DOI:
10.1039/D1PY01167G
(Review Article)
Polym. Chem., 2022,
13, 8-43
AIE-active macromolecules: designs, performances, and applications
Received
28th August 2021
, Accepted 22nd November 2021
First published on 23rd November 2021
Abstract
Macromolecules with aggregation-induced emission (AIE) characteristics as emerging soft luminescent materials have gained increasing attention owing to their facile formulation, good processability, high brightness in aggregate and solid states, wide functionality, and smart responsiveness, with great potential in an array of application fields. In this review, we systematically update the most recent advances in different AIE-active macromolecules like linear, hyperbranched, star-shaped, crosslinked, supramolecular, porous, and hybrid macromolecules. The special focus is on revealing the relationship between structures and properties, and how the AIE-active macromolecules take an important role in diverse applications like sensors, optoelectronics, therapeutics, printing, bioimaging, pollution elimination, and stimuli-responsive devices. We hope this review serves not only as a handbook for interested students, scientists, etc., but also and more importantly triggers significant progress of AIE-active macromolecules in the future.
1. Introduction
Aggregation-induced emission (AIE) refers to the phenomenon that certain luminophores (mainly fluorophores) exhibit low or virtually no photoemission upon radiative excitation in the molecular state but become highly emissive when aggregated (e.g., when forming aggregates in solution, in solid state, etc.).1 Such behaviour is quite abnormal when compared with many other traditional fluorophores. Conventional fluorophores usually face a massive barrier in their applications and developments, named aggregation-caused quenching (ACQ), which is the total converse of the AIE effect. ACQ describes the phenomenon that some luminophores are highly emissive in the mono-dispersed state but see a reduced emission intensity or even have their fluorescence almost totally quenched when aggregated.2 Obstructed by this inevitable barrier, applications requiring high fluorophore concentrations have been in deep trouble for ACQ-affected luminophores. However, the accidental discovery of the AIE effect in 2001 by Tang et al. opened up a path on which we can nullify problems caused by ACQ and use aggregation as a powerful tool.1
For luminophores, aggregation brings both ACQ and AIE effects which are in competition with each other. Whether AIE or ACQ dominates depends on many factors including molecular structures, how the molecules arrange themselves in the aggregated states, etc. Mechanistic inspections have suggested several hypotheses to rationalize the source of the AIE effect thus explaining why some luminophores are ACQ-dominated, while others are AIE-active. Among these hypotheses, the most widely accepted one is the restriction of intramolecular motion (RIM) theory.3–5 RIM consists of restriction of intramolecular rotation (RIR) (Fig. 1a) and restriction of intramolecular vibration (RIV) (Fig. 1b). This hypothesis is based on the fact that most ACQ-affected molecules have high coplanarity between aromatic rings in the molecule, but AIE molecules usually adapt a so-called propeller shape molecular structure. RIR states that in a propeller-shaped molecule, the “rotor” aromatic rings can rotate freely in the molecularly dissolved state, which creates a path for non-radiative decay. In this scenario, most energy is dissipated in the form of mechanical movement: in other words, intramolecular rotation. However, when aggregated, the intramolecular rotations are strictly restricted and thus mechanical energy dissipation is no longer a preferred way of relaxation. To compensate for the reduced intramolecular rotation energy relaxation, more exciton energy dissipates via the radiative pathway, with fluorescence emission. This results in the occurrence of AIE. RIV follows pretty much the same protocol as RIR.
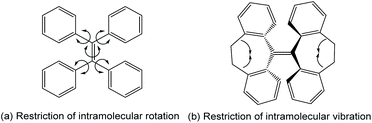 |
| Fig. 1 Restriction of intramolecular motions. Adapted from ref. 4. Copyright 2015 The Royal Society of Chemistry. | |
With the booming of AIE research in the past two decades, AIE-active macromolecule studies have seen growth as well, owing to the inherent advantages of macromolecules, like easier functionalization because different moieties in a macromolecule can cooperate for the same task,6,7 multi-functioning due to better capability of integrating more functional units8–11 and tuning of various properties,12–14 which are hard to achieve for AIE small molecules. Due to all these unique advantages, many reviews on AIE macromolecules have already appeared. Tang et al. provided a comprehensive and thorough review of AIE macromolecules in 2014 including their designs, structures, synthesis methods, and applications.6 Other excellent reviews about specific topics include AIE click polymerization,14 chiral AIE polymers,15 supramolecular AIE polymers,16 multi-component reactions for AIE polymer synthesis,17 AIE polymeric materials for bioimaging,7 and AIE materials for biomedical applications.18 Though all these reviews show undoubted academic excellence, most of them focus on specific types and applications of AIE-active macromolecules. We here report the most recent progress and development for AIE macromolecules, focusing on synthesis, structure, properties, applications, and the relationship among these factors. Through this review, we hope to provide a comprehensive, thorough vista about AIE macromolecules and serve as an encouragement and beacon for researchers interested in this field.
2. Design strategies, synthesis, and structures
During 20 years of AIE-research, many effective design strategies for AIE polymers have been developed by scientists around the world. Typical design and synthesis methods include polymerization of AIE-active monomers (Fig. 2a); side-chain functionalization with AIE functional groups (Fig. 2b); polymerization initiation with AIE-active initiators (Fig. 2c); polymerization of AIE-inactive monomers to achieve AIE-active polymers (Fig. 2d); copolymerization between AIE monomers and non-AIE monomers (Fig. 2e); attaching AIE luminogens (AIEgens) to polymerizable monomers followed by polymerization or copolymerization (Fig. 2f); etc. These typical methods can usually be utilized for synthesis of AIE macromolecules with different structures. For example, applying the method in Fig. 2c to an AIE-active initiator with multiple initiation sites can yield star-shaped AIE polymer. For further actualization of desired purposes, these strategies are sometimes combined to achieve synergetic performances of products.
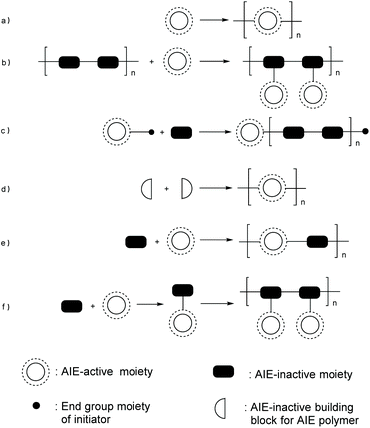 |
| Fig. 2 Synthetic strategies for AIE polymers. | |
Generally speaking, macromolecules can be classified by different structures that they adopt. Apart from the most commonly seen linear polymer structure, which most polymers adopt, some frequently seen polymer structures include hyperbranched structure,19–25 star-shape structure,26,27 and microporous structure.28–33 The AIE macromolecules that adopt these structures will have intrinsic advantages. For example, the microporous structure shows a prominent ability to adsorb small molecules like gas molecules and ions;10,34,35 star-shaped polymers have multiple functionalized chains connected to the central core thus higher density of functional units;26,36 and the synthesis of hyperbranched polymers is rather straightforward.21–23,25,37,38
2.1 Linear AIE polymers
Owing to the structural variety and good synthetic accessibility, linear AIE polymers always enjoy the most significant number of research focuses. By carefully choosing the monomers, electronic properties, absorption, and emission wavelengths, hydrophilicity and many other essential attributes can be fine-tuned to serve different purposes.
2.1.1 General AIE linear polymers.
Specific analyte detection with high sensitivity has always been a major task for chemosensors. So far, AIE-active polymers have found many applications in the sensing area with high sensitivity. A linear AIE-active polymer L1 which can be used as a Cu2+ fluorescent sensor (detailed mechanistic explanation in section 3.1) was synthesized by Wei et al. (Fig. 3 ).39 This linear polymer utilized the universal strategy in Fig. 2e, combining AIE-active tetraphenylethene (TPE) derivative with a sensor binding site, representing the most straightforward design strategy: using functional monomers directly as building blocks for AIE-active polymers. The synthesis of L1 was done by Sonogashira coupling with Pd catalysis, a common synthetic route with mild reaction conditions. To facilitate the dissolving of the polymer in organic solvents, n-octyl pendants were installed onto the TPE moieties since conjugated aromatic macromolecules usually show poor solubility in organic solvents.39 Moreover, hydrocarbon chains as side groups can also serve as spacers to reduce intermolecular interactions and thus reduce π–π stacking and lead to good AIE performance.
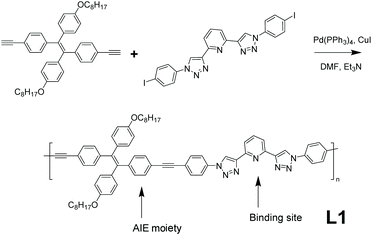 |
| Fig. 3 Synthetic route of polymer L1. Reprinted from ref. 39. Copyright 2020, with permission from Elsevier. | |
Safety concerns with explosives are a major issue around the world. The development of sensors to detect trace concentrations of explosive vapour has become a hot topic.40–43 Linear AIE polymer L2, which can serve as an explosive-detecting (detailed mechanistic explanation in section 3.1) sensor, was reported by Han et al. (Fig. 4a).44 Through anionic copolymerization, monomers 1,3-cyclohexadiene (1,3-CHD) and trans-stilbene (TS) were reacted together to give an alternating polymer. The alternating polymer then went through oxidative dehydrogenation by reacting with 2,3-dichloro-5,6-dicyano-1,4-benzoquinone (DDQ) to yield polymer L2. The strategy utilized here is from Fig. 2d, combining non-AIE monomers to build AIE-active polymers. Fig. 4b and c indicates that the fluorescence intensity of L2 rises with fw, showing AIE characteristics. AIE-active conjugated polymers like L2 are usually good AIE materials, but the syntheses of them normally require transition metal-based catalysed coupling reactions, like Sonogashira coupling for L1, Suzuki coupling, Wittig reaction, McMurry coupling, Hay–Glaser coupling, etc. The use of expensive metals and the non-recyclable nature of the catalysts inevitably result in the loss of high-cost metals. Syntheses like the one for L2 do not include precious metal catalysts, thus being a novel route worth investigating.
