispersant and sonicating for 30 minutes.
The samples of JXUST-12a were dispersed in EtOH or aqueous solutions (2 mL) containing 5 μL different drug molecules (0.2 M) to conduct luminescence experiments. Different from JXUST-12a, the fluorescence experiment of JXUST-12 was carried out in EtOH solution. The results show that JXUST-12 has a turn-off effect on NFT, NZF and DCN, and the luminescence quenching efficiencies (Q) reached 99.53%, 99.62% and 99.45%, respectively (Fig. 3). Q is calculated using the following formula: Q = (I0 − I)/I0 × 100% (I0 and I are the maximum luminescence intensities before and after the addition of the target analyte).46 Therefore, JXUST-12 can be considered as a promising luminescent probe for NFT, NZF and DCN molecules. Furthermore, anti-interference experiments were performed to validate the high selectivity of JXUST-12 for the detection of NFT, NZF and DCN. By mixing NFT, NZF and DCN with various coexisting drugs, respectively (the ratio of NFT/NZF/DCN and coexisting drugs was 1
:
1), the luminescence turn-off effect was still retained (Fig. S12, ESI†), implying that JXUST-12 could selectively detect NFT, NZF and DCN in the presence of other drugs. To better investigate the sensitivity of JXUST-12 towards NFT, NZF and DCN, titration experiments with varying concentrations of NFT, NZF and DCN were also performed through gradually adding NFT, NZF and DCN (0.004 M) to the suspension of JXUST-12. The intensity of JXUST-12 gradually decreased upon the addition of NFT, NZF and DCN (Fig. 5). The fitted Stern–Volmer (SV) curves showed that there was a good linear relationship between luminescence intensities and the concentrations of NFT, NZF and DCN (Fig. S7, ESI†). According to the Stern–Volmer equation: I0/I = 1 + KSV[M],47 the KSV values of NFT, NZF and DCN are 4.36 × 104 M−1, 4.33 × 104 M−1 and 4.75 × 104 M−1, respectively ([M] is the concentration of NFT, NZF and DCN; I0 and I are the luminescence intensities of JXUST-12 before and after adding analytes; and KSV is the quenching coefficient (M−1)). The LODs calculated using the 3σ/k formula (σ: standard error and k: the slope)48 for NFT, NZF and DCN are 0.86, 0.82 and 0.88 μM, respectively (Fig. 5 and S7, ESI†). As shown in Table S6 (ESI†), NFT, NZF and DCN identified by JXUST-12 have higher KSV values and lower LODs, so JXUST-12 could be used as a potential candidate for selective detection of NFT, NZF and DCN with high selectively and sensitivity. Considering the cost of materials synthesis, the reusable performance plays an important role in luminescent probes and thus the cyclic experiment was performed. The Q of JXUST-12 was retained even after 5 cycles (Fig. S16a, ESI†), and could be reused by washing with EtOH several times. The PXRD patterns of the fifth recycled samples were consistent with the simulated one (Fig. S17a, ESI†), indicating that the framework still remained stable. These results reveal that JXUST-12 has high selectivity, sensitivity and recyclability toward NFT, NZF and DCN.
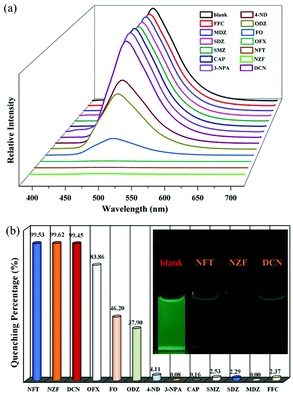 |
| Fig. 3 (a) The emission spectra of JXUST-12 dispersed in EtOH solution containing different drug molecules. (b) The quenching efficiencies of JXUST-12 dispersed in the EtOH solution containing different drug molecules (inset: the photographs of JXUST-12 dispersed in EtOH solutions containing NFT, NZF and DCN under a 365 nm UV-lamp). | |
Similarly, JXUST-12a also exhibited a turn-off effect for detecting NFT, NZF and DCN in EtOH solution (Fig. S8a, ESI†). A series of fluorescence experiments for sensing NFT, NZF and DCN were performed, which demonstrated that JXUST-12a had good anti-interference ability and recyclability (Fig. S13, S16b and S17b, ESI†). The Q value reached 99.34% (NFT), 99.21% (NZF) and 99.81% (DCN), respectively (Fig. S8b, ESI†). The Ksv values of NFT, NZF and DCN are 4.36 × 104 M−1, 4.33 × 104 M−1 and 4.75 × 104 M−1, respectively (Table S6, ESI†). The LODs of JXUST-12a sensing for NFT, NZF and DCN were calculated to be 0.44, 0.40 and 0.34 μM, respectively (Fig. S9, ESI†), which were lower than those of JXUST-12 and other reported examples (Table S6, ESI†).
