DOI:
10.1039/D1QI01337H
(Research Article)
Inorg. Chem. Front., 2022,
9, 119-126
Highly red-emissive salen–indium complexes: impact of 4-amino-substitution on the photophysical properties†
Received
22nd October 2021
, Accepted 4th November 2021
First published on 8th November 2021
Abstract
An approach to the design of highly emissive salen–indium complexes is presented. A series of 4-NR2-appended salen ligands possessing an electron-accepting 1,2-dicyanoethylene bridge and their indium complexes (R = Me (1), Et (2), and Ph (3)) were prepared in high yields via a one-pot synthetic pathway. All compounds exhibited narrow-bandwidth red emission (full width at half maximum = 29–42 nm) with high photoluminescence quantum yields (PLQYs, 50%−74%) in toluene. Specifically, 4-NEt2-appended indium complex 2 showed the highest PLQY of 74%, which is among the highest reported for salen-based organometallic luminophores. In contrast, reference complex 4 lacking a 4-amino group was poorly emissive (PLQY < 2%). Theoretical studies suggest that the salen-centered electronic transitions in 1–3 are enhanced by strong electronic interactions between the 4-amino donor and the electron-accepting bridge.
Introduction
Salen (N,N′-bis(salicylidene)-ethylenediamine), which is a type of Schiff base, is one of the most representative chelating platforms to strongly coordinate with various metal ions. It also possesses prominent properties such as a rigid framework, high thermal/chemical stability, and tunable optical properties.1 Because of these unique properties, numerous salen derivatives have been widely used as principal ligands of organometallic complexes.2 Recently, among its versatile applications, salen-based complexes have received considerable attention as a family of promising luminescent materials in the optoelectronic area, such as for organic light-emitting diodes (OLEDs) and photovoltaic cells.3 Thus, various studies have been devoted to the systematic design of salen-based complexes with enhanced photophysical properties.4
Moreover, indium complexes, which are heavier congeners of group 13 main-group complexes based on aluminum4–6 and gallium,2a,6c,7 have recently attracted considerable interest due to their facile complexation with various ligands, including salen, under mild conditions, and their ability to boost luminescent properties.8,9 To date, modification of the electronic structure of salen-based indium complexes has mainly focused on the introduction of functional groups at the C5 position (para position with respect to the oxygen) of the phenoxy ring, together with different bridging units, in the salen ligands.9 However, the effects of such modifications on the enhancement of luminescence properties in terms of emission-color purity and emission quantum efficiency were not remarkable, affording broad and moderately intense emissions, which were only comparable to those found in salen–Al complexes.4–6 Therefore, the search for a breakthrough that can improve the luminescence properties of salen–In complexes remains a challenge.
To tackle this issue, we turned our attention to the alteration of the periphery of salen frameworks at the C4 position, which is the para position with respect to the bridging group.2b,3i,10 Donor–acceptor (D–A)-type salen ligands were also considered to strengthen the electronic interactions between the two moieties. We envisaged that this strategy could enhance the photophysical properties of salen–In complexes, which will allow their potential use as efficient optoelectronic materials.11 In this study, we prepared 4-NR2-substituted salen–indium complexes (R = Me (1), Et (2), and Ph (3)) with a strong electron-accepting bridge, that is, a 1,2-dicyanoethylene bridge (EtCN2). Notably, different from the conventional multistep methods for the preparation of salen-based indium complexes, we newly developed a high-yield, one-pot synthetic route for the synthesis of complexes. Complexes 1–3 exhibit narrow-bandwidth red emissions with high photoluminescence quantum yields (PLQYs) up to 74% for NEt2-substituted complex 2, which is amongst the highest PLQYs for salen-based organometallic complexes reported to date.
Results and discussion
Synthesis and characterization
To prepare 4-NR2-appended salen–indium complexes with an electron-accepting EtCN2 bridging group, we first followed the general procedures9 that use the reaction between InMe3 and a salen ligand, produced by the condensation reaction of diamines with the respective aldehydes. However, only insoluble aggregates were formed. Interestingly, the combination of two equiv. of aldehydes, one equiv. of diaminomaleonitrile, and one equiv. of InMe3 under reflux in EtOH successfully produced indium complexes 1–4 (Scheme 1). These one-pot procedures are highly straightforward and all indium complexes were obtained in high yields (68–74%). The complexes are readily soluble in common organic solvents such as toluene, THF, and CH2Cl2. 1H and 13C NMR spectra (Fig. S1–S3 in the ESI†), and elemental analysis of 1–3 confirmed the formation of all complexes. In particular, the singlet proton resonating at approximately −0.28 ppm confirmed the presence of In–CH3 protons. Furthermore, the crystal structure of 2 clearly revealed the formation of a pentacoordinated monomeric indium species (Fig. 1 and Tables S1 and S2 in the ESI†). Complex 2 adopts a square-pyramidal geometry, as evidenced by the trigonality parameter2g value of 0.005. Notably, the salen framework is nearly coplanar, indicating π-conjugation in the ligand.
