DOI:
10.1039/D1QI01555A
(Research Article)
Inorg. Chem. Front., 2022,
9, 1812-1818
A highly stable Zn9-pyrazolate metal–organic framework with metallosalen ligands as a carbon dioxide cycloaddition catalyst†
Received
14th December 2021
, Accepted 13th January 2022
First published on 15th January 2022
Abstract
A three-dimensional (3D) metal–organic framework constructed from unprecedented Zn9O2(OH)2(pyz)12 (pyz = pyrazolate) clusters and Ni(salen)-derived linkers was reported. The MOF exhibits high catalytic activity for CO2 cycloaddition reactions with excellent stability. The MOF catalyst can be recycled at least 15 times without loss of activity and the turnover number (TON) per Ni site can reach as high as 2887.
As emerging materials, porous metal–organic frameworks (MOFs) have attracted much attention for applications in gas storage and separation,1 chemical sensing,2,3 catalysis,4 and many other fields over the past two decades.5 MOFs are constructed from organic linkers, usually polycarboxylates6,7 or polyazolates,8,9 and metal/metal cluster nodes, and thus are of rich structural and functional diversity that allows their fine-tuning and modification to meet different requirements of the mentioned applications. However, challenges remain. Chemical stability is one of the major concerns for further applications of MOFs.10–12 Several strategies have been developed to design more stable MOFs: (i) increasing the metal–ligand interaction strength with high pKa ligands (e.g. azoles) and/or high-valent metal ions (e.g. Zr4+,Cr3+);13,14 (ii) reducing the contact between water or aqueous attacking reagents and the coordination bonds of host frameworks;15 (iii) increasing the rigidity of ligands to enhance the stability.16 MOFs based on azolate donors usually show better stability than those of carboxylate donors because the sp2 N atoms tend to adopt saturated coordination and thus become locally hydrophobic and unavailable for interaction with attacking reagents.17,18 Meanwhile, interpenetrated motifs also significantly enhance the framework stability.19 It is not only because interpenetrated networks could reduce the pore size and increase the wall thickness, but also due to the higher strength of non-covalent interactions in interpenetrated structures which lock the linkers in place and prevent their displacement.15
Metallosalen complexes are one of the earliest developed and most widely explored categories of asymmetric transition metal catalysts.20–22 Since the early 1990s, metallosalen complexes have found catalytic applications for olefin epoxidation,23 aldehyde cyanation,24 epoxide ring-opening25 and CO2 cycloaddition reactions.26,27 Heterogenized metallosalen catalysts have been constructed as surface-grafted silica or resins,28 MOFs,29–31 covalent organic frameworks (COFs),32,33 and porous organic polymers (POPs)34 for better catalyst separation and recyclability. However, it is still challenging to balance the catalytic activity/selectivity with material stability. MOF catalysts based on metallosalen-derived polycarboxylate linkers have shown exceptional catalytic performance compared to other heterogeneous metallosalen catalysts,35–38 but the stability of those materials is not yet satisfactory.17
Herein, we report the synthesis and catalytic applications of a zinc-pyrazolate MOF constructed from Ni(salen) bispyrazolate ligands and unprecedented Zn9 clusters. The zinc-pyrazolate connection allows the MOF to withstand acid and base treatments as well as boiling water. The MOF also exhibits high catalytic activity and exceptional recyclability for CO2-epoxide cycloaddition reactions.