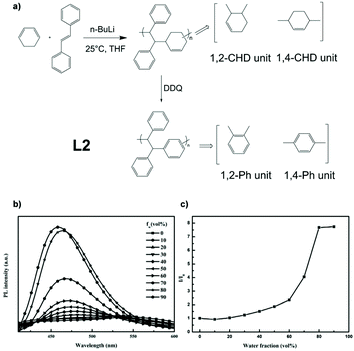 |
| Fig. 4 (a) Synthetic route of polymer L2. (b) Emission spectra of L2 in THF/water mixtures with different water fraction. (c) Plot of (I/I0) values versus the compositions of aqueous mixtures. I0 = emission intensity in pure THF solution. Reprinted from ref. 44. Copyright 2020, with permission from Elsevier. | |
One of the reasons that fluorescent materials have attracted lots of research attention is their ability to serve as bioimaging agents and thus provide a visual handle for clinical, surgical, and biomedical treatments (detailed discussion in section 3.3). Linear AIE polymer L3, which has the potential to be used for bioimaging, was reported by Wei et al. (Fig. 5).45 The synthesis of L3 consists of four steps: reaction between 4-(1,2,2-triphenylvinyl)benzaldehyde (TPB) and 4-methoxyphenylacetonitrile to give TPEOM; reaction between TPEOM and boron tribromide in dichloromethane to yield TPEOH; reaction between TPEOH and methacryloyl chloride in triethylamine to offer TPMA; and finally reaction between TPMA and poly(ethylene glycol) methacrylate (PEGMA), with azodiisobutyronitrile (AIBN) as radical initiator and chain transfer agent 4-cyano-4-(ethylthiocarbonothioylthio)pentanoic acid to give polymer L3, of which the synthetic strategy is shown in Fig. 2e. Due to the hydrophilic nature of PEGMA and the hydrophobic nature of TPMA, the polymer product becomes amphiphilic. The programming of PEGMA into the polymer chain not only endows the polymer with amphiphilicity which allows it to self-assemble into nanoparticles but also makes it biocompatible, and thus suitable for bioimaging applications.
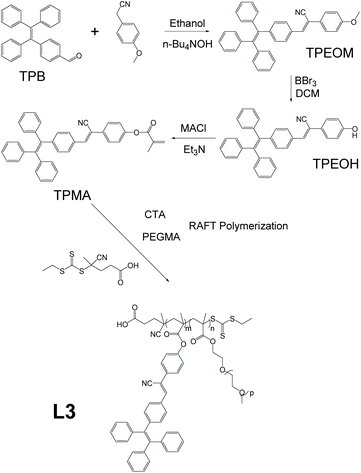 |
| Fig. 5 Synthetic route of polymer L3. | |
Linear polymer L4, which works as a dynamic tracking agent for the intracellular drug release process, was developed by Li and co-workers.8 The synthetic route of L4 is shown in Fig. 6, basically via Michael addition polymerization between 4-aminobutanic acid and TPE-based monomer, and then reaction with mannose-grafted side-chain polymer to produce polymer L4. The strategy used here should be classified as a variant of Fig. 2e, which copolymerizes AIE units with non-AIE units to obtain AIE polymer, and then further modification of the AIE polymer to obtain L4. With mannose moiety connected to the side chain as the identifier for mannose receptors, L4 forms nanoparticles that can go through mannose-mediated endocytosis to enter intracellular bacteria (ICB) infected phagocytes. Also, the TPE-containing backbone triggers the AIE effect when forming nanoparticles. When the L4 nanoparticles are loaded with deferoxamine-ciprofloxacin conjugates with Fe3+ (DFeC), the overlapping of emission from TPE moieties and the absorption of DFeC results in the FRET effect, and in turn, leads to quenching of fluorescence. The gradual recovery of fluorescence during the release process of DFeC can thus be utilized as the tracking agent of the drug releasing procedure.
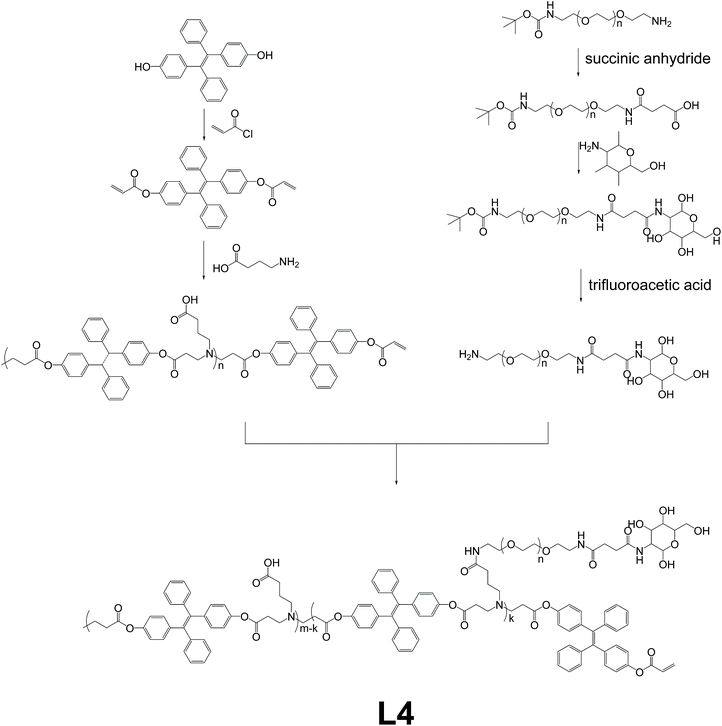 |
| Fig. 6 Synthetic route of polymer L4. Reprinted from ref. 8. Copyright 2020, with permission from Elsevier. | |
As the foundation of bone growth and a vital method for repairing damaged bones, osteogenic differentiation is a highly important process that requires in-depth clinical monitoring. However, most traditional monitoring methods for osteogenic differentiation include fixation or halting of osteocyte growth. To work around this problem, Zhang and co-workers reported a linear AIE-active polymer, L5.46 As demonstrated in Fig. 7, after several steps of preparation to obtain the monomer, which is a modified TPE unit, the monomers were polymerized together via Suzuki coupling, then underwent the final reaction to have four sodium carboxylate groups on every monomer unit, for which the synthetic strategy is same as that in Fig. 2e. With its conjugated backbone, L5 exhibits a maximum emission wavelength at 594 nm and maximum absorption at 429 nm (a large Stokes shift of 165 nm), proving L5 to be suitable for bioimaging applications. Due to the existence of sodium carboxylate groups, L5 shows good solubility in polar solvents and illuminates stronger with the addition of non-polar solvents such as THF. Further investigation illustrates that when incubated with a selection of different cells, it only shows strong fluorescence with specific differentiated cells, leading to the conclusion that L5 can be utilized as a bioimaging agent for osteogenic differentiation.
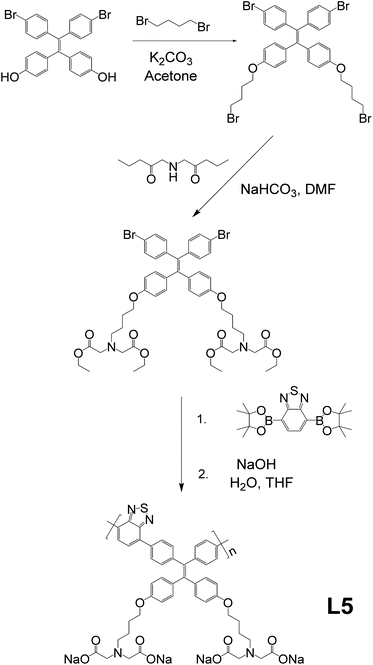 |
| Fig. 7 Synthetic route of L5. Reprinted from ref. 46. Copyright 2020, with permission from Elsevier. | |
Electrofluorochromism describes the fluorescence change of a material in response to an electrochemical redox applied to it.47 This special phenomenon is useful in various fields like display and sensing.48–51 AIE-active linear polymer L6, which exhibits outstanding electrofluorochromism and can be fabricated into porous polymer films, was developed by Chen and co-workers (Fig. 8).52 The polymer L6 itself is an alternating polymer with one AIEgen monomer and one AIE-inactive monomer, to build AIE-active macromolecules (Fig. 2e). To make it a porous polymer film, L6 was dissolved in electrolyte salt-containing dimethylacetamide solution, then solution-cast into films. Then the electrolyte salt grains were removed by immersing into acetonitrile to obtain porous L6 films. As for the molecular design aspects, the intended function of each part of L6 is demonstrated in Fig. 8b.