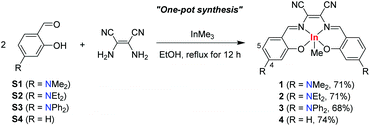 |
| Scheme 1 Synthesis of 1–4. | |
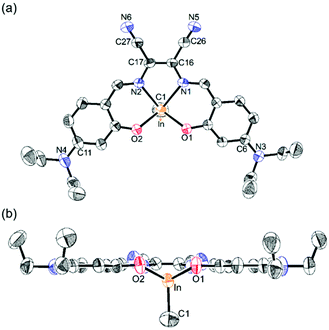 |
| Fig. 1 X-ray crystal structure of 2 (50% thermal ellipsoids): (a) top view and (b) side view. The H atoms are omitted for clarity. | |
Photophysical analysis of indium complexes
To investigate the photophysical properties of complexes 1–3, electronic absorption and emission spectra were recorded in toluene at room temperature (Fig. 2 and Table 1). All complexes exhibited two intense major absorption bands in the high-energy (395–451 nm) and low-energy (590–612 nm) regions. While the high-energy bands are attributed to typical transitions centered on salen moieties, as observed in previous salen–In complexes,9c,d the newly appearing low-energy bands can be attributed to the ππ* transitions on the entire salen ligands owing to the extended π-conjugation. This feature is also similarly observed in complex 4 lacking a 4-NR2 group (Fig. 2 and Table 1). The remarkably red-shifted low-energy absorption in 1–3 further supports strong π-conjugation between the electron-donating NR2 groups and the EtCN2-bridged acceptor. Such electronic interactions may lead to a significant decrease in the energy gap between the highest occupied molecular orbital (HOMO) and the lowest unoccupied molecular orbital (LUMO), causing red-shifted absorption (see computational results below). Indeed, the low-energy absorption of 1–3 is more red-shifted compared to that of 4 (566 nm). For complexes 1–3, the low-energy absorption maxima (λabs) gradually red-shifted as the substituent on the C4 position of the phenoxy ring was varied from NMe2 to NPh2. In addition, the reason for the more red-shifted absorption of 3 compared with 1 and 2 could be attributed to the less electron-donating (HOMO lowering) but strongly conjugating (LUMO lowering) feature of the NPh2 group.
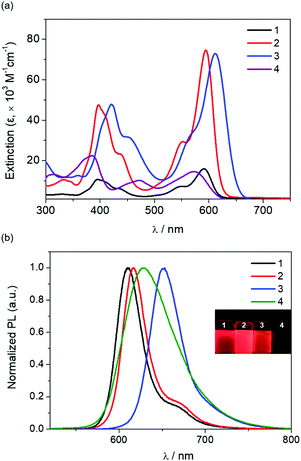 |
| Fig. 2 (a) UV–Vis absorption and (b) PL spectra (λex = 400–420 nm) in toluene (20 μM) of 1–4 at 298 K. The inset photograph shows the emission in toluene taken under a hand-held UV lamp (excitation at 365 nm). | |
Table 1 Photophysical data of indium complexes 1–4
Compd |
λ
abs/nm (ε × 10−3 M−1 cm−1)a |
λ
ex/nm |
λ
em/nm |
FWHM/nmc |
Φ
PL/%d |
τ/nse |
E
ox/Vf |
E
red/Vf |
HOMO/LUMO/eV |
Tola |
Filmb |
Tola |
Tola |
Filmb |
In degassed toluene at 298 K (20 μM).
In spin-coated PMMA film doped with the compound at 1 wt%.
Full width at half maximum.
Absolute photoluminescence quantum yield (PLQY).
Emission lifetime in toluene.
Oxidation and reduction onset potentials with reference to ferrocene/ferrocenium (Fc/Fc+).
Not measured.
Not observed due to low PLQY.
|
1
|
395 (10.56), 436 (6.15), 547 (7.14), 590 (15.88) |
405 |
610 |
603 |
34 |
70.3 |
19.5 |
3.89 |
0.43 |
−1.17 |
−5.23/−3.63 |
2
|
397 (47.46), 437 (23.14), 551 (29.19), 594 (74.65) |
400 |
617 |
612 |
29 |
74.3 |
23.9 |
5.64 |
0.44 |
−1.16 |
−5.24/−3.64 |
3
|
421 (47.80), 451 (31.54), 612 (72.98) |
420 |
652 |
643 |
42 |
49.8 |
33.4 |
3.52 |
0.57 |
−1.01 |
−5.37/−3.79 |
4
|
385 (22.39), 471 (10.20), 572 (14.53) |
400 |
629 |
—g |
69 |
<2.0 |
— |
n/oh |
— |
— |
— |
Electrochemical studies by cyclic voltammetry (CV) indicated that complexes 1–3 underwent irreversible oxidation and reduction processes (Table 1 and Fig. S5†). The onset oxidation (Eox = 0.44 V) and reduction (Ered = −1.16 V) potentials of 1 and 2 were virtually the same, while those of 3 anodically shifted (Eox = 0.57 V and Ered = −1.01 V). This can be associated with the electronic effects of the 4-NPh2 group, which is less electron-donating but strongly conjugates with the EtCN2-acceptor. Indeed, the electrochemical oxidation and reduction of 3, which exhibited slightly more stabilization of LUMO (ca. 0.15 eV) than HOMO (ca. 0.13 eV) compared with 1 and 2 led to the reduction of the band gap by 0.02 eV.