The Zn9-pyrazolate MOF Zn9O2(OH)2(L)6 was obtained by a solvothermal reaction between Zn(OAc)2·6H2O and the Ni(salen)-derived ligand H2L (Fig. 1b and Scheme S1†) in a DMF/H2O/trifluoroacetic acid mixture solution. As shown in the scanning electron microscopy (SEM) image, the crystalline MOF particles are of oblique dodecahedron shape with a uniform size of 20 μm (Fig. 1d). The exact formula and structure of the MOF were determined by single-crystal X-ray diffraction (SCXRD) studies. Preliminary reflection data reduction indicates that the MOF crystallizes in the cubic Fd
m space group. Further structure refinement revealed that the L2− linker exhibits symmetry-related, two-fold disorder. To better describe the structure, the apparent symmetry of the framework was arbitrarily lowered to the chiral F4132 space group with a racemic twinning parameter accounting for the disorder. The MOF has a formula of Zn9O2(OH)2(L)6 and contains an unprecedented [Zn9O2(OH)2(pyz)12] cluster secondary building unit (SBU) (Fig. 1a). In the SBU, eight Zn atoms are located at the eight vertexes of a cuboid with an additional Zn atom at the center. The central Zn(II) atom adopts a distorted tetrahedral ZnO4 coordination sphere, while the four O atoms connect to eight vertical Zn(II) atoms in a μ3-O fashion (Fig. 1a). The twelve pyrazolate groups coordinate to the Zn9 cuboid cluster from the directions of twelve edges; therefore, each vertical Zn(II) ion is tetracoordinated in a ZnN3O fashion. The Zn–Zn distances between vertical and central Zn(II) ions are 3.06 Å and 3.14 Å, respectively, and the distances between vertical Zn(II) ions are 3.58 Å, showing no intermetallic bonding. Previous studies on zinc-pyrazolate coordination chemistry have found [Zn4O(pyz)6] and [Zn3O(pyz)3] clusters, and ZnN4 tetrahedron SBUs;18,39–41 yet to the best of our knowledge, the [Zn9O2(OH)2(pyz)12] cluster has never been reported. The Zn9O2(OH)2 cluster SBU is connected to twelve adjacent SBUs through Ni(salen)-based bis(pyrazolate) linkers to form a 3D network with face-centered cubic unit (fcu) topology, the same as that constructed from Ni8 clusters and linear bis(pyrazolate) linkers.42 The MOF adopts a two-fold interpenetration structure, which is unprecedented in previous Ni8(pyrazolate) MOFs.42–45 The Zn9 cluster of one network is located in the tetrahedral cavity formed by another network with 50% occupancy (Fig.S4†). The two interpenetrating networks are symmetrically related by the 41 screw axis and pseudo d glide plane. Because of the interpenetration, the pore size of the Zn9O2(OH)2(L)6MOF is only 10 Å as measured from the single crystal structure, a relatively small value compared to the ligand length. The powder X-ray diffraction (PXRD) pattern of the bulk MOF sample matches well with that from single-crystal structure simulation, confirming the phase purity (Fig. 1e). X-ray photoelectron spectroscopy (XPS) shows that both Zn and Ni are in the +2 oxidation state (Fig.S5†).
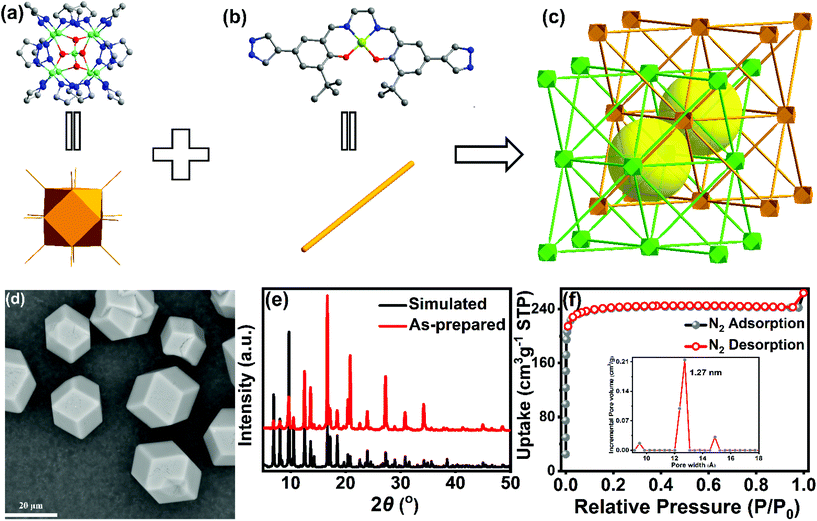 |
| Fig. 1 Construction of the Zn9O2(OH)2(L)6 MOF (Zn: turquoise; Ni: lime; N: blue; O: red.): (a) the Zn9O2(OH)2(pyrazolate)12 node; (b) the Ni(salen)-derived bis(pyrazolate) ligand; (c) two-fold interpenetration structure of the Zn9O2(OH)2(L)6 MOF; (d) SEM image of Zn9O2(OH)2(L)6; (e) simulated and experimental PXRD patterns of Zn9O2(OH)2(L)6; (f) N2 sorption isotherms of the Zn9O2(OH)2(L)6 MOF (inset: pore size distribution by the NLDFT method). | |
The N2 sorption isotherms (Fig. 1f) of Zn9O2(OH)2(L)6 revealed a typical type I behavior with a Brunauer–Emmett–Teller (BET) surface area of 934 m2g−1. Non-local density functional theory (NLDFT) pore size distribution analysis gave a total pore volume of 0.215 cm3g−1 with a pore size of 12.7 Å (Fig. 1f), which is in good agreement with the two-fold interpenetration structure. The CO2 sorption isotherm (Fig. S7a†) gave a maximum CO2 uptake of 168 cm3g−1. The Zn9O2(OH)2(L)6 MOF retained its crystallinity even after sorption tests as shown by the unchanged diffraction peaks (Fig.S7b†).