Due to the excellent water stability, JXUST-12a was used to sense drug molecules in the aqueous solution. The results showed that JXUST-12a exhibited a fluorescence turn-off effect toward NFT and NZF, and a fluorescence turn-on effect and blue shift of emission peaks toward OFX and FO (Fig. 4a). The fluorescence effects of JXUST-12a and JXUST-12a containing OFX and FO were obvious in the CIE chromaticity diagram (Fig. S11, ESI†). The Q value reached 99.34% (NFT) and 99.32% (NZF), respectively (Fig. 4b). According to the fluorescence enhancement equation: I/I0 = 1 + Kec[M]49 (Kec is the enhancement coefficient (M−1)), the Ksv/Kec values of NFT, NZF, OFX and FO are 1.74 × 105 M−1, 1.43 × 105 M−1, 5.11 × 103 M−1 and 6.03 × 103 M−1, respectively (Table S6, ESI†). The LODs of JXUST-12a toward NFT, NZF, OFX and FO were 0.39, 0.43, 7.34 and 2.28 μM, respectively (Fig. 6 and S10, ESI†). NFT, NZF, OFX and FO identified by JXUST-12a had higher Ksv/Kec values, lower LODs and a better recognition effect than some reported examples (Table S6, ESI†). As shown in Fig. S14, S16c and S17c (ESI†), JXUST-12a had high anti-interference ability and recyclability toward NFT and NZF. Additionally, the time responses of JXUST-12a for detecting OFX and FO were determined and the luminescence intensities of JXUST-12a were continuously recorded with the addition of 5 μL of 0.2 M OFX and FO in aqueous solution. The emission peak shifted gradually and accompanied by increasing fluorescence intensity (Fig. S15, ESI†), which showed the highly effective sensing process for OFX and FO. The results showed that JXUST-12a could be considered as an excellent luminescent sensor for detecting NFT, NZF, OFX and FO in aqueous solutions.
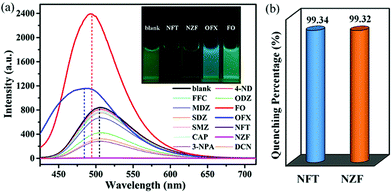 |
| Fig. 4 (a) Emission spectra of JXUST-12a dispersed in aqueous solutions containing different drug molecules. (b) Quenching efficiencies of NFT and NZF containing JXUST-12a dispersed in the aqueous solution. | |
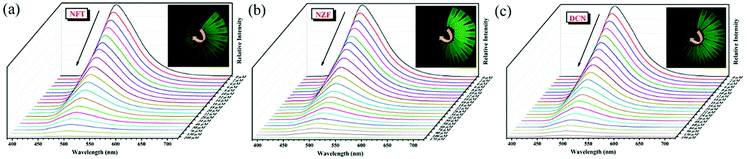 |
| Fig. 5 Emission spectra of JXUST-12 dispersed in EtOH solutions with various concentrations of NFT (a), NZF (b) and DCN (c). (Inset: photographs of JXUST-12 with NFT (a, 0–200 μM), NZF (b, 0–170 μM) and DCN (c, 0–196 μM) under a 365 nm UV-lamp.) | |
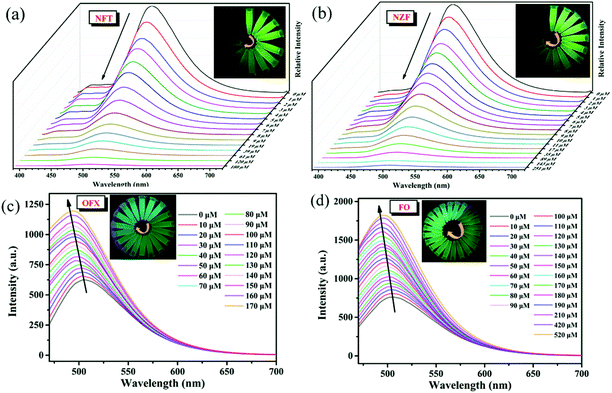 |
| Fig. 6 Emission spectra of JXUST-12a dispersed in aqueous solutions with various concentrations of NFT (a), NZF (b), OFX (c) and FO (d). (Inset: photographs of JXUST-12a with NFT (a, 0–180 μM), NZF (b, 0–251 μM), OFX (c, 0–170 μM) and FO (d, 0–520 μM) under a 365 nm UV-lamp.) | |
It is significant for luminescent materials to be used as devices for practical applications. For providing simple and handy detection, fluorescent test papers and composite films were further developed. The sample and filter paper were soaked in EtOH solutions and ultrasonicated for 30 minutes to form fluorescent test papers. The composite film materials were attained by adding poly(methyl methacrylate) (PMMA) and JXUST-12 or JXUST-12a in DMF, and then heating at 35 °C. Then fluorescent test papers and composite films were used to detect the analytes. As shown in Fig. 7 and 8, the fluorescence test papers and composite films of JXUST-12 and JXUST-12a had significant color changes observed with the naked eye under a 365 nm UV lamp before and after adding NFT, NZF, DCN, OFX or FO. The composite films of JXUST-12 and JXUST-12a have excellent flexibility (Fig. 8a and d). Therefore, the fluorescence test papers and composite films of JXUST-12 and JXUST-12a could be used as simple and handy luminescent probes.50