Next, the photoluminescence (PL) spectra of complexes 1–3 exhibited narrow red emissions centered at 610–652 nm in toluene solutions (Fig. 2 and Table 1). This emission feature is somewhat different from the broad emission of 4 (green line), which is similar to that appearing in usual salen-based complexes.9c,d Indeed, the full width at half maximum (FWHM) values of the PL emissions were very small for 1–3, ranging from 29–42 nm, whereas complex 4 showed a 71 nm FWHM. The weak shoulders over 650 nm for 1 and 2 and tail over 700 nm for 3 indicate that ππ* excited states are mainly responsible for the emissions of 1–3. The emission maxima (λem) shifted towards longer wavelengths in the order of 1 < 2 < 3, which is in accordance with their low-energy absorptions. In addition, the emission spectrum of 2 underwent slight bathochromic shifts in polar solvents such as MeCN and MeOH (∼4 nm), indicating weak CT characteristics (Fig. S6 and Table S3†). The single-exponential PL decay profiles with nanosecond-range emission lifetimes (τ) confirm that all emissions are fluorescence in nature (Fig. S7†). The PLQYs (ΦPL) of 1–3 in oxygen-free toluene solutions exhibited high values, and remarkably, 4-NEt2-substituted 2 had the highest PLQY of 74%, which is the highest among the reported PLQYs of indium complexes. The PLQY of 4-NMe2-substituted 1 (ΦPL = 70%) was slightly lower than that of 2, whereas 4-NPh2-substituted 3 exhibited a somewhat decreased PLQY (ΦPL = 50%). In contrast, unsubstituted reference complex 4 showed only 2% PLQY. These results demonstrate that in combination with a strong electron-accepting bridge in the salen ligands, and the introduction of electron-donating substituents at the C4 position of the phenoxy ring leads to a significant enhancement of PLQY. The high PLQY of 2 was also retained in polar solvents (ΦPL = 68% in MeCN and 44% in MeOH). Moreover, both powder and PMMA films doped with a high concentration (10 wt%) of 1–3 were poorly emissive, implying that aggregation-caused quenching (ACQ) occurred in the rigid state. However, the PMMA films doped with a low concentration (1 wt%) became moderately emissive (ΦPL = 20%–33%). PLQYs also increased in the order of 1 < 2 < 3 in the film state, indicating the suppression of ACQ by the steric bulkiness of the 4-NR2 substituent. Although slightly rigidochromic blue-shifts (∼8 nm) were observed in the film states of 1–3, the emission profiles were similar to those in toluene above (Fig. S8†). Accordingly, these findings demonstrate that the strategic design of indium complexes supported by D–A-type salen ligand platforms can achieve highly efficient red emissions with good color purity.
Theoretical calculations and orbital analysis
To elucidate the nature of the electronic transitions of 1–4, computational studies based on time-dependent density functional theory (TD-DFT) were performed on the ground state (S0)- and first singlet excited state (S1)-optimized structures of the complexes at the B3LYP/6-31G(d, p) level (Fig. 3, Table 2, Fig. S9–S13, and Tables S4–S13†). In the S0 state, the low-energy absorptions of 1–3 are mainly associated with the HOMO → LUMO transition (Fig. S9 and Table S4†). Both the HOMO and LUMO of 1–3 are distributed over the 4-NR2-appended salen ligands, characterizing the absorption process as the ππ* transition.
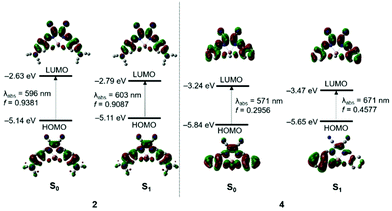 |
| Fig. 3 Frontier MOs of 2 and 4 in their S0- and S1-states with their relative energies from DFT calculations (isovalue 0.04). The transition energy (in nm) was calculated using the TD-B3LYP method at the 6-31G(d, p) level. | |
Table 2 Major electronic transitions of 2 and 4 calculated for their S0 and S1 optimized structures using the TD-B3LYP method at the 6-31G(d, p) levela
|
States |
λ
calc/nm |
f
calc
|
Assignment |
Singlet energies for the vertical transition calculated for the S0 and S1 optimized geometries.
|
2
|
S0 |
595.91 |
0.9381 |
HOMO → LUMO (99%) |
S1 |
605.58 |
0.9087 |
HOMO → LUMO (98%) |
4
|
S0 |
570.52 |
0.2956 |
HOMO → LUMO (99%) |
S1 |
670.58 |
0.4577 |
HOMO → LUMO (99%) |
The 4-NR2 substituents have moderate electronic contributions of ca. 20%–40% to the HOMOs depending on the nature of the NR2 group. Owing to the large spatial overlap between the HOMO and LUMO, a high oscillator strength was calculated for the absorption in 1–3 (fcalc = 0.89–1.01). In the case of complex 4 lacking a 4-NR2 substituent, the similar HOMO → LUMO transition is mainly associated with the low-energy absorption. HOMO is primarily located on the phenoxy ring (72%), whereas LUMO is distributed over the EtCN2 imine fragment (44%) with a reduced contribution from the phenoxy ring (55%) due to the large decrease in the contribution from the oxygen atom (Table S13†). Notably, the absorption oscillator strength of 4 is largely decreased (fcalc = 0.30), exhibiting a value one-third of those obtained for 1–3. The calculated low-energy absorption wavelengths are in the order of 4 (571 nm) < 1 (593 nm) < 2 (596 nm) < 3 (615 nm) in toluene, which is the same trend as that of the experimental absorption data (Table S4†).
In the S1 state, the lowest-energy emissions of 1–3 arise from the HOMO → LUMO transition whose MO distributions are very similar to those of the absorptions (Fig. S10 and Table S5†). The emission of 4 is also assignable to the HOMO → LUMO transition (Fig. 3 and Table 2). However, the HOMO in the S1 state of 4 has a predominant contribution from the phenoxy ring, while the LUMO is delocalized over the salen ligand. Thus, the emission originates from a salen-centered ππ* transition mixed with a partial CT between the phenoxy ring and EtCN2 imine fragment (Table S13†). In fact, this feature is similar to those previously reported for salen–indium luminophores9c,d and supports the broad emission of 4. In particular, the oscillator strengths of the emissions for 1–3 (fcalc = 0.62–0.91), which are on the same order as their PLQYs, are greater than that calculated for 4 (fcalc = 0.46) (Table S5†). This result is also in qualitative agreement with the higher PLQYs of 1–3 compared with that of 4. Finally, the computed emission wavelengths are in the order of 1 (603 nm) < 2 (606 nm) < 3 (615 nm). The emission wavelength of 4 is somewhat red-shifted due to the CT character of the emission (Table S5†). Therefore, these results support the experimentally observed emission wavelengths and bathochromic shifts, high PLQYs, and narrow bandwidth emission for 1–3.