To explore the chemical stability of Zn9O2(OH)2(L)6, the MOF samples were treated with HCl, NaOH and ammonia aqueous solutions at room temperature, and H2O and HCl upon heating. In pH 2 HCl, 2.5% ammonia, and 2 M NaOH solutions, the MOF samples were stable for at least 210 days at room temperature as indicated by the PXRD patterns (Fig. 2). In pH 3 HCl and H2O, the MOF samples were stable for at least 21 days at 100 °C (Fig. 2 and S8†). Therefore, Zn9O2(OH)2(L)6 exhibits better chemical stability compared with most pyrazolate-based MOFs (Table S3†).46,47 Such good stability could be attributed to a combination of several effects. First, the Zn-pyrazole saturated coordination protects the metal centre from being attacked by a base.10,48 Second, the two-fold interpenetrated structure reduces the MOF porosity and inhibits guest diffusion through MOF channels, making the MOF more stable.15,49 Third, the pyrazolate ligand with hydrophobic t-butyl group modification results in a hydrophobic MOF with a water contact angle of 114° (Fig. S9†), further reducing the contact of the MOF with aqueous attacking reagents.42 All these effects taken together lead to the high chemical stability of Zn9O2(OH)2(L)6.
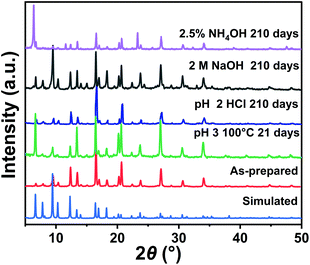 |
| Fig. 2 PXRD patterns of the as-prepared Zn9O2(OH)2(L)6 and those after acid, base and heating treatments. | |
Great interest has been shown in converting CO2 into value-added chemicals and reducing the greenhouse gas concentration in the atmosphere. The cycloaddition of CO2 with epoxides (Scheme 1) has been widely proposed as an effective approach for that purpose due to its near-100% atomic efficiency.50,51 Different types of catalysts, including organocatalysts,52 ionic liquids,53 metallosalen complexes,26 single atom catalysts54 and other coordination compounds,55,56 have been reported as effective catalysts for CO2 cycloaddition reactions. POPs34 and MOFs38,57–60 have been reported as the heterogeneous version of those molecular catalysts to facilitate the separation and recycling processes. Given the high stability of the Ni(salen)-derived Zn9O2(OH)2(L)6 MOF, we expected that it could be a good candidate as a heterogeneous CO2 cycloaddition catalyst. We first investigated its catalytic activity with styrene oxide as a model substrate. The reaction was almost complete in 24 hours at 80 °C in the presence of 1.5% MOF catalyst and 1.5% tetrabutylammonium bromide (TBAB) co-catalyst and under 7 bar CO2 atmosphere. When the MOF and TBAB loading was decreased from 1.5% to 0.5%, the yield of the carbonate product only decreased from 99% to 95% (entries 1 and 2, Table 1), indicating the high catalytic activity of the MOF/TBAB catalyst. When either the MOF or TBAB was absent, the product yield was much lower under the same pressure and temperature conditions (Fig. 3a and entries 3 and 4, Table 1), showing that both the components are necessary for the catalysis. Using the Ni(salen)-derived ligand H2L instead of the MOF gave a similar yield for styrene carbonate, showing that the ligand is the catalytically active component (entry 5, Table 1). Reducing the reaction temperature or pressure led to lower yields, yet such an effect can be compensated for by increasing the catalyst loading to 1% (entries 6–8, Table 1).
 |
| Scheme 1 CO2 cycloaddition reaction catalyzed by the MOF and TBAB. | |
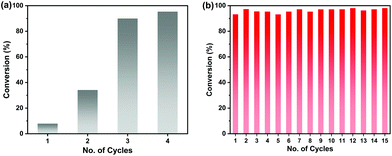 |
| Fig. 3 (a) Styrene oxide conversions with different catalysts; (b) styrene oxide conversions at different runs in the reuse experiments for CO2 cycloaddition. Reaction conditions: 80 °C, 7 atm CO2, 0.5% MOF catalyst and 0.5% TBAB co-catalyst. | |
Table 1 Optimization of reaction conditions for the cycloaddition of CO2 with styrene oxide
Entry |
Catalyst (%) |
TBAB (%) |
P (atm) |
T (°C) |
Conversion (%) |
The ligand H2L was used instead of the MOF.