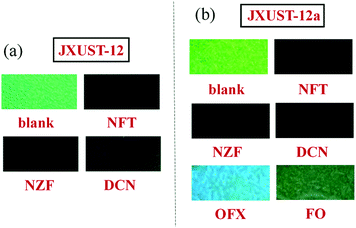 |
| Fig. 7 Optical images of JXUST-12 (a) and JXUST-12a (b) based fluorescent test papers with various drugs under 365 nm UV light. | |
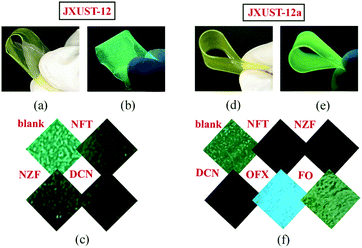 |
| Fig. 8 Optical images of JXUST-12 and JXUST-12a based composite films with various drug molecules in natural light (a and d) and under a 365 nm UV-lamp (b, c, e and f). | |
Detection mechanism
The related mechanisms of detecting analytes have been discussed. Generally, the luminescence change induced by small molecules may be attributed to the following mechanisms: (1) structure collapse,51 (2) competitive energy absorption,29 (3) absorbance caused enhancement (ACE),49 and (4) photoinduced electron transfer (PET).52,55 Herein, in order to confirm the mechanism of luminescence quenching of JXUST-12 and JXUST-12a toward NFT, NZF and DCN in EtOH solution, the stability of JXUST-12 and JXUST-12a before and after adding NFT, NZF and DCN molecules was respectively explored. The PXRD patterns of JXUST-12 and JXUST-12a dispersed in EtOH solutions of NFT, NZF and DCN were very well consistent with the simulated ones (Fig. S18a, ESI†), demonstrating that the mechanism of the collapse of the framework could be excluded. Then, the UV-vis absorption spectra of JXUST-12 and JXUST-12a upon the addition of NFT, NZF or DCN in EtOH solution were measured. The UV-vis absorption spectra of NFT, NZF and DCN in the range of 335 to 385 nm were basically overlapped with the fluorescence excitation spectra of JXUST-12 and JXUST-12a, while no obvious peaks were observed for other drug molecules in the same wavelength range (Fig. S19a, ESI†). The results demonstrated that the competitive absorption between NFT (or NZF and DCN) and the excitation of JXUST-12 and JXUST-12a led to fluorescence quenching.29,52 Besides, PET is a process of excited electron transfer from the photo-excited donor (luminophores) to the lowest unoccupied molecular orbital (LUMO) of the acceptor.53 The main driving force is the energy gap between the LUMO of the reactants.54 NFT, NZF and DCN with strong electron withdrawing groups (–NO2) and the LUMO might have caused excitation of electrons from the conduction band of the excited states of JXUST-12 and JXUST-12a.55 Therefore, the luminescence quenching of JXUST-12 and JXUST-12a toward NFT, NZF and DCN in the EtOH solution might be attributed to the PET mechanism. Because the luminescence of JXUST-12 mainly originated from H4BTDI ligand, the PET process between the H4BTDI ligand and NFT (or NZF, DCN) might quench the luminescence of JXUST-12. To further demonstrate the PET mechanism, the calculation based on density functional theory (DFT) was implemented. The LUMO energy level of H4BTDI (−3.35 eV) (Fig. 9) was higher than those of NFT (−3.86 eV) and NZF (−3.62 eV),33 which supported the PET process from H4BTDI to NFT and NZF. However, the LUMO energy level of H4BTDI (−3.35 eV) was lower than that of DCN (−2.4 eV),30 which was not supported by the PET process from H4BTDI to DCN. Therefore, the mechanism of the luminescence quenching for JXUST-12 and JXUST-12a on adding NFT and NZF in the EtOH solution might be due to the competitive energy absorption mechanism and the PET mechanism, while the quenching of JXUST-12 and JXUST-12a induced by DCN was due to the existence of competitive absorption.