Experimental
General considerations
All manipulations were carried out under an inert N2 atmosphere using standard Schlenk and glove box techniques. All anhydrous grade solvents (dichloromethane, dimethylformamide (DMF), toluene, ethanol (EtOH), diethyl ether) purchased from Alfa Aesar were dried by passing them through an activated alumina column and stored over activated molecular sieves (5 Å). Spectrophotometric-grade solvents (toluene, acetonitrile (MeCN), and methanol (MeOH)) were used as received. Compounds S1,12S3,13 and trimethylindium (InMe3)8,9 were analogously prepared according to literature procedures. Commercial reagents (4-(diethylamino)salicylaldehyde (S2), salicylaldehyde (S4), and diaminomaleonitrile) were purchased and used without any further purification. Deuterated solvent (CDCl3, chloroform-d1) from Cambridge Isotope Laboratories was used after drying over activated molecular sieves (5 Å). NMR spectra were recorded on a Bruker Avance 400 spectrometer (400.13 MHz for 1H and 100.62 MHz for 13C) at ambient temperature. Chemical shifts are given in ppm, and are referenced against external Me4Si (1H and 13C). Elemental analyses were performed on an EA3000 (Eurovector). Cyclic voltammetry experiments were carried out using an Autolab/PGSTAT101 system.
Synthesis of 1
A reaction mixture of 4-(dimethylamino)salicylaldehyde (S1) (0.18 g, 1.1 mmol), diaminomaleonitrile (0.054 g, 0.50 mmol), and InMe3 (0.088 g, 0.55 mmol) in EtOH (20 mL) was refluxed for 12 h. After cooling to room temperature, all the volatiles were removed under reduced pressure. The residue was washed with 30 mL of anhydrous diethyl ether and dried in vacuo to give a dark green solid (0.19 g, 71%). 1H NMR (CDCl3): δ 8.00 (s, 2H), 6.96 (d, J = 8.0 Hz, 2H), 6.24 (d, J = 8.0 Hz, 2H), 6.14 (s, 2H), 3.07 (s, 12H), −0.25 (s, 3H, In–CH3). 13C NMR (CDCl3): δ 173.84, 159.25, 157.55, 138.22, 119.05, 112.36, 111.59, 106.23, 103.00, 40.13, 5.04 (In–CH3). Anal. calcd for C23H23InN6O2: C, 52.09; H, 4.37; N, 15.85. Found: C, 52.01; H, 4.36; N, 15.58.
Synthesis of 2
This compound was prepared in a manner analogous to the synthesis of 1 using 4-(diethylamino)salicylaldehyde (S2) (0.20 g, 1.1 mmol). The desired product 2 was obtained as a dark green solid (0.21 g, 71%). 1H NMR (CDCl3): δ 7.96 (s, 2H), 6.93 (d, J = 8.0 Hz, 2H), 6.19 (d, J = 8.0 Hz, 2H), 6.16 (s, 2H), 3.39 (m, 8H), 1.20 (t, J = 8.0 Hz, 12H), −0.26 (s, 3H, In–CH3). 13C NMR (CDCl3): δ 173.81, 158.52, 155.58, 138.41, 118.74, 112.15, 111.70, 106.06, 102.29, 44.90, 12.91. Anal. calcd for C27H31InN6O2: C, 55.30; H, 5.33; N, 14.33. Found: C, 55.31; H, 5.07; N, 14.05.
Synthesis of 3
This compound was prepared in a manner analogous to the synthesis of 1 using S3 (0.32 g, 1.1 mmol). The desired product 3 was obtained as a dark green solid (0.26 g, 68%). 1H NMR (CDCl3): δ 8.04 (s, 2H), 7.30 (dd, J = 8.0 Hz, 8H), 7.16 (m, 12H), 6.90 (d, J = 8.0 Hz, 2H), 6.30 (s, 2H), 6.28 (d, J = 8.0 Hz, 2H), −0.23 (s, 3H, In–CH3). 13C NMR (CDCl3): δ 174.18, 159.99, 157.06, 144.90, 137.46, 129.64, 127.35, 126.11, 119.68, 114.16, 111.14, 111.07, 109.88. Anal. calcd for C43H31InN6O2: C, 66.34; H, 4.01; N, 10.80. Found: C, 66.10; H, 4.01; N, 10.59.
Synthesis of 4
This compound was prepared in a manner analogous to the synthesis of 1 using salicylaldehyde (S4) (0.13 g, 1.1 mmol). The desired product 4 was obtained as a dark brown solid (0.16 g, 74%). 1H NMR (CDCl3): δ 8.53 (s, 2H), 7.40 (m, 4H), 6.83 (d, J = 8.0 Hz, 2H), 6.67 (m, 2H), −0.24 (s, 3H, In–CH3). 13C NMR (CDCl3): δ 175.02, 159.74, 156.79, 139.62, 119.95, 113.36, 111.92, 107.27, 103.50. Anal. calcd for C19H13InN4O2: C, 51.38; H, 2.95; N, 12.61. Found: C, 51.60; H, 2.96; N, 12.45.