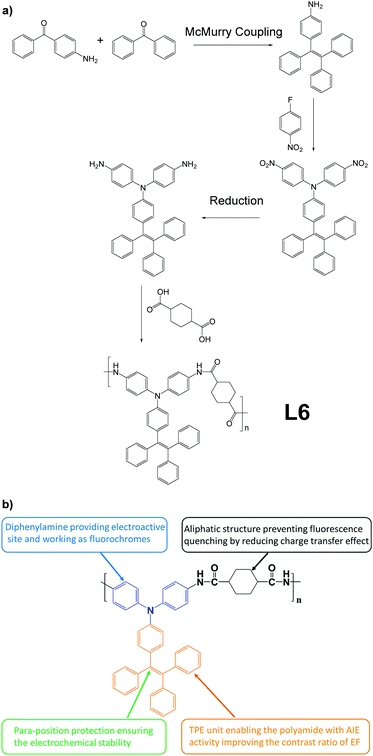 |
| Fig. 8 (a) Synthetic route of L6. (b) Explanation of function of different moieties in L6. Adapted with permission from ref. 52. Copyright 2018 American Chemical Society. | |
Thermoresponsive polymers are polymers that exhibit discontinuous, very large changes in properties in response to temperature change.53 Previous works showed thermoresponsive polymers to be good candidates for biomedical applications such as bioimaging and drug delivery.53–55 Being one of the major application directions of AIE polymers as well, biomedical application thus becomes an overlapping area for thermoresponsive polymers and AIE polymers, which means combining them would56 provide both with a wider range of functionalities. L7 is an AIE-active and thermoresponsive linear polymer, reported by Xu and co-workers, which can be utilized as a bioimaging agent (Fig. 9).57 It was synthesized through controllable reversible addition–fragmentation chain transfer (RAFT) polymerization between thermoresponsive units N-isopropylacrylamide (NIPAM) and AIE-active units 1-ethenyl-4-(1,2,2-triphenylethenyl)-benzene (TPEE), for which the synthetic strategy is shown in Fig. 2f. The resulting polymer shows very low photoluminescence (PL) intensity in pure THF but sees a very large PL intensity increase of about 34 times when the solvent system has fw = 90%, indicating excellent AIE characteristics. Due to the thermoresponsive nature brought by NIPAM, L7 has its micellar hydrodynamic diameter decreased by 20 nm (151 nm to 131 nm). Further investigation reveals that L7 also possesses great biocompatibility (for L7 concentration of 25 μg mL−1, more than 95% of cells incubated with L7 remain active after 24 h). The L7 taken up by a cell locates mainly in the cytoplasm in the vicinity of the nucleus. In addition, L7 exhibits excellent morphological and fluorescent stability. The particle size of L7 micelles does not see any obvious change after 1 month of storage, and PL intensity of L7 after 48 h of UV radiation sees no obvious change either.
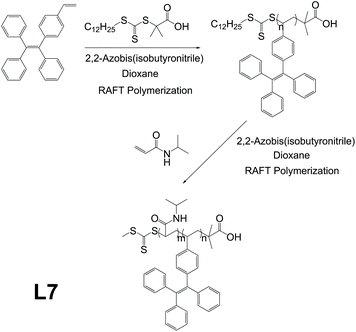 |
| Fig. 9 Synthetic route of L7. Reprinted from ref. 56. Copyright 2020, with permission from Elsevier. | |
Since AIEgens exhibit strong fluorescence in the aggregate state and good photostability, AIEgen-assisted confocal fluorescence microscopy is ideal for characterizing the microstructure of polymer nanocomposites.56,58 Cheng et al. reported the AIE-active linear polymer L8, which was synthesized through the AIEgen monomer 4-(1,2,2-triphenylvinyl)benzaldehyde (TPE-CHO) grafting to non-AIE-active polyvinyl alcohol (PVA) by aldolization (Fig. 10a and 2b).59 Furthermore, a PDMS-MMT-L8 nanocomposite composed of L8, MMT, and PDMS was prepared by the ice template method (Fig. 10b). In the composite, the AIE unit L8 can reconstruct the three-dimensional structure and be used for in situ crack tracing with the fluorescence images under a confocal fluorescence microscope.
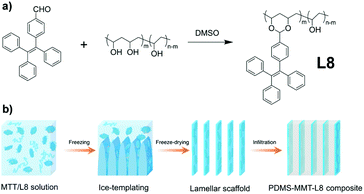 |
| Fig. 10 (a) Synthetic route of L8. (b) Fabrication process of the PDMS-MMT-L8 nanocomposite. | |
Sulfur is an abundant, cheap, stable, and readily available resource. However, the utilization of elemental sulfur to prepare sulfur-containing polymers is extremely limited, and therefore new sulfur conversion methods are of high importance.60,61 Tang et al. reported a linear polymer, L9, obtained by multi-component polymerization, which realized the simple one-step conversion of elemental sulfur into functional polymers.62 As shown in Fig. 11, L9, based on the design strategies for AIE polymers in Fig. 2e, was yielded from a cost-effective one-step process. The polymerization involves sulfur, diols, and diisocyanates under mild conditions (55 °C) and within a short period (1 h), using only base as a catalyst at high yields (up to 95%) to obtain L9 with large molecular weight (up to Mw of 53
100). When the TPE unit is introduced, L9 can be designed as a “turn-on” type fluorescent chemical sensor. Since L9 has a strong binding affinity to mercury ions, L9 could coordinate with mercury ions, and its polymer segments will aggregate with turning-on of fluorescence. The results show that L9 could achieve good selectivity, sensitivity, and “open” detection of mercury ions.
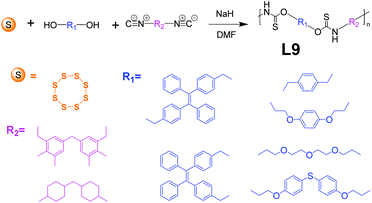 |
| Fig. 11 Synthetic route of L9. Adapted from ref. 62. Copyright 2021 American Chemical Society. | |
2.1.2 Yne polymerization.
Yne polymerization (triple bond polymerization), which is derived from the click polymerization developed by Sharpless et al.,63 sees great potential in syntheses of functional polymers due to many outstanding attributes, including mild reaction condition requirements, superb efficiencies, good selectivity, and simple product purification process.64–67 In recent years, yne polymerization has become very popular for AIE-active macromolecule synthesis. Many studies utilizing yne polymerization have been done by researchers, and three representatives are summarized here.
Click polymerization bears many inherent advantages such as mild reaction conditions, good yield, high efficiency, etc. Utilization of click polymerization in AIE polymer syntheses would provide AIE macromolecules with good prospects of industrialization and mass production. Linear polymers like L10a and L10b, finding application as explosive detectors, were synthesized and reported by Tang et al. (Fig. 12).68 The click polymerization that produces polymer L10a and L10b is a derivation of the click reaction that Sharpless and co-workers developed.63 The specific click polymerization that Tang and co-workers developed for polymers L10a and L10b, which was derived from Fig. 2e, was between ester-activated alkynes and aliphatic diols, in the presence of bicyclo[2.2.2]-1,4-diazaoctane (DABCO).68L10a and L10b both exhibit a prominent rise in PL intensities when fw increases and reach PL intensity peak at fw = 90%. Compared to their PL intensities in pure THF solution, when fw = 90%, L10a saw a 65-times increase, while L10b saw an increase by 125 times. This indicates their excellent AIE property. They show a drastic decrease in PL intensities upon mixing with explosive picric acid while maintaining the emission profile.
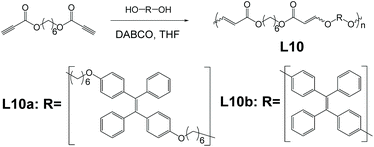 |
| Fig. 12 Synthetic route of polymer L10a and L10b. Adapted from ref. 68 with permission of The Royal Society of Chemistry, Copyright 2020. | |
Linear polymer L11, synthesized through facile, catalyst-free, and mild reactions, was developed and reported by Tang and co-workers.69 As illustrated in Fig. 13, the strategy to build AIE-active macromolecule L11 is essentially similar to that of L10a and L10b and belongs to the method of Fig. 2e, involving a straightforward one-pot click-polymerization synthesis from monomers, which only requires 60 °C reaction temperature and can be done in the open air. Upon reaction completion at 24 h, L11 polymers yielded from this reaction possess high molecular weights (highest Mw = 50
300), high yield (approx. 91%), and good stereoselectivity (Z-selectivity up to 84%). In addition, the films cast from L11 show low chromatic dispersions and good refractive indices. Moreover, the TPE moiety in the monomer can be swapped with other functional units (e.g., benzophenone moiety); thus, syntheses of non AIE-active analogies of L11 are also possible via this click polymerization method.
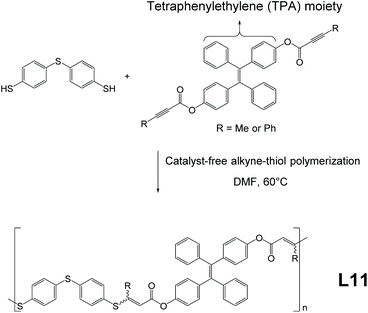 |
| Fig. 13 Catalyst-free alkyne-thiol synthetic route of L11. Adapted from ref. 69. Copyright 2020 American Chemical Society. | |
Linear AIE-active polymer L12, which is prepared by thiol–yne metal-free click polymerization, was reported by Tang et al.70 As demonstrated in Fig. 14, poly(vinylene sulfide) L12 is produced by click polymerization of its monomers with the help of K3PO4 at 100 °C. The synthesis protocol directs the reaction in a stereoselective, anti-Markovnikov way (Z-specific). As compared to L11, which is also a product from click polymerization, L12 exhibits better Z-selectivity (100% compared to 84% of L11) but comes at a price of slightly more demanding reaction conditions (higher reaction temperature and nitrogen atmosphere). Attribute-wise, the good thermal stability (decomposition temperature higher than 312 °C) and good refractive index (n > 1.628 for λ = 400 to 1700 nm) provide L12 with the possibility to be applied as a nanomaterial and organic photonic material.