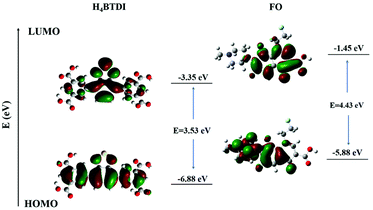 |
| Fig. 9 The calculated contour plots of the HOMO, LUMO and HOMO–LUMO energy band gaps for H4BTDI and FO. | |
The mechanisms of JXUST-12a sensing NFT, NZF, OFX and FO in the aqueous solution were also studied. Because JXUST-12a had the same quenching effect after adding NFT and NZF in the EtOH and aqueous solutions, it can be speculated that they could have the same quenching mechanism. Firstly, the luminescence quenching did not stem from the collapse of the framework because of the structural integrity of the recyclable sample confirmed by the PXRD patterns (Fig. S18c, ESI†). Secondly, the absorption spectra of the NFT and NZF in aqueous solutions were overlapped with the excitation spectra of JXUST-12a (Fig. S19b, ESI†). Thirdly, the PET process from H4BTDI to NFT and NZF is confirmed because the LUMO energy level of H4BTDI was higher than those of NFT and NZF. Similar to JXUST-12a in the EtOH solution, the fluorescence quenching of JXUST-12a in the aqueous solution toward NFT and NZF might be due to the competitive energy absorption and PET mechanism.
In addition, the mechanisms of fluorescence enhancement and blue shift of JXUST-12a on adding OFX and FO in the aqueous solution were explored. The PXRD patterns of JXUST-12a before and after adding OFX and FO matched well with the simulated one, indicating the retention of the framework (Fig. S18c, ESI†). The UV-vis absorption spectra of JXUST-12a on adding OFX and FO was measured. The results showed that the absorbance increased significantly from 250 to 400 nm after the addition of OFX and FO (Fig. S20, ESI†). At the same time, the absorbance gradually enhanced with the increase of the concentration of OFX and FO (Fig. S21, ESI†), indicating that the fluorescence turn-on effect and blue shift of JXUST-12a on adding OFX and FO in the aqueous solution might be the ACE mechanism.51 In addition, the LUMO energy levels of OFX and FO were −0.30 eV (ref. 33) and −1.45 eV, which are higher than the LUMO of H4BTDI (−3.35 eV) (Fig. 9). Therefore, the excited electrons of the LUMO of OFX and FO were transferred to the LUMO of the H4BTDI of JXUST-12a, which made JXUST-12a display fluorescence turn-on effect and blue shift.46,47,53,54 Therefore, the detection of OFX and FO by JXUST-12a in the aqueous solution might be attributed to the ACE and PET mechanisms.
Conclusions
One novel TbIII-based MOF (JXUST-12) have been successfully designed and synthesized by the incorporation of TbIII ions and H4BTDI ligands under solvothermal conditions. Interestingly, the temperature- and solvent-induced reversible SCSC conversion between JXUST-12 and JXUST-12a could be achieved and the transformed JXUST-12a exhibits excellent water stability and pH stabilities. JXUST-12 and JXUST-12a exhibit the fluorescence turn-off effect toward NFT, NZF and DCN in EtOH solution. Besides, JXUST-12a not only has the fluorescence turn-off effect toward NFT and NZF, but also has a fluorescence turn-on effect and blue shift toward OFX and FO in the aqueous solution. The LODs of JXUST-12a for the detection of NFT, NZF or DCN were lower than those of JXUST-12, indicating better fluorescence sensing properties. The fluorescence test papers and composite films were further developed and the practical application of fluorescence sensors was expanded. Further studies of MOF-based fluorescent sensors with SCSC transformation are under way in our group.
Conflicts of interest
There are no conflicts to declare.
Acknowledgements
This work was supported by the National Natural Science Foundation of China (22061019, 21861018 and 22161019), the Natural Science Foundation of Jiangxi Province of China (20202ACBL213001 and 20182BCB22010), Jiangxi Provincial Key Laboratory of Functional Molecular Materials Chemistry (20212BCD42018), the Youth Jinggang Scholars Program in Jiangxi Province (QNJG2019053), and the Two Thousand Talents Program in Jiangxi Province (jxsq2019201068). We also thank Xi-Ying Cao at South China Normal University for DFT calculations.
Notes and references
- A. Mallick, A. M. El-Zohry, O. Shekhah, J. Yin, J.-T. Jia, H. Aggarwal, A.-H. Emwas, O. F. Mohammed and M. Eddaoudi, Unprecedented ultralow detection limit of amines using a thiadiazole-functionalized Zr(IV)-based metal-organic framework, J. Am. Chem. Soc., 2019, 141, 7245–7249 CrossRef CAS PubMed.
- D.-X. Gu, W.-T. Yang, D.-Y. Lin, X.-D. Qin, Y.-H. Yang, F.-X. Wang, Q.-H. Pan and Z.-M. Su, Water-stable lanthanide-based metal-organic gel for the detection of organic amines and white-light emission, J. Mater. Chem. C, 2020, 8, 13648–13654 RSC.