Photophysical measurements
The UV–Vis absorption and photoluminescence (PL) spectra were recorded on a Varian Cary 100 and FS5 spectrophotometer, respectively. Solution PL spectra were obtained from oxygen-free solutions (20 μM in toluene, MeCN, and MeOH). Oxygen-free solvents were prepared by degassing the spectroscopic-grade solvents for ca. 30 min and kept in a nitrogen-filled glove box. PL spectra and absolute photoluminescence quantum yields (PLQYs, ΦPL) of film were obtained with poly(methyl methacrylate) (PMMA) matrices doped with compounds on quatz plates. Absolute PLQYs of solutions and films were measured on an absolute PL quantum yield spectrophotometer (Quantaurus-QY C11347-11, Hamamatsu Photonics) equipped with a 3.3-inch integrating sphere. PL decays were measured on a FS5 spectrophotometer (Edinburgh Instruments) using time-correlated single-photon counting (TCSPC) mode (with an EPL-375 picosecond pulsed diode laser as a light source). The lifetimes of fluorescence (τ) were estimated by fitting decay curves measured from a TCSPC mode. The HOMO and LUMO energy levels were estimated from the electrochemical oxidation and reduction (Eonset or E1/2) peaks of cyclic voltammograms.
Theoretical calculations
The optimized structures of 1–4 in the ground (S0) and first excited (S1) state were determined by the density functional theory (DFT) method with the B3LYP functional14 and 6-31G(d,p) levels.15 The basis sets in 6-31G(d,p) were used for other atoms except for the central indium atoms. The LANL2DZ effective core potentials (ECPs) and corresponding basis sets were used to handle the indium atoms.16 The electronic transition energies including an account of electron correlation were obtained from TD-DFT17 calculations using the hybrid B3LYP functional (TD-B3LYP). All calculations were performed using the GAUSSIAN 16 program package.18 The GaussSum 3.0 was used to calculate the percent contribution of a group in a molecule to each molecular orbital.19
Conclusions
In summary, we demonstrated that 4-NR2-appended salen–indium complexes (R = Me (1), Et (2), and Ph (3)) with an electron-accepting EtCN2 bridge can be prepared via a facile one-pot synthetic method and they are highly emissive with narrow PL emission bandwidths. The X-ray crystal structure of 2 revealed a planar π-conjugated arrangement in the salen ligand. Along with this structural feature, the donor–acceptor-type salen ligand induced intense red emission with high PLQY in the solution state. Notably, complex 2 with a 4-NEt2-substituent exhibited the highest PLQY (ΦPL = 76%) among the indium complexes reported to date. The PL emission was also very narrow owing to the diminished CT character of the electronic transition. These photophysical results were further supported by TD-DFT calculations. We believe that the findings in this study may provide insights into the development of highly luminescent salen–indium complexes for optoelectronic applications.
Conflicts of interest
There are no conflicts to declare.
Acknowledgements
This work was supported by the Basic Science Research Program funded by the Ministry of Science and ICT (MSIT) (NRF-2019R1A2C1009969 for M. H. Park and NRF-2019R1A2C1084952 for M. H. Lee) through the National Research Foundation of Korea (NRF) funded by the Ministry of Science and ICT.
References
-
(a) M. Andruch, Compartmental Schiff-base ligands—a rich library of tectons in designing magnetic and luminescent materials, Chem. Commun., 2011, 47, 3025–3042 RSC;
(b) P. A. Vigato and S. Tamburini, The challenge of cyclic and acyclic schiff bases and related derivatives, Coord. Chem. Rev., 2004, 248, 1717–2128 CrossRef CAS.
-
(a) H.-Y. Yin, J.-J. Gao, X. Chen, B. Ma, Z.-S. Yang, J. Tang, B.-W. Wang, T. Chen, C. Wang, S. Gao and J.-L. Zhang, A Gallium(III) Complex that Engages Protein Disulfide Isomerase A3 (PDIA3) as an Anticancer Target, Angew. Chem., Int. Ed., 2020, 59, 20147–20153 CrossRef CAS PubMed;
(b) D. Xie, J. Jing, Y.-B. Cai, J. Tang, J.-J. Chen and J.-L. Zhang, Construction of an orthogonal ZnSalen/Salophen library as a colour palette for one- and two-photon live cell imaging, Chem. Sci., 2014, 5, 2318–2327 RSC;
(c) J. Jing, J.-J. Chen, Y. Hai, J. Zhan, P. Xu and J.-L. Zhang, Rational design of ZnSalen as a single and two photon activatable fluorophore in living cells, Chem. Sci., 2012, 3, 3315–3320 RSC;
(d) P. Wu, D. L. Ma, C. H. Leung, S. C. Yan, N. Y. Zhu, R. Abagyan and C. M. Che, Stabilization of G-Quadruplex DNA with Platinum(II) Schiff Base Complexes: Luminescent Probe and Down-Regulation of c-myc Oncogene Expression, Chem. – Eur. J., 2009, 15, 13008–13021 CrossRef CAS PubMed;
(e) K. C. Gupta and A. K. Sutar, Catalytic activities of Schiff base transition metal complexes, Coord. Chem. Rev., 2008, 252, 1420–1450 CrossRef CAS;
(f) P. G. Cozzi, Metal–Salen Schiff base complexes in catalysis: practical aspects, Coord. Chem. Rev., 2004, 33, 410–421 CAS;
(g) D. A. Atwood and M. J. Harvey, Group 13 Compounds Incorporating Salen Ligands, Chem. Rev., 2001, 101, 37–52 CrossRef CAS PubMed;
(h) E. Lamour, S. Routier, J. L. Bernier, J. P. Catteau, C. Bailly and H. Vezin, Oxidation of CuII to CuIII, Free Radical Production, and DNA Cleavage by Hydroxy-salen–Copper Complexes. Isomeric Effects Studied by ESR and Electrochemistry, J. Am. Chem. Soc., 1999, 121, 1862–1869 CrossRef CAS.