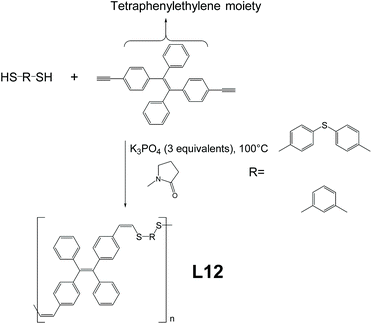 |
| Fig. 14 Thiolyne metal-free synthetic route of L12. Adapted from ref. 70 with permission from The Royal Society of Chemistry, Copyright 2019. | |
2.1.3 Linear AIE-active peptides.
Peptides, which consist of amino acids, are some of the most commonly seen biological polymers in the bodies of humans and other animals. For biological and medical applications such as biosensing, many AIE-active peptides were developed.71–73 The most significant advantage of AIE peptides is that the restriction of intramolecular motion of the AIEgen exerted by ordered self-assembly of peptides enhances the luminescence when AIE-active peptides encounter corresponding analytes.72,74–76
As an important exocrine enzyme in living organisms, alkaline phosphatase (ALP) plays a pivotal role in regulating various biological processes.77 Unusual levels of ALP could be a sign of various kinds of diseases, including cardiovascular disease, bone diseases, etc.78–82 However, most traditional ALP detecting methods involve cell fixation or result in irreversible nucleated crystallization. As such, conventional ALP detecting methods face big obstacles when applied to living cells. L13, which is an AIEgen-peptide conjugate utilizing in situ self-assembly-controlled fluorescence, was developed by Liu and co-workers (Fig. 15 ).76 This AIE peptide provides efficient living cell ALP tracking. In terms of synthesis, the peptide chain part was produced through solid-phase peptide synthesis. The chain end was then treated with 4-azidobutanoic acid, followed by functionalization with AIEgens. As shown in Fig. 15, the bulky side pendant hinders L13 from self-aggregating nicely before reacting with ALP. Thus, the unrestricted intramolecular motion of the AIE-active moieties inhibits the AIE effect. Upon contact with bacterial ALP, the bulky side pendant is turned into a small hydroxyl group, which would no longer hinder self-aggregation. Consequently, L13 forms aggregates that greatly cut down the intramolecular motion, and hence triggers the AIE effect with bright illumination. Further investigations show the PL intensity of L13 increased by 2.9 to 3.4 times after 2 h incubation with high ALP expressing bacteria and is highly selective as well, indicating that L13 is a good ALP sensor.
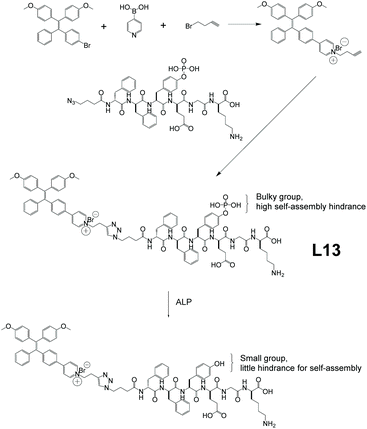 |
| Fig. 15 Synthetic route of L13, and its structural change when in contact with alkaline phosphatase (ALP). Adapted with permission from ref. 76. Copyright 2020 American Chemical Society. | |
2.1.4 Bacteria-templated polymerization.
In biological applications, specificity and selectivity towards targeted objectives can be pivotal. A synthetic strategy has been developed utilizing bacteria themselves as polymerization templates for AIE linear polymers to realize precise targeting. L14, a “turn-on” type self-selective bacteria-killing drug, was designed by Liu and co-workers (Fig. 16).83 The monomers for L14 went through atom transfer radical polymerization (ATRP), mediated by the ‘template’ bacterium to yield L14, such a strategy also belonging to the method of Fig. 2e. As a result, the L14 polymer chain only binds to the surface of its template bacterium, causing restriction of intramolecular motions, thus turning on the AIE fluorescence. Design-wise, the three monomers of L14 each have their specific allocated job. The red one serves as AIE moiety and phototherapy agent for photodynamic bacteria killing. The green one works as a solubility enhancer and a spacer between the other two monomers. The blue one is a permanent cation for binding onto negatively charged bacterial surfaces.
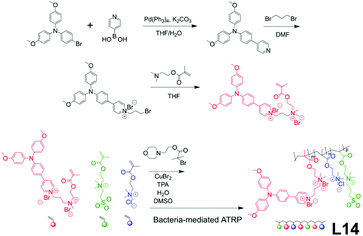 |
| Fig. 16 Synthetic route of L14. Adapted from ref. 83. Copyright 2015 Wiley-VCH. | |
2.1.5 Linear AIE polymers for gene delivery.
Gene delivery describes the process that introduces foreign genetic material (e.g., DNA, RNA) into targeted host cells. For a gene delivery to be successfully performed, the foreign genetic material must reach the nuclei while staying stable.84–88 To realize this goal, a suitable gene vector (the carrier of the foreign genetic material) must be chosen. Naturally, AIE materials with their capability of visualizing the gene delivery process are excellent potential candidates for this application.
For gene delivery, two main challenges are the escape of gene vectors (endo/lysosomal) and the consequent unpacking of nucleic acids in the cytosol. To tackle these problems, L15, a linear AIE polymeric gene vector, was designed by Liu et al.89Fig. 17 gives the brief synthetic route of L15. The TPE derivative, which makes L15 AIE-active, was synthesized to have reactive oxygen species (ROS)-cleavable aminoacrylate linkers at both ends, which subsequently was connected to oligoethylenimine of 800 Da (OEI800). OEI800 shows not only good biocompatibility but also good DNA binding ability, making it an ideal choice for DNA vector components. In addition, polyethylene glycol sidearms were added to adjust the water solubility of the resultant polymer. This strategy used here is shown in Fig. 2f. Like most other amphiphilic polymers, L15 is capable of self-assembly in water as well, and the electrostatic force between OEI800 moieties and DNA allows L15 to have DNA molecules trapped inside the nanoparticles of it nicely, thus serving as a gene vector (further details about the gene delivery application of L15 in section 3.4).
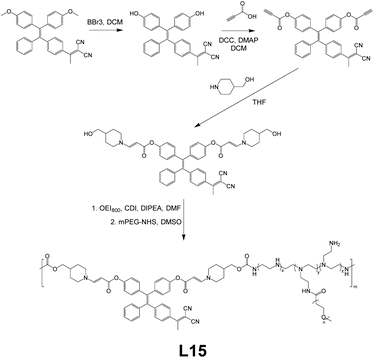 |
| Fig. 17 Synthetic route of L15. Adapted from ref. 89. Copyright 2015 Wiley-VCH. | |
2.2 Hyperbranched AIE macromolecules
Hyperbranched macromolecules are macromolecules with dendritic branches connected to a central core. Hyperbranched polymers are endowed with unique properties like high solubility, low viscosity, and low molecular entanglement with single-molecular dispersion in aqueous media with their unique architectural structures.19–21,23–25,90,91 Along with the discovery and development of AIE-active materials, numerous AIE hyperbranched polymers have also been developed. The two most commonly used synthetic strategies for hyperbranched macromolecules are convergent and divergent syntheses. For convergent synthesis, dendritic branches are synthesized first, followed by the connection onto a central core, while divergent synthesis grows the branches from the central core and makes them dendritic in consecutive steps. There are many hyperbranched AIE macromolecules that are synthesized via sophisticated methods, for instance, combinations of various synthesis strategies. This section summarizes the designs, structures, and syntheses of several recently announced, representative AIE-active hyperbranched polymers.
2.2.1 General hyperbranched AIE macromolecules.
Most hyperbranched AIE macromolecules adopt the classical hyperbranched structure, which consists of a central core and dendritic arms connected to it, and representative examples are presented in this section.
To detect pyrophosphate ions (PPi), which are vital in many cellular biochemical systems,75,92,93 hyperbranched polymer H1, which could serve as a “turn-on” type PPi detector, was reported by Wang et al. (Fig. 18).94 Polymer H1 was synthesized by first connecting TPE-epoxide to one of the monomers, tris(2-aminoethyl)amine (TAEA). The modified monomer (which is a part of the dendritic chain) was then reacted with the other monomer, N,N′-hexmethylenebisacrylamide (HMBA), and more TAEA to form the final polymer. In the final step, growth of the dendritic branches and connecting branches to the TAEA core were done simultaneously. Thus, the synthesis route is by a hybrid of the convergent and divergent methods, as shown in Fig. 2e. Also, further fine-tuning of the detecting performance of H1 can be achieved by altering the relative equivalents of TPE-epoxide, TAEA, and HMBA. The existence of both hydrophilic amine groups and hydrophobic TPE groups makes H1 an amphiphilic polymer, thus forming micelles in water solution easily, with AIE-active moieties as core and amine chains as shell. Owing to the hyperbranched structure of H1, when forming micelles in pure water, the outermost hydrophilic groups and the rather rigid dendritic backbone provide cavities for aromatic rings on the TPE moieties to rotate, hence increasing the non-radiative decay, and in turn, quenching the fluorescence even when aggregated. This special attribute allows H1 to be used as a “turn-on” type sensor rather than the normal “turn-off” type AIE-based sensors like L10a and L10b.