|
1 |
1.5 |
1.5 |
7 |
80 |
99 |
2 |
0.5 |
0.5 |
7 |
80 |
95 |
3 |
0.5 |
0 |
7 |
80 |
8 |
4 |
0 |
0.5 |
7 |
80 |
34 |
5a |
0.5 |
0.5 |
7 |
80 |
90 |
6 |
0.5 |
0.5 |
4 |
80 |
68 |
7 |
0.5 |
0.5 |
7 |
60 |
67 |
8 |
1 |
1 |
4 |
80 |
95 |
The high chemical stability of the Zn9O2(OH)2(L)6 MOF allows easy recycling of the catalysts. After the reaction, the MOF catalyst was simply filtered off and washed to remove product residues in the pores, and then it was added to another batch of reactants. In this way, the MOF catalyst can be recycled at least 15 times without any loss in its catalytic activity (Fig. 3b). A total turnover number (TON) of more than 2887 was achieved after 15 cycles. SEM, PXRD and N2 sorption experiments showed that the MOF maintains the original morphology, structure and porosity after catalysis (Fig.S11†).
The catalytic activity of the Zn9O2(OH)2(L)6 MOF for substrates with different sizes was also investigated. With the increasing molecular sizes of the substrates, the isolated yields for the corresponding carbonate products showed a descending trend of 89%, 30%, and 9% for epichlorohydrin, styrene oxide, and stilbene oxide, respectively (Table S4†). The size-dependent conversion, therefore, demonstrates that the catalysis takes place in the MOF channels and not only on the external surface.
We next explored the catalytic activity of the MOF/TBAB system with different epoxide substrates. As shown in Table 2, the MOF/TBAB catalyst showed good conversion for epichlorohydrin (99%), phenyl glycidyl ether (98%) and cyclopentene oxide (82%) substrates, and moderate conversion for cyclohexene oxide (24%). It is worth noting that cyclohexene oxide is one of the very inactive substrates owing to its steric hindrance originating from the bicyclic structure.61 However, the yield of cyclohexene carbonate can be optimized to 82% by increasing the catalyst loading to 1.5%. This yield outperformed many previously reported heterogeneous catalysts under similar loading, temperature, and pressure conditions (Table S5†). The results fully demonstrate that the MOF has excellent catalytic activity and provides a convincing experimental basis for industrial applications.
Table 2 Cycloaddition reactions of CO2 with different epoxide substrates
Entry |
Substrate |
Catalyst (%) |
TBAB (%) |
Conversion (%) |
Yield (%) |
1 |
|
0.5 |
0.5 |
99 |
96 |
2 |
|
0.5 |
0.5 |
98 |
97 |
3 |
|
0.5 |
0.5 |
82 |
78 |
4 |
|
0.5 |
0.5 |
24 |
19 |
5 |
1.5 |
1.5 |
82 |
79 |
In summary, we reported the synthesis of a Zn-pyrazolate MOF with an unprecedented Zn9O2(OH)2(pyz)12 cluster and a two-fold interpenetration network structure. This MOF exhibits excellent chemical and stability in pH 2–14 aqueous solutions as well as in boiling water or weak acids. The MOF efficiently catalyzes CO2 cycloaddition reactions with various epoxide substrates, showing its high activity and exceptional recyclability. Consequently, this work will offer significant guidance in the design and synthesis of highly stable metal–pyrazolate MOFs, thereby promoting the discovery of their structures as well as potential applications.
Conflicts of interest
The authors declare no conflict of interest.
Acknowledgements
This work is financially supported by the National Key Research and Development Program of China (2017YFA0700103) and the National Natural Science Foundation of China (Grant No. 22005306). The authors greatly thank Prof. Li-Xin Wu (FJIRSM, CAS) for the help with contact angle measurements, Prof. Xin-Qiang Fang (FJIRSM, CAS) for the help with ee determination, and Bai-Tong Liu (FJIRSM, CAS), Jia-Wei Chen (XMU) and Shi-Rui Zhang (SNNU) for the help with crystallography and N2 sorption analysis.
Notes and references
- P. Q. Liao, N. Y. Huang, W. X. Zhang, J. P. Zhang and X. M. Chen, Controlling guest conformation for efficient purification of butadiene, Science, 2017, 356, 1193–1196 CrossRef CAS PubMed.
- Y. Cui, B. Li, H. He, W. Zhou, B. Chen and G. Qian, Metal-Organic Frameworks as Platforms for Functional Materials, Acc. Chem. Res., 2016, 49, 483–493 CrossRef CAS PubMed.
- M. L. Hu, S. A. A. Razavi, M. Piroozzadeh and A. Morsali, Sensing organic analytes by metal-organic frameworks: a new way of considering the topic, Inorg. Chem. Front., 2020, 7, 1598–1632 RSC.