- J.-K. Jin, K. Wu, X.-Y. Liu, G.-Q. Huang, Y.-L. Huang, D. Luo, M. Xie, Y.-F. Zhao, W.-G. Lu, X.-P. Zhou, J. He and D. Li, Building a pyrazole–benzothiadiazole–pyrazole photosensitizer into metal-organic frameworks for photocatalytic aerobic oxidation, J. Am. Chem. Soc., 2021, 143, 21340–21349 CrossRef CAS PubMed.
- S.-J. Liu, C. Cao, S.-L. Yao, T.-F. Zheng, Z.-X. Wang, C. Liu, J.-S. Liao, J.-L. Chen, Y.-W. Li and H.-R. Wen, Temperature- and vapor-induced reversible single-crystal-to-single-crystal transformations of three 2D/3D GdIII-organic frameworks exhibiting significant magnetocaloric effects, Dalton Trans., 2017, 46, 64–70 RSC.
- R. Li, S.-H. Wang, X.-X. Chen, J. Lu, Z.-H. Fu, Y. Li, G. Xu, F.-K. Zheng and G.-C. Guo, Highly anisotropic and water molecule-dependent proton conductivity in a 2D homochiral copper(II) metal–organic framework, Chem. Mater., 2017, 29, 2321–2331 CrossRef CAS.
- J. Wang, Y. Zhang, P.-X. Zhang, J.-B. Hu, R.-B. Lin, Q. Deng, Z.-L. Zeng, H.-B. Xing, S.-G. Deng and B.-L. Chen, Optimizing pore space for flexible-robust metal–organic framework to boost trace acetylene removal, J. Am. Chem. Soc., 2020, 142, 9744–9751 CAS.
- C. Wang, L.-J. Li, J. G. Bell, X.-X. Lv, S.-F. Tang, X.-B. Zhao and K. M. Thomas, Hysteretic gas and vapor sorption in flexible interpenetrated lanthanide-based metal-organic frameworks with coordinated molecular gating via reversible single-crystal-to-single-crystal transformation for enhanced selectivity, Chem. Mater., 2015, 27, 1502–1516 CrossRef CAS.
- Z.-J. Li, M. Lei, H.-L. Bao, Y. Ju, H.-J. Lu, Y.-X. Li, Z.-H. Zhang, X.-F. Guo, Y. Qian, M.-Y. He, J.-Q. Wang, W. Liu and J. Lin, A cationic thorium-organic framework with triple single-crystal-to-single-crystal transformation peculiarities for ultrasensitive anion recognition, Chem. Sci., 2021, 12, 15833–15842 RSC.
- A. Atilgan, T. Islamoglu, A. J. Howarth, J. T. Hupp and O. K. Farha, Detoxification of a sulfur mustard simulant using a BODIPY-functionalized zirconium-based metal-organic framework, ACS Appl. Mater. Interfaces, 2017, 9, 24555–24560 CrossRef CAS PubMed.
- Z.-Q. Yao, J. Xu, B. Zou, Z.-P. Hu, K. Wang, Y.-J. Yuan, Y.-P. Chen, R. Feng, J.-B. Xiong, J.-L. Hao and X.-H. Bu, A dual-stimuli-responsive coordination network featuring a reversible wide-range luminescence tuning behavior, Angew. Chem., Int. Ed., 2019, 58, 5614–5618 CrossRef CAS PubMed.
- W.-W. He, S.-L. Li and Y.-Q. Lan, Liquid-free single-crystal to single-crystal transformations in coordination polymers, Inorg. Chem. Front., 2018, 5, 279–300 RSC.
- Y.-Q. Lan, H.-L. Jiang, S.-L. Li and Q. Xu, Solvent-Induced controllable synthesis, single-crystal to single-crystal transformation and
encapsulation of Alq3 for modulated luminescence in (4,8)-connected metal-organic frameworks, Inorg. Chem., 2012, 51, 7484–7491 CrossRef CAS PubMed.
- X.-J. Hong, M.-F. Wang, H.-G. Jin, Q.-G. Zhan, Y.-T. Liu, H.-Y. Jia, X. Liu and Y.-P. Cai, Single-crystal to single-crystal transformation from a 1-D chain-like structure to a 2-D coordination polymer on heating, CrystEngComm, 2013, 15, 5606–5611 RSC.
- J. Yang, X.-Q. Wang, F.-N. Dai, L.-L. Zhang, R.-M. Wang and D.-F. Sun, Improving the porosity and catalytic capacity of a zinc paddlewheel metal-organic framework (MOF) through metal-ion metathesis in a single-crystal-to-single-crystal fashion, Inorg. Chem., 2014, 53, 10649–10653 CrossRef CAS PubMed.