-
(a) J. Zhang, F. C. Zhao, X. J. Zhu, W. K. Wong, D. G. Ma and W. Y. Wong, New phosphorescent platinum(II) Schiff base complexes for PHOLED applications, J. Mater. Chem., 2012, 22, 16448–16457 RSC;
(b) H. Yersin, A. F. Rausch, R. Czerwieniec, T. Hofback and T. Fischer, The triplet state of organo-transition metal compounds. Triplet harvesting and singlet harvesting for efficient OLEDs, Coord. Chem. Rev., 2011, 255, 2622–2652 CrossRef CAS;
(c) C. M. Che, C. C. Kwok, S. W. Lai, A. F. Rausch, W. J. Finkenzeller, N. Y. Zhu and H. Yersin, Photophysical Properties and OLED Applications of Phosphorescent Platinum(II) Schiff Base Complexes, Chem. – Eur. J., 2010, 16, 233–247 CrossRef CAS PubMed;
(d) J. O. Huh, M. H. Lee, H. Jang, K. Y. Hwang, J. S. Lee, S. H. Kim and Y. Do, A Novel Solution-Processible Heterodinuclear AlIII/IrIII Complex for Host–Dopant Assembly OLEDs, Inorg. Chem., 2008, 47, 6566–6568 CrossRef CAS;
(e) K. Y. Hwang, M. H. Lee, H. Jang, Y. Sung, J. S. Lee, S. H. Kim and Y. Do, Aluminium–salen luminophores as new hole-blocking materials for phosphorescent OLEDs, Dalton Trans., 2008, 1818–1820 RSC;
(f) F. Galbrecht, X. H. Yang, B. S. Nehls, D. Neher, T. Farrell and U. Scherf, Semiconducting polyfluorenes with electrophosphorescent on-chain platinum–salen chromophores, Chem. Commun., 2005, 2378–2380 RSC;
(g) H. F. Xiang, S. C. Chan, K. K. Y. Wu, C. M. Che and P. T. Lai, High-efficiency red electrophosphorescence based on neutral bis(pyrrole)-diimine platinum(II) complex, Chem. Commun., 2005, 1408–1410 RSC;
(h) C. M. Che, S. C. Chan, H. F. Xiang, M. C. W. Chan, Y. Liu and Y. Wang, Tetradentate Schiff base platinum(II) complexes as new class of phosphorescent materials for high-efficiency and white-light electroluminescent devices, Chem. Commun., 2004, 1484–1485 RSC;
(i) P. Wang, Z. Hong, Z. Xie, S. Tong, O. Wong, C.-S. Lee, N. Wong, L. Hung and S. Lee, A bis-salicylaldiminato Schiff base and its zinc complex as new highly fluorescent red dopants for high performance organic electroluminescence devices, Chem. Commun., 2003, 1664–1665 RSC.
-
(a) V. Béreau, C. Duhayon, A. Sournia-Saquet and J.-P. Sutter, Tuning of the emission efficiency and HOMO-LUMO band gap for ester-functionalized {Al(salophen)(H2O)2}+ blue luminophors, Inorg. Chem., 2012, 51, 1309–1318 CrossRef;
(b) V. Béreau, V. Jubéra, P. Arnaud, A. Kaiba, P. Guionneau and J.-P. Sutter, Modulation of the luminescence quantum efficiency for blue luminophor {Al(salophen)}+ by ester-substituents, Dalton Trans., 2010, 39, 2070–2077 RSC;
(c) K. Y. Hwang, H. Kim, Y. S. Lee, M. H. Lee and Y. Do, Synthesis and Properties of Salen–Aluminum Complexes as a Novel Class of Color-Tunable Luminophores, Chem. – Eur. J., 2009, 15, 6478–6487 CrossRef CAS PubMed.
-
(a) S. W. Kwak, H. Jin, J. H. Lee, H. Hwang, M. Kim, Y. Kim, Y. Chung, K. M. Lee and M. H. Park, Systematic Control of the Overlapping Energy Region for an Efficient Intramolecular Energy Transfer: Functionalized Salen-Al/Triphenylamine Guest-Host Assemblies, Inorg. Chem., 2019, 58, 2454–2462 CrossRef CAS PubMed;
(b) S. W. Kwak, H. Jin, H. Shin, J. H. Lee, H. Hwang, J. Lee, M. Kim, Y. Chung, Y. Kim, K. M. Lee and M. H. Park, A salen–Al/carbazole dyad-based guest-host assembly: enhancement of luminescence efficiency via intramolecular energy transfer, Chem. Commun., 2018, 54, 4712–4715 RSC;
(c) S. W. Kwak, B. H. Choi, J. H. Lee, H. Hwang, J. Lee, H. Kwon, Y. Chung, K. M. Lee and M. H. Park, Synthesis and dual-emission feature of salen-Al/triarylborane dyads, Inorg. Chem., 2017, 56, 6039–6043 CrossRef CAS;
(d) C. Sohn, J. Jeong, J. H. Lee, B. H. Choi, H. Hwang, G.-T. Bae, K. M. Lee and M. H. Park, Novel aluminium-BODIPY dyads: intriguing dual-emission via photoinduced energy transfer, Dalton Trans., 2016, 45, 5825–5832 RSC.