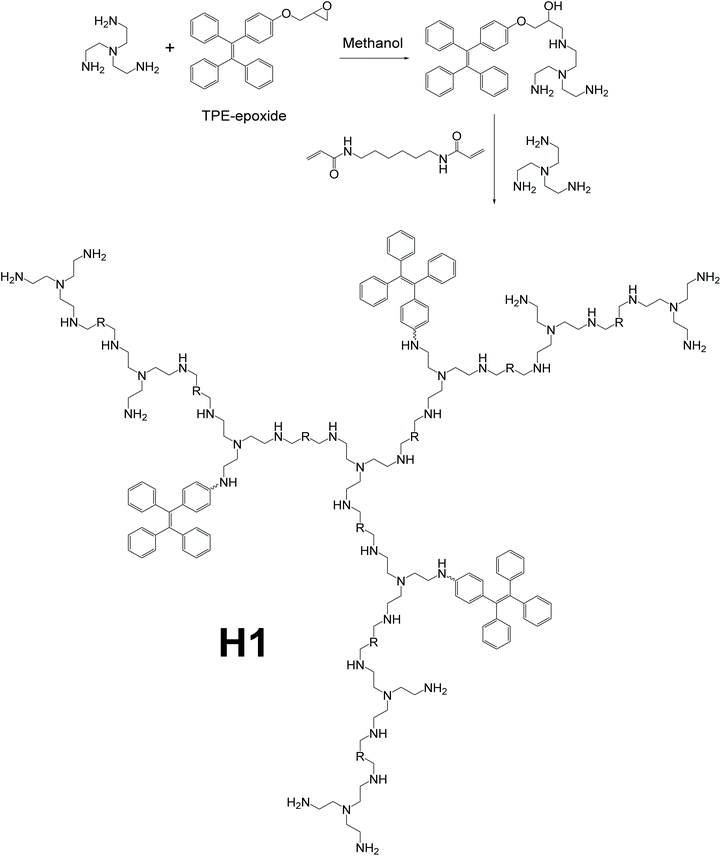 |
| Fig. 18 Synthetic route of polymer H1. Reprinted from ref. 94. Copyright 2019, with permission from Elsevier. | |
Stimulus-responsive materials refer to materials that have their physical/chemical properties altered in response to one or more types of stimuli, for instance, light, temperature change, mechanical force, chemical change, etc. (further details about the stimulus-responsive materials of AIE macromolecules in section 3.7).95,96 As its name implies, a multi-stimuli-responsive material is a material that can respond to multiple types of stimuli. Stimuli-responsive materials are widely used as smart materials in various applications like drug delivery, sensing, catalysis, and more.95–97 Hyperbranched polymer H2, which was developed to have a multi-stimuli-responsive fluorescence nature, was reported by Feng and co-workers (Fig. 19).37 Multiple available reaction sites on malic acid (MA) and triethanolamine (TEA) made the synthesis of hyperbranched polymer H2 fairly straightforward, being a simple one-pot condensation polymerization. Despite the easy synthesis, the structure and properties of H2 are still intriguing. Unlike most AIE-active molecules, H2 does not possess any rotatable aromatic rings. Although the synthetic strategies for H2 and L2 are the same, which is that illustrated in Fig. 2d, L2 has aromatic rotors on the polymer backbone while H2 not only uses non-AIE-active monomers but also has no aromatic ring rotor at all. Same as H1, H2 is also an amphiphilic polymer due to the existence of both hydrophilic groups (hydroxyls) and hydrophobic groups (esters). When aggregating in solution, H2 firstly forms self-assembled micelles with hydrophilic hydroxyl groups as shell and ester groups as core. Then, as the aggregation goes on, the micelles will aggregate to form supramolecular assemblies due to the interaction between hydroxyl groups on the micelle surface. This supramolecular assembling causes the overlapping of π electrons and lone pair electrons, which becomes a hindrance to non-radiative decay, resulting in the AIE effect (Fig. 19b–i).
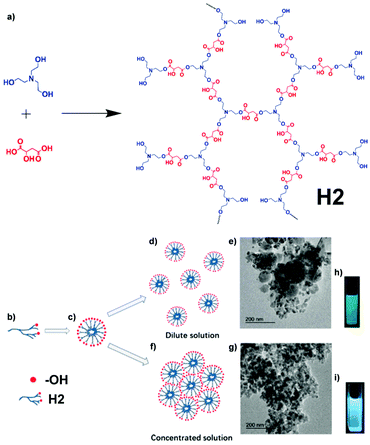 |
| Fig. 19 (a) Synthetic route of H2. (b) H2 with some unreacted hydroxyl groups. (c) The formation of HPAE supramolecular self-assemblies in aqueous solution. The aggregates of self-assemblies of H2 at different concentrations: (d) 2 mg mL−1 and (f) 60 mg mL−1. TEM micrographs of (e) 2 mg mL−1 and (g) 60 mg mL−1H2 solutions. Photographs of H2 solutions excited by UV light at (h) 2 mg mL−1 and (i) 60 mg mL−1. Reprinted from ref. 37. Copyright 2020, with permission from Elsevier. | |
Hyperbranched polymer H3, which was found to exhibit a hyperbranching-enhanced emission (HEE) effect, was reported by Zhang and co-workers (Fig. 20).38 Utilizing ATRP, H3 was synthesized from its monomers 4-(cyanomethyl)phenyl methacrylate (CPMA) and 2-((2-bromo-2-methylpropanoyl)oxy)ethyl methacrylate (BMPOEM). The synthetic strategy of H3 belongs to that shown Fig. 2e. BMPOEM serves as both initiator and branching agent, and the branching degree of the polymer produced can be controlled by varying the ratio of the two monomers. To prove H3 shows the HEE effect, several contrasting compounds (M1) and polymers (M2, M3) were made. It was found that M3 shows only weak emission and weak AIE effect; M1 shows strong emission but suffers from ACQ; although M2 is hyperbranched, it does not have AIE property and only emits weakly upon excitation. On the other hand, H3 emits strongly and shows the AIE effect. Based on these compounds’ rather closely related monomer structures, a conclusion was drawn that the HEE effect brings AIE to H3. The HEE effect was believed to emerge from the formation of nitrile clusters and improved through-space conjugation in the hyperbranched polymer due to the tight conformation and higher nitrile group density. H3 showed a PL intensity increase of 28 times from fw = 0% to fw = 90%, again proving its AIE characteristic.
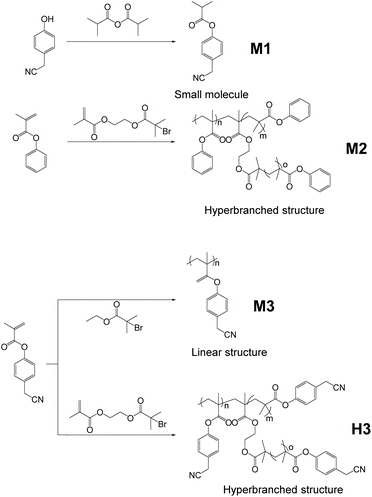 |
| Fig. 20 Synthetic route of H3, M1, M2 and M3. Adapted with permission from ref. 38. Copyright 2019 American Chemical Society. | |
2.2.2 Crosslinked AIE macromolecules.
Some hyperbranched macromolecules possess moieties capable of crosslinking with each other to form a very large web-like structure, and H4 is an example. As a common polymerization reaction, emulsion polymerization has found application in syntheses of various polymeric materials, including AIE polymers such as H4. H4 is designed to be a thermosensitive microgel, synthesized via emulsion polymerization and reported by Tang et al.98 As demonstrated in Fig. 21, H4 is composed of three components: 4-acylhydrazine-(2-hydroxy-3-(methacryloxypropyl))pyridine hydrochloride (AH monomer) as a comonomer; 1,1,2,2-tetrakis(4-methanoylphenyl)ethene (TPE-4ALD, M4) as AIE-active moiety; and NIPAM as the crosslinker due to its suitable lower critical solution temperature (the temperature below which the mixture has all its components miscible in all compositions). The three components were mixed in deionized water with acetic acid and 2,2′-azobis(2-methylpropionamidine) dihydrochloride (AIBA), then underwent surfactant-free emulsion polymerization to afford the crosslinked microgel H4.99–103 This strategy of preparing complex three-dimensional crosslinking system AIE macromolecules can be seen in Fig. 2e. One interesting property of H4 is cononsolvency, which means H4 can exhibit poor solubility when two of its good solvents are mixed at a certain ratio.104–106 Due to the micrometric, three-dimensional, flexible crosslinked structure of H4, it can respond to stimuli, like temperature changes, fairly quickly. With temperature rising, the maximum emission wavelength of H4 shows hypsochromic change with increased PL intensity. This is due to the shrinkage of crosslinked structures, limiting intramolecular motion, thus reducing energy dissipation via the non-radiative decay pathway.