- Y. B. Huang, J. Liang, X. S. Wang and R. Cao, Multifunctional metal-organic framework catalysts: synergistic catalysis and tandem reactions, Chem. Soc. Rev., 2017, 46, 126–157 RSC.
- H. Furukawa, K. E. Cordova, M. O'Keeffe and O. M. Yaghi, The chemistry and applications of metal-organic frameworks, Science, 2013, 341, 1230444 CrossRef PubMed.
- Y. Bai, Y. Dou, L. H. Xie, W. Rutledge, J. R. Li and H. C. Zhou, Zr-based metal-organic frameworks: design, synthesis, structure, and applications, Chem. Soc. Rev., 2016, 45, 2327–2367 RSC.
- W. Gong, Y. Liu, H. Y. Li and Y. Cui, Metal-organic frameworks as solid Brønsted acid catalysts for advanced organic transformations, Coord. Chem. Rev., 2020, 420, 213400 CrossRef CAS.
- C. Pettinari, A. Tabacaru and S. Galli, Coordination polymers and metal-organic frameworks based on poly(pyrazole)-containing ligands, Coord. Chem. Rev., 2016, 307, 1–31 CrossRef CAS.
- J. P. Zhang, H. L. Zhou, D. D. Zhou, P. Q. Liao and X. M. Chen, Controlling flexibility of metal-organic frameworks, Natl. Sci. Rev., 2018, 5, 907–919 CrossRef CAS.
- N. Li, J. Xu, R. Feng, T. L. Hu and X. H. Bu, Governing metal-organic frameworks towards high stability, Chem. Commun., 2016, 52, 8501–8513 RSC.
- H. He, Q. Sun, W. Gao, J. A. Perman, F. Sun, G. Zhu, B. Aguila, K. Forrest, B. Space and S. Ma, A Stable Metal-Organic Framework Featuring a Local Buffer Environment for Carbon Dioxide Fixation, Angew. Chem., Int. Ed., 2018, 57, 4657–4662 CrossRef CAS PubMed.
- C. Healy, K. M. Patil, B. H. Wilson, L. Hermanspahn, N. C. Harvey-Reid, B. I. Howard, C. Kleinjan, J. Kolien, F. Payet, S. G. Telfer, P. E. Kruger and T. D. Bennett, The thermal stability of metal-organic frameworks, Coord. Chem. Rev., 2020, 419, 213388 CrossRef CAS.
- K. S. Park, Z. Ni, A. P. Côté, J. Y. Choi, R. Huang, F. J. Uribe-Romo, H. K. Chae, M. O'Keeffe and O. M. Yaghi, Exceptional chemical and thermal stability of zeolitic imidazolate frameworks, Proc. Natl. Acad. Sci. U. S. A., 2006, 103, 10186–10191 CrossRef CAS PubMed.
- G. Huang, L. Yang, Q. Yin, Z. B. Fang, X. J. Hu, A. A. Zhang, J. Jiang, T. F. Liu and R. Cao, A Comparison of Two Isoreticular Metal-Organic Frameworks with Cationic and Neutral Skeletons: Stability, Mechanism, and Catalytic Activity, Angew. Chem., Int. Ed., 2020, 59, 4385–4390 CrossRef CAS PubMed.
- M. Ding, X. Cai and H. L. Jiang, Improving MOF stability: approaches and applications, Chem. Sci., 2019, 10, 10209–10230 RSC.
- X. L. Lv, S. Yuan, L. H. Xie, H. F. Darke, Y. Chen, T. He, C. Dong, B. Wang, Y. Z. Zhang, J. R. Li and H. C. Zhou, Ligand Rigidification for Enhancing the Stability of Metal-Organic Frameworks, J. Am. Chem. Soc., 2019, 141, 10283–10293 CrossRef CAS PubMed.
- A. V. Desai, S. Sharma, S. Let and S. K. Ghosh, N-donor linker based metal-organic frameworks (MOFs): Advancement and prospects as functional materials, Coord. Chem. Rev., 2019, 395, 146–192 CrossRef CAS.
- A. A. Zhang, X. Cheng, X. He, W. Liu, S. Deng, R. Cao and T. F. Liu, Harnessing Electrostatic Interactions for Enhanced Conductivity in Metal-Organic Frameworks, Research, 2021, 2021, 9874273 CAS.
- H. L. Jiang, T. A. Makal and H. C. Zhou, Interpenetration control in metal-organic frameworks for functional applications, Coord. Chem. Rev., 2013, 257, 2232–2249 CrossRef CAS.
- E. N. Jacobsen, W. Zhang, A. R. Muci, J. R. Ecker and L. Deng, Highly Enantioselective Epoxidation Catalysts Derived from 1,2-Diaminocyclohexane, J. Am. Chem. Soc., 1991, 113, 7063–7064 CrossRef CAS.