-
(a) N. Lin, J. Qiao, L. Duan, J. Xue and L. Wang, Rational design of chelated aluminum complexes toward highly efficient and thermally stable electron-transporting materials, Chem. Mater., 2014, 26, 3693–3700 CrossRef CAS;
(b) C. Pérez-Bolívar, S.-y. Takizawa, G. Nishimura, V. A. Montes and P. Anzenbacher, Jr., High-Efficiency Tris(8-hydroxyquinoline)aluminum (Alq3) Complexes for Organic White-Light-Emitting Diodes and Solid-State Lighting, Chem. – Eur. J., 2011, 17, 9076–9082 CrossRef;
(c) S.-H. Liao, J.-R. Shiu, S.-W. Liu, S.-J. Yeh, Y.-H. Chen, C.-T. Chen, T. J. Chow and C.-I. Wu, Hydroxynaphthyridine-derived group III metal chelates: wide band gap and deep blue analogues of green Alq3 (tris(8-hydroxyquinolate)aluminum) and their versatile applications for organic light-emitting diodes, J. Am. Chem. Soc., 2009, 131, 763–777 CrossRef CAS;
(d) S. Wang, Luminescence and electroluminescence of Al(III), B(III), Be(II) and Zn(II) complexes with nitrogen donors, Coord. Chem.
Rev., 2001, 215, 79–98 CrossRef CAS.
-
(a) M. K. Pal, N. Kushwah, D. Manna, A. Wadawale, V. Sudarsan, T. K. Ghanty and V. K. Jain, Organometallics, 2013, 32, 104–111 CrossRef CAS;
(b) N. Kushwah, M. K. Pal, A. Wadawale, V. Sudarsan, D. Manna, T. K. Ghanty and V. K. Jain, Diorgano-Gallium and -Indium Complexes Derived from Benzoazole Ligands: Synthesis, Characterization, Photoluminescence, and Computational Studies, Organometallics, 2012, 31, 3836–3843 CrossRef CAS;
(c) Y.-H. Song, Y.-C. Chiu, Y. Chi, P.-T. Chou, Y.-M. Cheng, C.-W. Lin, G.-H. Lee and A. J. Carty, Synthesis, Characterization, and Photophysical Properties of Luminescent Gallium and Indium Complexes Constructed using Tridentate 6-Azolyl-2,2′-bipyridine Chelates, Organometallics, 2008, 27, 80–87 CrossRef CAS.
- S. W. Kwak, M. B. Kim, H. Shin, J. H. Lee, H. Hwang, J. Y. Ryu, J. Lee, M. Kim, Y. Chung, J. C. Choe, Y. Kim, K. M. Lee and M. H. Park, A Series of Quinolinol-Based Indium Luminophores: A Rational Design Approach for Manipulating Photophysical Properties, Inorg. Chem., 2019, 58, 8056–8063 CrossRef.
-
(a) C. H. Ryu, S. W. Kwak, H. W. Lee, J. H. Lee, H. Hwang, M. Kim, Y. Chung, Y. Kim, M. H. Park and K. M. Lee, Carbazole-Appended Salen–Indium Conjugate Systems: Synthesis and Enhanced Luminescence Efficiency, Inorg. Chem., 2019, 58, 12358–12364 CrossRef CAS;
(b) S. W. Kwak, H. Kwon, J. H. Lee, H. Hwang, M. Kim, Y. Chung, Y. Kim, K. M. Lee and M. H. Park, Salen-indium/triarylborane triads: synthesis and ratiometric emission-colour changes by fluoride ion binding, Dalton Trans., 2018, 47, 5310–5317 RSC;
(c) K. Hyun, H. Jin, W. H. Woo, H. Shin, J. H. Lee, H. Hwang, M. Kim, K. M. Lee, M. H. Park and Y. Kim, Systematic design of indium-based luminophores with color-tunable emission via combined manipulation of HOMO and LUMO levels, Dyes Pigm., 2018, 158, 285–294 CrossRef CAS;
(d) S. H. Lee, N. Shin, S. W. Kwak, K. Hyun, W. H. Woo, J. H. Lee, H. Hwang, M. Kim, J. Lee, Y. Kim, K. M. Lee and M. H. Park, Intriguing indium-salen complexes as multicolor luminophores, Inorg. Chem., 2017, 56, 2621–2626 CrossRef CAS PubMed.
-
(a) J. Cheng, F. Gou, X. Zhang, G. Shen, X. Zhou and H. Xiang, A Class of Multiresponsive Colorimetric and Fluorescent pH Probes via Three Different Reaction Mechanisms of Salen Complexes: A Selective and Accurate pH Measurement, Inorg. Chem., 2016, 55, 9221–9229 CrossRef CAS;
(b) J. Cheng, X. Ma, Y. Zhang, J. Liu, X. Zhou and H. Xiang, Optical Chemosensors Based on Transmetalation of Salen-Based Schiff Base Complexes, Inorg. Chem., 2014, 53, 3210–3219 CrossRef CAS PubMed;
(c) J. Cheng, K. Wei, X. Ma, X. Zhou and H. Xiang, Synthesis and Photophysical Properties of Colorful Salen-Type Schiff Bases, J. Phys. Chem. C, 2013, 117, 16552–16563 CrossRef CAS.