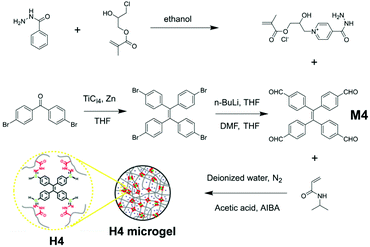 |
| Fig. 21 Synthetic route of H4. Adapted with permission from ref. 98. Copyright 2018 American Chemical Society. | |
2.3 Star-shaped AIE macromolecules
As the simplest branched polymers, star-shaped macromolecules share some similarities with hyperbranched polymers, like having a central core in their architectures and having arms reaching out from the central core; but the arms of star-shaped macromolecules are not dendritic, which is the fundamental difference between star-shaped macromolecules and hyperbranched macromolecules. Compared to general linear polymer structures, star-shaped polymers possess many inherent special properties such as high arm density (thus high functional group density), low internal viscosity, and low hydrodynamic radius.107–110 Taking advantage of these unique attributes, star-shaped polymers find application in drug delivery,111 thermoplastics,112etc. Combining with AIE features, these applications can be further enhanced (e.g., drug delivery agent with fluorescence bioimaging function), and other new applications can be realized.36,113
In sensing applications, usual fluorescent materials are designed to be either “turn-off” or “turn-on” types, but there are some exceptions. Star-shaped oligomer SS1, which serves as an “on–off–on” type of fluorescent sensor of Cu2+ and S2+, was synthesized and reported by Murugesapandian and co-workers (Fig. 22).113 Synthesis of SS1 can be done fairly facilely via Schiff base condensation reaction between triaminoguanidine hydrochloride and 4-diethylaminosalicylaldehyde. Although the structure of SS1 looks unremarkable at first glance, the mechanism called excited-state intramolecular proton transfer (ESIPT) is quite interesting to explore. ESIPT-active molecules usually have intramolecular hydrogen bonds between proton donors and proton acceptors. Upon excitation, proton transfer will happen between proton donors and acceptors, resulting in phototautomerization. In this architecture, three pairs of proton donors (OH) and acceptors (N
CH) are integrated, making it ESIPT-active. Therefore, the strategy for preparing SS1 should be classified as polymerization of AIE-inactive monomers to obtain AIE living polymers (Fig. 2d). Thanks to the ESIPT and AIE effects, SS1 is mechanoresponsive (more on this in section 3.7).
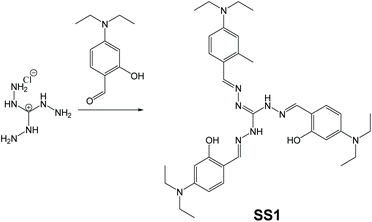 |
| Fig. 22 Synthetic route of SS1. Adapted from ref. 113. Copyright 2019 American Chemical Society. | |
Star-shaped polymer SS2, which is an AIE nanogel reported by Feng et al. (Fig. 23),36 has demonstrated a universal and highly efficient way to design an AIE-active nanogel. As shown in Fig. 23, the core and part of the star arms were synthesized via ATRP with monomer A, a hexa-functional initiator, N,N,N′,N′′,N′′′-pentamethyldiethylenetriamine (PMDETA) and copper bromide (CuBr), followed by another ATRP reaction to connect monomer B to each of the star arms (PMDETA and CuBr are used for the second ATRP reaction as well). Finally, the resultant star-shaped block copolymer underwent McMurry coupling with AIE-active groups to yield the AIE-active nanogel SS2. This strategy is a combination of those of Fig. 2b and c. The polymer was firstly made by initiation of initiator, then made into block copolymer with another monomer, and finally went through post-functionalization with AIEgen to achieve AIE-active star-shaped polymer. Working as spacers which were fixed onto polymeric networks, AIE-active moieties in SS2 have their intramolecular motions highly restricted even when dissolved in dilute solutions. This uncommon RIM in the non-aggregated state allows SS2 to emit nicely even when in dilute solution, unlike most other AIE macromolecules which have their fluorescence quenched in dilute solution.
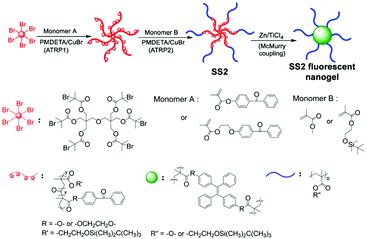 |
| Fig. 23 Synthetic route of SS2. Adapted from ref. 36. Copyright 2018 Multidisciplinary Digital Publishing Institute. | |
2.4 AIE-active supramolecular assemblies
Supramolecular assemblies are AIE-active macromolecules that possess complicated molecular assembly networks held together by non-covalent bonds and interactions, such as hydrogen bonds, π–π interactions, van der Waals forces, etc.114,115 The complex structures of supramolecular assemblies provide them with more possibilities of functionalities. With the advent of AIE, incorporating AIE moieties into supramolecular macromolecules has become a hot topic for researchers.
2.4.1 General supramolecular AIE macromolecules.
Supramolecular macromolecule SM1, which was designed to be an optical material, was developed by Lin and co-workers.116 From Fig. 24a, we can see that SM1 adopts a star-shaped structure and is obtained via the convergent synthetic route. The reason why it was categorized as supramolecular is visually illustrated in Fig. 24b. SM1 forms supramolecular assemblies which are held together by multiple hydrogen bonds (N
C⋯O
C and C
C–H⋯O
C). An interesting finding is that SM1 film shows dramatic transparency change when in contact with triethylamine vapour. In addition, this change can even be reversed by exposing the film to HCl vapour.
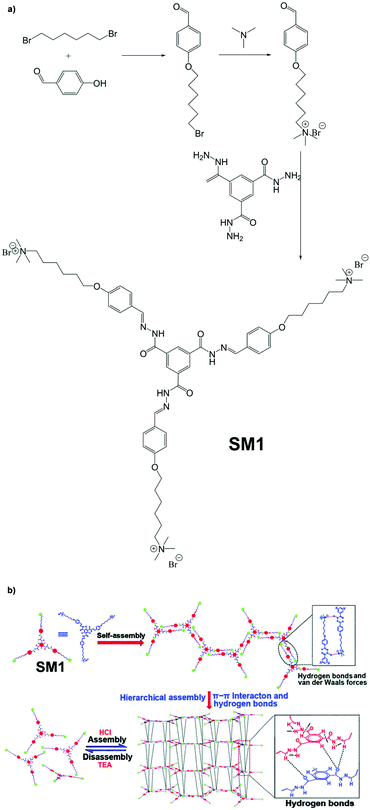 |
| Fig. 24 (a) Synthetic route of SM1. (b) Formation of supramolecular assemblies of SM1. Adapted from ref. 116 with permission of The Royal Society of Chemistry, Copyright 2020. | |
Incorporating AIE-active moieties into drug carriers will give a fluorescence imaging handle for the dynamic and precise tracking of the drug delivery process. Supramolecular macromolecule SM2 synthesized by the method in Fig. 2d, which was developed to be a drug carrier, was reported by Wang et al.9 As can be seen from Fig. 25, the core of SM2, which is a hyperbranched polymer of H-PAMAM with inherent AIE effect, was synthesized first via Michael addition and amination reaction. The hyperbranched polymer was then used as the core of the larger star-shaped polymer, and star arms were connected onto the core to form a big star-shaped polymer. SM2 was then combined with doxorubicin (DOX) and α-cyclodextrin (α-CD) to yield the drug-loaded carrier. The resulting drug-loaded carrier possesses not only AIE characteristics but also is capable of exhibiting the FRET effect between the drug DOX (acceptor) and SM2 (donor) as well.
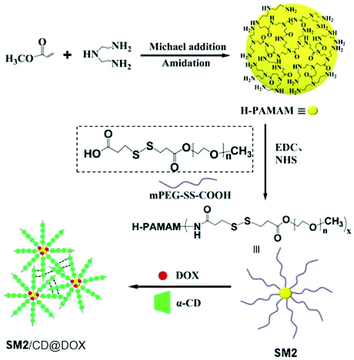 |
| Fig. 25 Synthetic route of SM2. Adapted with permission from ref. 9. Copyright 2019 American Chemical Society. | |
Supramolecular assembly SM3, which exhibited aggregation-induced emission enhancement (AIEE), was developed and reported by Liu and co-workers (Fig. 26).117 This giant assembly consists of 12 big antler-shaped ligands M4, four star-shaped ligands M5, and 48 Cd2+ ions. The syntheses of M4 and M5 are shown in Fig. 26a. This 64-component supramolecular metallo-cage is a gigantic assembly. Despite the sophisticated structure, supramolecular SM3 assemblies are still able to self-assemble with each other, forming even bigger assemblies. In this case, the AIE supramolecular SM3 is self-assembled from two different AIE-active monomers and can further self-assemble to form a larger AIE supramolecular aggregate, for which the synthetic strategy can be classified as a two-step polymerization of AIE-active compounds, as shown in Fig. 2a. According to tests carried out by Liu, the aggregation of SM3 assemblies resulted in the strengthening of emission intensity, which is the AIEE phenomenon (Fig. 26c).