- E. J. Allain, L. P. Hager, L. Deng and E. N. Jacobsen, Highly Enantioselective Epoxidation of Disubstituted Alkenes with Hydrogen-Peroxide Catalyzed by Chloroperoxidase, J. Am. Chem. Soc., 1993, 115, 4415–4416 CrossRef CAS.
- M. Palucki, P. J. Pospisil, W. Zhang and E. N. Jacobsen, Highly Enantioselective, Low-Temperature Epoxidation of Styrene, J. Am. Chem. Soc., 2002, 116, 9333–9334 CrossRef.
- H. Yoon and C. J. Burrows, Catalysis of Alkene Oxidation by Nickel Salen Complexes Using Naocl under Phase-Transfer Conditions, J. Am. Chem. Soc., 1988, 110, 4087–4089 CrossRef CAS.
- S. Liang and X. R. Bu, Tertiary pentyl groups enhance salen titanium catalyst for highly enantioselective trimethylsilylcyanation of aldehydes, J. Org. Chem., 2002, 67, 2702–2704 CrossRef CAS PubMed.
- L. E. Martinez, J. L. Leighton, D. H. Carsten and E. N. Jacobsen, Highly Enantioselective Ring-Opening of Epoxides Catalyzed by (Salen)Cr(Iii) Complexes, J. Am. Chem. Soc., 1995, 117, 5897–5898 CrossRef CAS.
- J. Meléndez, M. North and R. Pasquale, Synthesis of Cyclic Carbonates from Atmospheric Pressure Carbon Dioxide Using Exceptionally Active Aluminium(salen) Complexes as Catalysts, Eur. J. Inorg. Chem., 2007, 2007, 3323–3326 CrossRef.
- M. Taherimehr, A. Decortes, S. M. Al-Amsyar, W. Lueangchaichaweng, C. J. Whiteoak, E. C. Escudero-Adan, A. W. Kleij and P. P. Pescarmona, A highly active Zn(salphen) catalyst for production of organic carbonates in a green CO2 medium, Catal. Sci. Technol., 2012, 2, 2231–2237 RSC.
- M. Balas, S. Beaudoin, A. Proust, F. Launay and R. Villanneau, Advantages of Covalent Immobilization of Metal-Salophen on Amino-Functionalized Mesoporous Silica in Terms of Recycling and Catalytic Activity for CO2 Cycloaddition onto Epoxides, Eur. J. Inorg. Chem., 2021, 2021, 1581–1591 CrossRef CAS.
- S. J. Lee, K. L. Mulfort, J. L. O'Donnell, X. Zuo, A. J. Goshe, P. J. Wesson, S. T. Nguyen, J. T. Hupp and D. M. Tiede, Supramolecular porphyrinic prisms: coordinative assembly and solution phase X-ray structural characterization, Chem. Commun., 2006, 4581–4583, 10.1039/b610025b.
- F. Song, C. Wang, J. M. Falkowski, L. Ma and W. Lin, Isoreticular chiral metal-organic frameworks for asymmetric alkene epoxidation: tuning catalytic activity by controlling framework catenation and varying open channel sizes, J. Am. Chem. Soc., 2010, 132, 15390–15398 CrossRef CAS PubMed.
- J. Jiao, C. Tan, Z. Li, Y. Liu, X. Han and Y. Cui, Design and Assembly of Chiral Coordination Cages for Asymmetric Sequential Reactions, J. Am. Chem. Soc., 2018, 140, 2251–2259 CrossRef CAS PubMed.
- W. Zhou, Q. W. Deng, G. Q. Ren, L. Sun, L. Yang, Y. M. Li, D. Zhai, Y. H. Zhou and W. Q. Deng, Enhanced carbon dioxide conversion at ambient conditions via a pore enrichment effect, Nat. Commun., 2020, 11, 4481 CrossRef CAS PubMed.
- X. Han, Q. Xia, J. Huang, Y. Liu, C. Tan and Y. Cui, Chiral Covalent Organic Frameworks with High Chemical Stability for Heterogeneous Asymmetric Catalysis, J. Am. Chem. Soc., 2017, 139, 8693–8697 CrossRef CAS PubMed.
- T. T. Liu, J. Liang, Y. B. Huang and R. Cao, A bifunctional cationic porous organic polymer based on a Salen-(Al) metalloligand for the cycloaddition of carbon dioxide to produce cyclic carbonates, Chem. Commun., 2016, 52, 13288–13291 RSC.
- J. M. Falkowski, C. Wang, S. Liu and W. Lin, Actuation of asymmetric cyclopropanation catalysts: reversible single-crystal to single-crystal reduction of metal-organic frameworks, Angew. Chem., Int. Ed., 2011, 50, 8674–8678 CrossRef CAS PubMed.