-
(a) A. K.-W. Chan, M. Ng, Y.-C. Wong, M.-Y. Chan, W.-T. Wong and V. W.-W. Yam, Synthesis and characterization of luminescent cyclometalated platinum(II) complexes with tunable emissive colors and studies of their application in organic memories and organic light-emitting devices, J. Am. Chem. Soc., 2017, 139, 10750–10761 CrossRef CAS;
(b) C.-H. Lee, M.-C. Tang, Y.-C. Wong, M.-Y. Chan and V. W.-W. Yam, Sky-Blue-Emitting Dendritic Alkynylgold(III) Complexes for Solution-Processable Organic Light-Emitting Devices, J. Am. Chem. Soc., 2017, 139, 10539–10550 CrossRef CAS PubMed;
(c) F. K.-W. Kong, M.-C. Tang, Y.-C. Wong, M. Ng, M.-Y. Chan and V. W.-W. Yam, Strategy for the Realization of Efficient Solution-Processable Phosphorescent Organic Light-Emitting Devices: Design and Synthesis of Bipolar Alkynylplatinum(II) Complexes, J. Am. Chem. Soc., 2017, 139, 6351–6362 CrossRef CAS;
(d) F. K.-W. Kong, M.-C. Tang, Y.-C. Wong, M.-Y. Chan and V. W.-W. Yam, Design Strategy for High-Performance Dendritic Carbazole-Containing Alkynylplatinum(II) Complexes and Their Application in Solution-Processable Organic Light-Emitting Devices, J. Am. Chem. Soc., 2016, 138, 6281–6291 CrossRef CAS PubMed;
(e) M.-C. Tang, D. P.-K. Tsang, M. M.-Y. Chan, K. M.-C. Wong and V. W.-W. Yam, Dendritic Luminescent Gold(III) Complexes for Highly Efficient Solution-Processable Organic Light-Emitting Devices, Angew. Chem., Int. Ed., 2013, 52, 446–449 CrossRef CAS PubMed.
- L. Fan, X. Wang, J. Ge, F. Li, X. Wang, J. Wang, S. Shuang and C. Dong, A lysosome-targeting and polarity-specific fluorescent probe for cancer diagnosis, Chem. Commun., 2019, 55, 4703–4706 RSC.
- S. S. Ali, A. Gangopadhyay, A. K. Pramanik, U. N. Guria, S. K. Samanta and A. K. Mahapatra, Ratiometric sensing of nerve agent mimic DCP through in situ benzisoxazole formation, Dyes Pigm., 2019, 170, 107585 CrossRef CAS.
- P. J. Stephens, F. J. Devlin, C. F. Chabalowski and M. J. Frisch, Ab initio calculation of vibrational absorption and circular dichroism spectra using density functional force fields, J. Phys. Chem., 1994, 98, 11623–11627 CrossRef CAS.
- C. Lee, W. Yang and R. G. Parr, Development of the Colle-Salvetti correlation-energy formula into a functional of the electron density, Phys. Rev. B: Condens. Matter Mater. Phys., 1988, 37, 785–789 CrossRef CAS.
- P. J. Hay and W. R. Wadt, Ab initio effective core potentials for molecular calculations. Potentials for the transition metal atoms Sc to Hg, J. Chem. Phys., 1985, 82, 270–283 CrossRef CAS.
- E. Runge and E. K. U. Gross, Density-functional theory for time-dependent systems, Phys. Rev. Lett., 1984, 52, 997–1000 CrossRef CAS.
-
M. J. Frisch, G. W. Trucks, H. B. Schlegel, G. E. Scuseria, M. A. Robb, J. R. Cheeseman, G. Scalmani, V. Barone, G. A. Petersson, H. Nakatsuji, X. Li, M. Caricato, A. V. Marenich, J. Bloino, B. G. Janesko, R. Gomperts, B. Mennucci, H. P. Hratchian, J. V. Ortiz, A. F. Izmaylov, J. L. Sonnenberg, D. Williams-Young, F. Ding, F. Lipparini, F. Egidi, J. Goings, B. Peng, A. Petrone, T. Henderson, D. Ranasinghe, V. G. Zakrzewski, J. Gao, N. Rega, G. Zheng, W. Liang, M. Hada, M. Ehara, K. Toyota, R. Fukuda, J. Hasegawa, M. Ishida, T. Nakajima, Y. Honda, O. Kitao, H. Nakai, T. Vreven, K. Throssell, J. A. Montgomery Jr., J. E. Peralta, F. Ogliaro, M. J. Bearpark, J. J. Heyd, E. N. Brothers, K. N. Kudin, V. N. Staroverov, T. A. Keith, R. Kobayashi, J. Normand, K. Raghavachari, A. P. Rendell, J. C. Burant, S. S. Iyengar, J. Tomasi, M. Cossi, J. M. Millam, M. Klene, C. Adamo, R. Cammi, J. W. Ochterski, R. L. Martin, K. Morokuma, O. Farkas, J. B. Foresman and D. J. Fox, Gaussian 16 Revision B.01, Gaussian. Inc., Wallingford, CT, 2016 Search PubMed.
- N. M. O'Boyle, A. L. Tenderholt and K. M. Langner, A library for package independent computational chemistry algorithms, J. Comput. Chem., 2008, 29, 839–845 CrossRef.
Footnotes |
† Electronic supplementary information (ESI) available: Characterization data (1H and 13C NMR spectra), X-ray cryatallographic, spectroscopic, and computational data for all In complexes. CCDC 2091666. For ESI and crystallographic data in CIF or other electronic format see DOI: 10.1039/d1qi01337h |
‡ The first and second authors contributed equally to this work. |
|
This journal is © the Partner Organisations 2022 |
Click here to see how this site uses Cookies. View our privacy policy here.