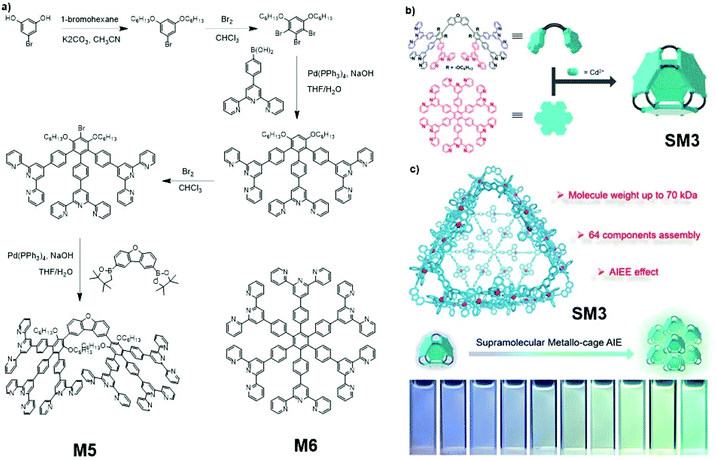 |
| Fig. 26 (a) Synthetic route of M5 and M6. (b) Formation of supramolecular assembly SM3. (c) AIEE effect of SM3. Adapted with permission from ref. 117. Copyright 2020 American Chemical Society. | |
Supramolecular assemblies SM4a and SM4b, which were designed to be artificial light-harvesting materials, were reported by Cao and co-workers (Fig. 27).118 Due to their ability to shift a bioimage channel from blue/green to red, SM4a and SM4b can be applied to improve the bioimaging signal-to-noise ratio. During the synthesis, the apex monomers work as the corners of four adjacent rectangular structures, and the connectors serve as the sides of the rectangular structures. Together, they form grid-sheet-like macromolecule sheets. The macromolecule sheets can then undergo self-assembly into supramolecular assemblies SM4a and SM4b, and they can adopt either AA-eclipsed or AB-staggered structures between different sheets, for which the synthetic route is shown in Fig. 2e. Designed to possess high porosity (more about porous AIE macromolecules in section 2.5), the cationic, hydrophobic cavities in SM4a and SM4b make them ideal matrices for dispersion of many organic dyes, and the supramolecular network skeleton provides reasonable gaps between organic dyes to prevent the ACQ effect. When the cavities are occupied by organic dyes such as Nile red, the FRET effect will happen between the supramolecular assembly network (donor) and the organic dye (acceptor), leading to a much stronger emission of the organic dye.
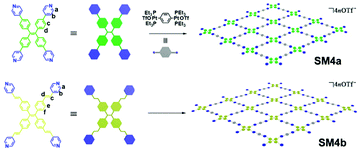 |
| Fig. 27 Synthetic route of SM4a and SM4b. Adapted with permission from ref. 118. Copyright 2020 American Chemical Society. | |
Exosomes refer to nanoscale extracellular vesicles, which are closely involved with signal transduction and material transport between cells.88 The fusion and fission behavior of exosomes is very important to communication among cells.119 To explore the membrane behavior of exosome fusion and fission and its regulatory factors, Wang et al. took advantage of the reversibility of redox reactions and prepared a kind of AIE supramolecular vesicle, SM5, based on Fe2+ coordination.120SM5 is self-assembled by AIE-active M7, cetyltrimethylammonium bromide (CTAB), and Fe2+ (Fig. 28). A negatively charged four-arm molecule, M7, can combine with 8 positively charged CTABs through ionic interaction and self-assemble to form a neutral fluorescent vesicle, SM5. After SM5 is formed, there are still coordination units on M7 so that SM5 can coordinate with various metal ions (such as Fe2+, Fe3+). When Fe2+ is oxidized to Fe3+, the hydrolysis of Fe3+ reduces the coordination ability of the vesicles, resulting in that the vesicles tend to fuse and form large-sized vesicles. On the contrary, when Fe3+ is reduced to Fe2+, the vesicles tend to fission and form small vesicles (Fig. 28b). Meanwhile, one can use the AIE characteristics of SM5 to monitor the accumulation of molecules through fluorescence emission in the process of vesicle transformation, thereby providing an innovative understanding of the fusion and fission behavior of exosomes. Moreover, SM5 can be used as a nanocarrier to load siRNA and transfer siRNA to cancer cells through a fusion process.
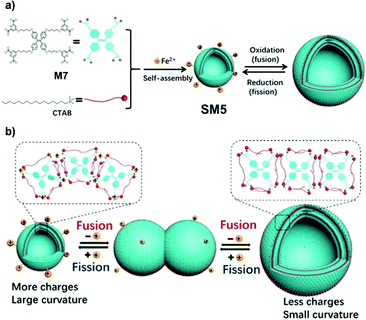 |
| Fig. 28 (a) Schematic illustration of construction of SM5, and its reversible and controllable fusion-fission behaviours. (b) Schematic illustration of possible mechanism of reversible and controllable fusion and fission behaviours. Adapted from ref. 120. Copyright 2020 Wiley-VCH. | |
2.4.2 Supramolecular AIE-active peptides.
Being biological polymers themselves, peptides enjoy excellent biocompatibilities compared to other polymers. Thus in biological/medical applications, research has been done to combine the advantages of the AIE effect and peptides together for the greater good.72–76
SM6, composed of M8 and M9, supramolecular assemblies that exhibit tumor micro-environment responsiveness and can provide image guidance for cancer therapy, was reported by Ding and co-workers.11 As shown in Fig. 29a, M8 consists of a unit of α-cyclodextrin, a peptide chain, and an AIE-active moiety. To make the supramolecular assembly multi-functional, M9 with AIE moiety substituted by gemcitabine (GEM) was also synthesized. These serve as a cancer-treating drug. M8 and M9 chains will then aggregate together with a modified polyethylene glycol (PEG) chain via host–guest interaction to form supramolecular assembly SM6. The synthesis method for AIE-active macromolecule SM6 belongs to that shown in Fig. 2e. This specific structural design has an outer shell of modified PEGs which can be cleaved by matrix metalloproteinase-2 (MMP-2) to form a positively charged outer layer. The positive charges on the outer layer of the assembly can facilitate endocytosis into cancerous cells due to the negative charges on the cell membrane of cancerous cells. Upon being taken up by the targeted cells, the high intracellular concentration of glutathione (GSH) would cleave between GEM and the rest of the molecule, thus releasing GEM for cancer treatment objectives (Fig. 29b). Meanwhile, the AIE-active molecule SM8 with FR/NIR fluorescence could also serve a function for drug tracking and diagnosis of pancreatic cancer.
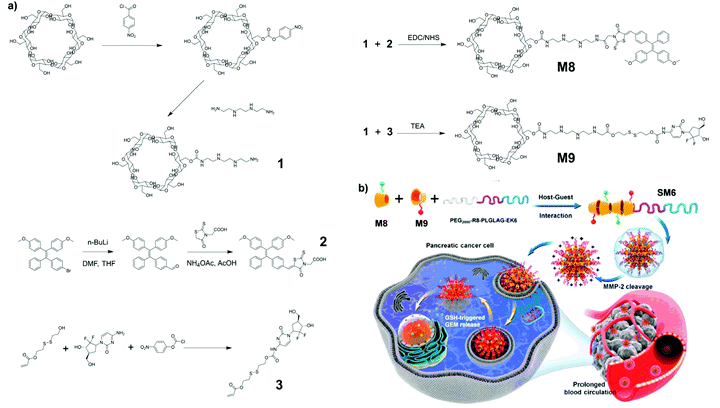 |
| Fig. 29 (a) Synthetic route and (b) formation of supramolecular assembly of SM6. Adapted with permission from ref. 11. Copyright 2020 American Chemical Society. | |
2.5 Microporous AIE macromolecules
Microporous macromolecules are macromolecules with pores of diameters below 2 nm.121 Microporous macromolecules can be further divided into categories, including covalent organic frameworks (COFs), metal organic frameworks (MOFs), coordination polymers, macro-cages, etc. Owing to their rigid three-dimensional structures and high porosity, microporous macromolecules usually have attributes like low density, high surface area, stable chemical properties, etc.28–33 These attributes enable porous macromolecules to find applications in areas such as gas adsorption,34 film fabrication,122etc. With these applications, combining AIE-active moieties with porous macromolecules for win-win outcomes (e.g., analyte adsorption with concurrent AIE fluorescence sensing) becomes a hot topic for researchers worldwide.
2.5.1 COF AIE macromolecules.
COFs are two-dimensional and three-dimensional covalent materials with their structural components held together by covalent bonds.123 Good examples of COFs are diamond and graphite, which clearly exemplify one of the outstanding characteristics of a microporous material, stable chemical property. Getting AIEgens into such frameworks will endow COFs with AIE, which can further broaden the field of application of COFs.
AIE-active COF CO1, which is capable of concurrent detection and adsorption of Ru3+, was reported by Yan and co-workers (Fig. 30).35CO1 consists of AIEgen (2,6-bis(4-(diphenylamino)styryl)-1-methylpyridin-4(1H)-ylidene) malononitrile (M10) as interconnecting agent and octavinylsilsesquioxane (OVS) which has multiple sidearms available for connection. The two monomers underwent Friedel–Crafts reaction, as shown in Fig. 2e, to yield CO1. Despite still being AIE-active, CO1 showed much weaker AIE effect compared to its monomer TPA-TCMP due to the structural separation between adjacent AIEgens, which does not allow them to aggregate as closely as they can in the monomer form. However, CO1 does provide more stable emission signal output as compared to its AIE-active monomer.