- C. F. Zhu, Q. C. Xia, X. Chen, Y. Liu, X. Du and Y. Cui, Chiral Metal-Organic Framework as a Platform for Cooperative Catalysis in Asymmetric Cyanosilylation of Aldehydes, ACS Catal., 2016, 6, 7590–7596 CrossRef CAS.
- C. Tan, X. Han, Z. Li, Y. Liu and Y. Cui, Controlled Exchange of Achiral Linkers with Chiral Linkers in Zr-Based UiO-68 Metal-Organic Framework, J. Am. Chem. Soc., 2018, 140, 16229–16236 CrossRef CAS PubMed.
- T. T. Liu, J. Liang, R. Xu, Y. B. Huang and R. Cao, Salen-Co(iii) insertion in multivariate cationic metal-organic frameworks for the enhanced cycloaddition reaction of carbon dioxide, Chem. Commun., 2019, 55, 4063–4066 RSC.
- V. Colombo, S. Galli, H. J. Choi, G. D. Han, A. Maspero, G. Palmisano, N. Masciocchi and J. R. Long, High thermal and chemical stability in pyrazolate-bridged metal-organic frameworks with exposed metal sites, Chem. Sci., 2011, 2, 1311–1319 RSC.
- N. Mosca, R. Vismara, J. A. Fernandes, G. Tuci, C. Di Nicola, K. V. Domasevitch, C. Giacobbe, G. Giambastiani, C. Pettinari, M. Aragones-Anglada, P. Z. Moghadam, D. Fairen-Jimenez, A. Rossin and S. Galli, Nitro-functionalized Bis(pyrazolate) Metal-Organic Frameworks as Carbon Dioxide Capture Materials under Ambient Conditions, Chem. – Eur. J., 2018, 24, 13170–13180 CrossRef CAS PubMed.
- R. Vismara, G. Tuci, N. Mosca, K. V. Domasevitch, C. Di Nicola, C. Pettinari, G. Giambastiani, S. Galli and A. Rossin, Amino-decorated bis(pyrazolate) metal-organic frameworks for carbon dioxide capture and green conversion into cyclic carbonates, Inorg. Chem. Front., 2019, 6, 533–545 RSC.
- N. M. Padial, E. Quartapelle Procopio, C. Montoro, E. Lopez, J. E. Oltra, V. Colombo, A. Maspero, N. Masciocchi, S. Galli, I. Senkovska, S. Kaskel, E. Barea and J. A. Navarro, Highly hydrophobic isoreticular porous metal-organic frameworks for the capture of harmful volatile organic compounds, Angew. Chem., Int. Ed., 2013, 52, 8290–8294 CrossRef CAS PubMed.
- N. Masciocchi, S. Galli, V. Colombo, A. Maspero, G. Palmisano, B. Seyyedi, C. Lamberti and S. Bordiga, Cubic octanuclear Ni(II) clusters in highly porous polypyrazolyl-based materials, J. Am. Chem. Soc., 2010, 132, 7902–7904 CrossRef CAS PubMed.
- E. Q. Procopio, S. Rojas, N. M. Padial, S. Galli, N. Masciocchi, F. Linares, D. Miguel, J. E. Oltra, J. A. Navarro and E. Barea, Study of the incorporation and release of the non-conventional half-sandwich ruthenium(II) metallodrug RAPTA-C on a robust MOF, Chem. Commun., 2011, 47, 11751–11753 RSC.
- J. Hu, X. Deng, H. Zhang, Y. Diao, S. Cheng, S. L. Zheng, W. M. Liao, J. He and Z. Xu, Linker Deficiency, Aromatic Ring Fusion, and Electrocatalysis in a Porous Ni8-Pyrazolate Network, Inorg. Chem., 2021, 60, 161–166 CrossRef CAS PubMed.
- S. Galli, A. Maspero, C. Giacobbe, G. Palmisano, L. Nardo, A. Comotti, I. Bassanetti, P. Sozzani and N. Masciocchi, When long bis(pyrazolates) meet late transition metals: structure, stability and adsorption of metal-organic frameworks featuring large parallel channels, J. Mater. Chem. A, 2014, 2, 12208–12221 RSC.
- T. He, Y. Z. Zhang, B. Wang, X. L. Lv, L. H. Xie and J. R. Li, A Base-Resistant Zn(II) -Based Metal-Organic Framework: Synthesis, Structure, Postsynthetic Modification, and Gas Adsorption, ChemPlusChem, 2016, 81, 864–871 CrossRef CAS PubMed.