- Y.-H. Yang, G.-J. Ren, W.-K. Yang, D.-Y. Lin, M.-L. Li, Z. Chang, Y. Fang, Z.-Q. Liang and Q.-H. Pan, Single-crystal to single-crystal transformation of metal-organic framework nanoparticles for encapsulation and pH-stimulated release of camptothecin, ACS Appl. Nano Mater., 2021, 4, 7191–7198 CrossRef CAS.
- S.-S. Bao, N.-Z. Li, J. M. Taylor, Y. Shen, H. Kitagawa and L.-M. Zheng, Co-Ca phosphonate showing humidity-sensitive single crystal to single crystal structural transformation and tunable proton conduction properties, Chem. Mater., 2015, 27, 8116–8125 CrossRef CAS.
- N. Li, J. Xu, R. Feng, T.-L. Hu and X.-H. Bu, Governing metal-organic frameworks towards high stability, Chem. Commun., 2016, 52, 8501–8513 RSC.
- F.-F. Li, L. Zhang, L.-L. Gong, C.-S. Yan, H.-Y. Gao and F. Luo, Reversible photo/thermoswitchable dual-color fluorescence through single-crystal-to-single-crystal transformation, Dalton Trans., 2017, 46, 338–341 RSC.
- J. Jin, J.-J. Xue, Y.-C. Liu, G.-P. Yang and Y.-Y. Wang, Recent progresses in luminescent metal-organic frameworks (LMOFs) as sensors for the detection of anions and cations in aqueous solution, Dalton Trans., 2021, 50, 1950–1972 RSC.
- L. Ding, Y. Zhao, H. Li, Q. Zhang, W. Yang, B. Fu and Q. Pan, A highly selective ratiometric fluorescent probe for doxycycline based on the fluorescence sensitization effect of bovine serum albumin, J. Hazard. Mater., 2021, 416, 125759 CrossRef CAS PubMed.
- M.-L. Li, G.-J. Ren, W.-T. Yang, Y.-H. Yang, W.-K. Yang, Y. Gao, P.-F. Qiu and Q.-H. Pan, Dual-emitting piezofluorochromic dye@MOF for white-light generation, Chem. Commun., 2021, 57, 1340–1343 RSC.
- J. Cheng, Y.-F. Li, J. Zhong, Z.-W. Lu, G.-T. Wang, M.-M. Sun, Y.-Y. Jiang, P. Zou, X.-X. Wang, Q.-B. Zhao, Y.-Y. Wang and H.-B. Rao, Molecularly imprinted electrochemical sensor based on biomass carbon decorated with MOF-derived Cr2O3 and silver nanoparticles for selective and sensitive detection of nitrofurazone, Chem. Eng. J., 2020, 398, 125664 CrossRef CAS.
- H.-W. Yang, P. Xu, B. Ding, Z.-Y. Liu, X.-J. Zhao and E.-C. Yang, A highly-stable luminescent Eu-MOF exhibiting efficient response to nitrofuran antibiotics through inner filter effect and photoinduced electron transfer, Eur. J. Inorg. Chem., 2019, 2019, 5077–5084 CrossRef CAS.
- A. S. Oberoi, Y.-Y. Jia, H.-Q. Zhang, S. K. Khanal and H. Lu, Insights into the fate and removal of antibiotics in engineered biological treatment systems: a critical review, Environ. Sci. Technol., 2019, 53, 7234–7264 CrossRef CAS PubMed.
- P.-L. Wang, L.-H. Xie, E. A. Joseph, J.-R. Li, X.-O. Su and H.-C. Zhou, Metal-organic frameworks for food safety, Chem. Rev., 2019, 119, 10638–10690 CrossRef CAS PubMed.
- Y.-F. Xie, X.-Y. Zhu, Y.-Y. Sun, H.-Y. Wang, H. Qian and W.-R. Yao, Rapid detection method for nitrofuran antibiotic residues by surface-enhanced raman spectroscopy, Eur. Food Res. Technol., 2012, 235, 555–561 CrossRef CAS.
- G. Chakraborty, P. Das and S. K. Mandal, Strategic construction of highly stable metal-organic frameworks combining both semi-rigid tetrapodal and rigid ditopic linkers: selective and ultrafast sensing of 4-nitroaniline in water, ACS Appl. Mater. Interfaces, 2018, 10, 42406–42416 CrossRef CAS PubMed.
- B. Wang, X.-L. Lv, D.-W. Feng, L.-H. Xie, J. Zhang, M. Li, Y.-B. Xie, J.-R. Li and H.-C. Zhou, Highly stable Zr(IV)-based metal-organic frameworks for the detection and removal of antibiotics and organic explosives in water, J. Am. Chem. Soc., 2016, 138, 6204–6216 CrossRef CAS PubMed.
- N. Seal, M. Singh, S. Das, R. Goswami, B. Pathak and S. Neogi, Dual-functionalization actuated trimodal attribute in ultra-robust MOF: exceptionally selective capture and effectual fixation of CO2 with fast-responsive, nanomolar detection of assorted organo-contaminants in water, Mater. Chem. Front., 2021, 5, 979–994 RSC.
- C.-L. Tao, B. Chen, X.-G. Liu, L.-J. Zhou, X.-L. Zhu, J. Cao, Z.-G. Gu, Z.-J. Zhao, L. Shen and B.-Z. Tang, A highly luminescent entangled metal-organic framework based on pyridine-substituted tetraphenylethene for efficient pesticide detection, Chem. Commun., 2017, 53, 9975–9978 RSC.
- M. Hollweg, H.-P. Kapfhammer, M. Krupinski and H.-J. Möller Nervenarzt, Psychopathol ogische syndrome unter behandlung mit gyrasehemmern, Nervenarzt, 1997, 68, 38–47 CrossRef CAS PubMed.
- J.-N. Xiao, M.-Y. Liu, F.-L. Tian and Z.-L. Liu, Stable Europium-based metal-organic frameworks for naked-eye ultrasensitive detecting fluoroquinolones antibiotics, Inorg. Chem., 2021, 60, 5282–5289 CrossRef CAS PubMed.
- C.-P. Li, W.-W. Long, Z. Lei, L. Guo, M.-J. Xie, J. Lü and X.-D. Zhu, Anionic metal-organic framework as a unique turn-on fluorescent chemical sensor for ultra-sensitive detection of antibiotics, Chem. Commun., 2020, 56, 12403–12406 RSC.
- R. A. Pearson, C. Evans and J. G. Bendall, Nitrofurazone quantification in milk at the european union minimum required performance limit of 1 ng g−1: circumventing the semicarbazide problem, Food Addit. Contam., Part A, 2016, 33, 1324–1336 CAS.
- M. Tubino, M. M. D. C. Vila and M. N. Palumbo, Determination of nitrofurazone in topical pharmaceutical preparations: comparison of the UV-visible diffuse reflectance versus transmittance versus HPLC methods, J. Braz. Chem. Soc., 2009, 20, 1901–1907 CrossRef CAS.
- S.-L. Yao, S.-J. Liu, X.-M. Tian, T.-F. Zheng, C. Cao, C.-Y. Niu, Y.-Q. Chen, J.-L. Chen, H.-P. Huang and H.-R. Wen, A ZnII-based metal-organic framework with a rare tcj topology as a turn-on fluorescent sensor for acetylacetone, Inorg. Chem., 2019, 58, 3578–3581 CrossRef CAS PubMed.
- J. Li, S.-L. Yao, S.-J. Liu and Y.-Q. Chen, Fluorescent sensors for aldehydes based on luminescent metal-organic frameworks, Dalton Trans., 2021, 50, 7166–7175 RSC.
- Z. Lei, L. Hu, Z.-H. Yu, Q.-Y. Yao, X. Chen, H. Li, R.-M. Liu, C.-P. Li and X.-D. Zhu, Ancillary ligand enabled structural and fluorescence diversity in metal-organic frameworks: application for the ultra-sensitive detection of nitrofuran antibiotics, Inorg. Chem. Front., 2021, 8, 1290–1296 RSC.
- Q. Cheng, X. Han, Y. Tong, C. Huang, J. Ding and H.-W. Hou, Two 3D Cd(II) metal–organic frameworks linked by benzothiadiazole dicarboxylates: fantastic S@Cd6 cage, benzothiadiazole antidimmer, and dual emission, Inorg. Chem., 2017, 56, 1696–1705 CrossRef CAS PubMed.
- W.-Q. Zhang, Q.-Y. Li, J.-Y. Cheng, K. Cheng, X.-Y. Yang, Y.-W. Li, X.-S. Zhao and X.-J. Wang, Ratiometric luminescent detection of organic amines due to the induced lactam–lactim tautomerization of organic linker in a metal-organic framework, ACS Appl. Mater. Interfaces, 2017, 9, 31352–31356 CrossRef CAS PubMed.
- A. M. El-Zohry, A. Alturki, J. Yin, A. Mallick, O. Shekhah, M. Eddaoudi, B. S. Ooi and O. F. Mohammed, Tunable twisting motion of organic linkers via concentration and hydrogen-bond formation, J. Phys. Chem. C, 2019, 123, 5900–5906 CrossRef CAS.
- X.-M. Tian, S.-L. Yao, C.-Q. Qiu, T.-F. Zheng, Y.-Q. Chen, H.-P. Huang, J.-L. Chen, S.-J. Liu and H.-R. Wen, Turn-on luminescent sensor toward Fe3+, Cr3+, and Al3+ based on a Co(II) metal-organic framework with open functional sites, Inorg. Chem., 2020, 59, 2803–2810 CrossRef CAS PubMed.