- T. He, Z. Huang, S. Yuan, X. L. Lv, X. J. Kong, X. Zou, H. C. Zhou and J. R. Li, Kinetically Controlled Reticular Assembly of a Chemically Stable Mesoporous Ni(II)-Pyrazolate Metal-Organic Framework, J. Am. Chem. Soc., 2020, 142, 13491–13499 CrossRef CAS PubMed.
- Y.-N. Gong, D.-C. Zhong and T.-B. Lu, Interpenetrating metal–organic frameworks, CrystEngComm, 2016, 18, 2596–2606 RSC.
- R. R. Shaikh, S. Pornpraprom and V. D'Elia, Catalytic Strategies for the Cycloaddition of Pure, Diluted, and Waste CO2 to Epoxides under Ambient Conditions, ACS Catal., 2017, 8, 419–450 CrossRef.
- J. Lan, Y. Qu, Z. Wang, P. Xu and J. Sun, A facile fabrication of a multi-functional and hierarchical Zn-based MOF as an efficient catalyst for CO2 fixation at room-temperature, Inorg. Chem. Front., 2021, 8, 3085–3095 RSC.
- M. E. Wilhelm, M. H. Anthofer, M. Cokoja, I. I. E. Markovits, W. A. Herrmann and F. E. Kuhn, Cycloaddition of carbon dioxide and epoxides using pentaerythritol and halides as dual catalyst system, ChemSusChem, 2014, 7, 1357–1360 CrossRef CAS PubMed.
- B.-H. Xu, J.-Q. Wang, J. Sun, Y. Huang, J.-P. Zhang, X.-P. Zhang and S.-J. Zhang, Fixation of CO2into cyclic carbonates catalyzed by ionic liquids: a multi-scale approach, Green Chem., 2015, 17, 108–122 RSC.
- Z. Zheng, Z. Wang, Y. Xue, F. He and Y. Li, Selective Conversion of CO2 into Cyclic Carbonate on Atom Level Catalysts, ACS Mater. Au, 2021, 1, 107–115 CrossRef CAS.
- L. Hua, B. Li, C. Han, P. Gao, Y. Wang, D. Yuan and Y. Yao, Synthesis of Homo- and Heteronuclear Rare-Earth Metal Complexes Stabilized by Ethanolamine-Bridged Bis(phenolato) Ligands and Their Application in Catalyzing Reactions of CO2 and Epoxides, Inorg. Chem., 2019, 58, 8775–8786 CrossRef CAS PubMed.
- Y. T. Zheng, X. Q. Wang, C. Liu, B. Q. Yu, W. L. Li, H. L. Wang, T. T. Sun and J. Z. Jiang, Triptycene-supported bimetallic salen porous organic polymers for high efficiency CO2 fixation to cyclic carbonates, Inorg. Chem. Front., 2021, 8, 2880–2888 RSC.
- Y. Z. Li, G. D. Wang, H. Y. Yang, L. Hou, Y. Y. Wang and Z. H. Zhu, Novel cage-like MOF for gas separation, CO2 conversion and selective adsorption of an organic dye, Inorg. Chem. Front., 2020, 7, 746–755 RSC.
- C. He, J. Liang, Y.-H. Zou, J.-D. Yi, Y.-B. Huang and R. Cao, Metal-Organic Frameworks Bonded with Metal N-Heterocyclic Carbenes for efficient catalysis, Natl. Sci. Rev., 2021 DOI:10.1093/nsr/nwab157.
- J. Liang, Q. Wu, Y. B. Huang and R. Cao, Reticular frameworks and their derived materials for CO2 conversion by thermo−catalysis, EnergyChem, 2021, 3, 100064 CrossRef CAS.
- Y. H. Zou, Y. B. Huang, D. H. Si, Q. Yin, Q. J. Wu, Z. Weng and R. Cao, Porous Metal-Organic Framework Liquids for Enhanced CO2 Adsorption and Catalytic Conversion, Angew. Chem., Int. Ed., 2021, 60, 20915–20920 CrossRef CAS PubMed.
- Y. B. N. Tran, P. T. K. Nguyen, Q. T. Luong and K. D. Nguyen, Series of M-MOF-184 (M = Mg, Co, Ni, Zn, Cu, Fe) Metal-Organic Frameworks for Catalysis Cycloaddition of CO2, Inorg. Chem., 2020, 59, 16747–16759 CrossRef CAS PubMed.
Footnote |
† Electronic supplementary information (ESI) available.CCDC 2128369. For ESI and crystallographic data in CIF or other electronic format see DOI: 10.1039/d1qi01555a |
|
This journal is © the Partner Organisations 2022 |
Click here to see how this site uses Cookies. View our privacy policy here.