-
M. Llunell, D. Casanova, J. Cirera, P. Alemany and S. Alvarez, SHAPE, version 2.1, Universitat de Barcelona, Barcelona, Spain, 2013 Search PubMed.
- D. Zhao, D. Yue, K. Jiang, Y.-J. Cui, Q. Zhang, Y. Yang and G.-D. Qian, Ratiometric dual-emitting MOF⊃dye thermometers with a tunable operating range and sensitivity, J. Mater. Chem. C, 2017, 5, 1607–1613 RSC.
- C.-L. Song, Y.-B. He, B. Li, Y.-J. Ling, H.-L. Wang, Y.-L. Feng, R. Krishnac and B.-L. Chen, Enhanced CO2 sorption and selectivity by functionalization of a NbO-type metal-organic framework with polarized benzothiadiazole moieties, Chem. Commun., 2014, 50, 12105–12108 RSC.
- S. Pramanik, C. Zheng, X. Zhang, T. J. Emge and J. Li, New microporous metal-organic framework demonstrating unique selectivity for detection of high explosives and aromatic compounds, J. Am. Chem. Soc., 2011, 133, 4153–4155 CrossRef CAS PubMed.
- Z.-Q. Liu, Y. Zhao, X.-D. Zhang, Y.-S. Kang, Q.-Y. Lu, M. Azam, S. I. Al-Resayes and W.-Y. Sun, Metal-organic frameworks with 1,4-di(1H-imidazol-4-yl)benzene and varied carboxylate ligands for selectively sensing Fe(III) ion and ketone molecules, Dalton Trans., 2017, 46, 13943–13951 RSC.
- Y.-F. Zhao, M.-Y. Wan, J.-P. Bai, H. Zeng, W.-G. Lu and D. Li, pH-Modulated luminescence switching in a Eu-MOF: rapid detection of acidic amino acids, J. Mater. Chem. A, 2019, 7, 11127–11133 RSC.
- M. Wang, L. Guo and D.-P. Cao, Metal-organic framework as luminescence turn-on sensor for selective detection of metal ions: absorbance caused enhancement mechanism, Sens. Actuators, B, 2018, 256, 839–845 CrossRef CAS.
- W. Chen, R.-Q. Fan, J.-Z. Fan, H.-Y. Liu, T.-C. Sun, P. Wang and Y.-L. Yang, Lanthanide coordination polymer-based composite films for selective and highly sensitive detection of Cr2O72− in aqueous media, Inorg. Chem., 2019, 58, 15118–15125 CrossRef CAS PubMed.
- S.-Y. Wu, M.-C. Zhu, Y. Zhang, M. Kosinova, V. P. Fedin and E. Gao, A water stable lanthanide coordination polymer as multicenter platform for ratiometric luminescent sensing antibiotics, Chem. – Eur. J., 2020, 26, 3137–3144 CrossRef CAS PubMed.
- X.-H. Wang, M.-Y. Lei, T.-J. Zhang, Q.-F. Zhang, R.-F. Zhang and M. Yang, A water-stable multi-responsive luminescent Zn-MOF sensor for detecting of TNP, NZF and Cr2O72− in aqueous media, Dalton Trans., 2021, 50, 3816–3824 RSC.
- S. S. Nagarkar, B. Joarder, A. K. Chaudhari, S. Mukherjee and S. K. Ghosh, Highly selective detection of nitro explosives by a luminescent metal-organic framework, Angew. Chem., Int. Ed., 2013, 52, 2881–2885 CrossRef CAS PubMed.
- S.-Y. Wu, Y.-N. Lin, J.-W. Liu, W. Shi, G.-M. Yang and P. Cheng, Rapid detection of the biomarkers for carcinoid tumors by a water stable luminescent lanthanide metal-organic framework sensor, Adv. Funct. Mater., 2018, 28, 1707169 CrossRef.
- W. P. Lustig, S. Mukherjee, N. D. Rudd, A. V. Desai, J. Li and S. K. Ghosh, Metal-organic frameworks: functional luminescent and photonic materials for sensing applications, Chem. Soc. Rev., 2017, 46, 3242–3285 RSC.
Footnotes |
† Electronic supplementary information (ESI) available: X-ray crystallographic data, selected bond lengths and angles, SHAPE analysis of the TbIII ions, ESI table, structural figures, part of the experimental details, IR, PXRD, TGA curves, luminescence spectra and UV-vis absorption spectra. CCDC 2126107 (JXUST-12) and 2126108 (JXUST-12a). For ESI and crystallographic data in CIF or other electronic format see DOI: 10.1039/d2qi00023g |
‡ These authors contributed equally to this work and should be considered co-first authors. |
|
This journal is © the Partner Organisations 2022 |