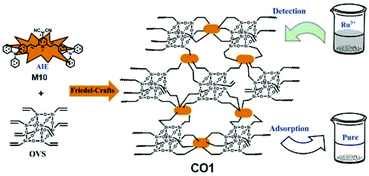 |
| Fig. 30 Synthetic route of CO1. Reprinted from ref. 35. Copyright 2020, with permission from Elsevier. | |
A high signal-to-noise ratio is always one of the ultimate goals, which researchers keep in mind when designing detectors. AIE-active COFs CO2, CO3, and CO4, which can be used carry out ultrasensitive amino acid detection, were prepared and reported by Zhao and co-workers.124 As shown in Fig. 31, CO2–4 involves a similar synthesis scheme to that of SM4a and SM4b (Fig. 2e). M11, the AIEgen involved in the syntheses of CO2-4, can be facilely synthesized from TPE bromination followed by Suzuki cross-coupling, as illustrated in Fig. 31a. M11 was then dissolved in 1,4-dioxane, sonicated, and mixed with aqueous acetic acid and chosen interlinking small molecules (hydrazine, para-phenyldiamine, or a mix of both). The reaction vessel was then flash-cooled with liquid N2, allowed to warm to room temperature and heated to 120 °C, and reacted for 5 days. The resulting COF CO2-4 adopts layered structures which can stack together to form supramolecular assemblies. In CO2-4, the ultrathin two-dimensional sheet structure provides a better pathway for energy migration, thus allowing it to perform turn-off type biomolecular recognition with high analyte (amino acids) sensitivities. Also, the amino acid binding affinities (expressed as the fluorescence quenching constant) of CO2, CO3, and CO4 are in the order CO2 < CO3 < CO4, which is in line with their number of azine moieties, e.g., the more azine units in the structure, the higher the affinity towards amino acids. Further tests verified that CO2 is also capable of detecting L-dopa (a drug for Parkinson's disease) in a “turn-off” manner as well.
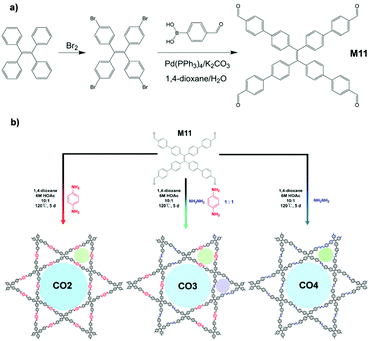 |
| Fig. 31 (a) Synthetic route of M11 (AIEgen in CO2-4). (b) Synthetic approach for the formation of the 2D COFs (CO2-4) and COF model compound. Adapted from ref. 124. Copyright 2019 American Chemical Society. | |
2.5.2 MOF AIE macromolecules.
MOFs are materials of one-, two- or three-dimensional structures formed by metal ions or clusters with organic ligands coordinated to metal ions/clusters.28,125 Owing to their excellent surface area, adjustable porosity, and highly programmable structures, MOFs have found applications in many areas like sensing, gas adsorption, catalysis, etc.125–128 In the past few years, various AIE-active MOFs have been reported, which possess novel properties and structures, and we select several examples in the following.
AIE-active MOF MO1, which finds application as an aqueous Cr2O72− detecting and removing agent, was reported by Liu et al.10 (Fig. 32). This MOF structure consists of Zr clusters (each Zr cluster has chemical formula Zr6O4(OH)8(H2O)4(COO)8) and 2,3,5,6-tetrakis(4-carboxyphenyl)pyrazine (M12). To produce MO1, M12 was first synthesized via the synthetic route in Fig. 32a. Upon obtaining M12, it was dissolved together with ZrCl4 in dimethylformamide (DMF), followed by the addition of formic acid. The mixture was then heated to 120 °C for 72 h to obtain MO1. The strategy here belongs to that in Fig. 2e. Due to the use of AIE-active TPE derivative M12 (solid-state quantum yield 5.08%) as the building block, MO1 shows a quantum yield of 7.02% in solid state. Under 385 nm excitation radiation, MO1 has its emission peak situated at 450 nm. The extensive porous MOF in MO1 allows it to perform good adsorption of Cr2O72− and the large surface area subsequently facilitates the photocatalytic reduction of Cr(VI) to Cr(III).
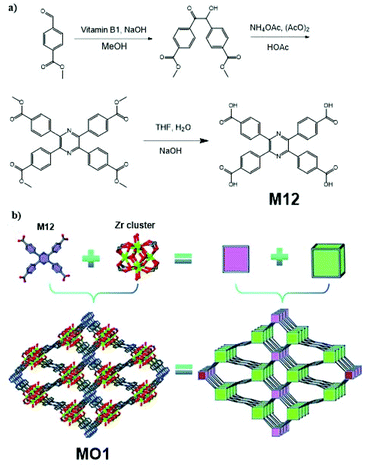 |
| Fig. 32 (a) Synthetic route of M12. (b) Topology simplification of MO1 involving ball-and-stick view and polyhedron view (H atoms are omitted for clarity and the colour code is Zr, green; C, grey; O, red; and N, blue). Adapted from ref. 10. Copyright 2013 The Royal Society of Chemistry. | |
AIE-active MOF MO2, which can serve as a biosensing agent for carcinoembryonic antigen, was reported by Xiao and co-workers.129 The synthetic strategy taken for MO2 is similar to the one for MO1. As can be seen from Fig. 33, AIE-active TPE derivative M13 was heated together with hafnium tetrachloride (HfCl4) in DMF to yield the MOF MO2. Despite the simple synthesis, MO2 can be made into ultrathin two-dimensional (2D) sheets, exhibiting excellent electochemiluminescence (ECL) property. Compared to M13 and 3D aggregates of MO2, 2D sheets of MO2 performed much better in ECL. The mechanisms resulting in this phenomenon are: immobilization of M13 moieties in the framework hinders the non-radiative energy dissipation and increases its special packing density; similar to CO2-4, the porous 2D structure provides a shorter diffusion path for species that would lead to ECL, and the electrochemical excitation of the AIE-active M13 moieties is made easier. With its highly sensitive ECL characteristics, 2D thin layers of MO2 can be utilized in carcinoembryonic antigen detector fabrication.
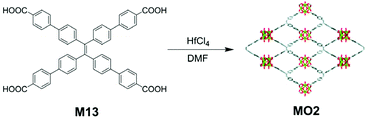 |
| Fig. 33 Synthetic route of MO2. Adapted from ref. 129. Copyright 2009 The Royal Society of Chemistry. | |
AIE-active MOF MO3, which exhibits dual mechano-switchable circularly polarized luminescence (CPL), was reported by Liu et al.130 (Fig. 34). CPL refers to an electromagnetic wave which generates an electric field of constant magnitude but rotates at a constant angular velocity in a plane perpendicular to the propagation direction of the wave.131–133 CPL can be further divided into right-CPL and left-CPL, in which left and right refer to the rotation manner of the electric field, right-handed or left-handed. Traditional syntheses of CPL materials usually involve lengthy and complicated steps. To work around these, chiral reticular self-assembly was employed for the synthesis of MO3. Briefly, tetrakis(4-pyridylphenyl)ethylene (M14, AIEgen in MO3), camphoric acid (CAM), and Cd(NO3)2C4H2O were dissolved in the solvent system (DMF + H2O + ethanol). The mixture was then heated to 95 °C and allowed to react for 24 h. Similar to the synthesis method in Fig. 2e, the synthesis of MO3 used an AIE-active unit and two non-AIE-active units to build AIE-active macromolecules. As a result, MO3 was obtained as an optically pure MOF, which was claimed as the first-ever optically pure AIEgen MOF that is capable of exhibiting dual mechano-switchable circular dichroism (CD), fluorescence, and CPL. Upon forming the MOF, frame-enabled emission was triggered and chiral functionality via through-space chirality transfer was realized. According to the use of different CAM (D-CAM or L-CAM) in the synthesis, the obtained MO3 will show the same chirality as the CAM used. In the D-CAM case, Cd(II) polyhedrons, D-CAM, and TPyPE will all arrange in a right-handed manner, while in the L-CAM case, all will arrange left-handedly.
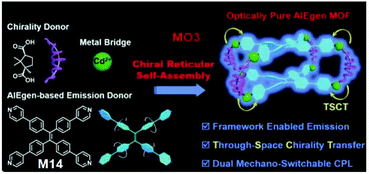 |
| Fig. 34 Structure of MO3 and its chiral reticular self-assembly synthetic strategy. Adapted from ref. 130. Copyright 2020 Wiley-VCH. | |
For effective photodynamic therapy (PDT), both precise activation of photosensitizers (PSs) in the targeted lesion and minimization of ROS loss by endogenous antioxidants in micro-environments of disease tissues are needed. To achieve these goals without complicated molecular design and modification of PSs, general tumor-responsive PDT platforms are urgently needed. MO4, a MOF material developed by Liu et al.,134 could effectively deliver molecules into tumors and further can be decomposed in a tumoral acidic environment with release of the encapsulated PS and activation of ROS generation. MO4 is composed of the MOF material MOF-199, which has been well documented,135–137 and an AIE-active PS, M15. The PS M15 is synthesized via the route demonstrated in Fig. 35a, and it is physically adsorbed onto MOF-199 to form MO4. MOF-199 protects the PS loaded onto it from reacting with endogenous antioxidants preventing them from generating ROS. MOF-199 is then coated by F-127 to yield MO4 nanoparticles (Fig. 35b). The enhanced permeation and retention (EPR) effect results in the accumulation of MO4 in cancerous cells. Consecutively, inside cancerous cells, Cu2+ in MO4 will react with glutathione, a typical endogenous antioxidant, thus resulting in the breaking down of MO4 and releasing PSs from MO4. At this stage, the ROS generation from PS is no longer prohibited by MO4, and PDT can be activated (Fig. 35b). Importantly, the scavenging of glutathione by Cu2+ greatly cuts down ROS loss through reaction with antioxidants, largely increasing the PDT efficiency. Moreover, the AIE nature of the PS means that the high fluorescence signals can serve as effective guidance for the PDT.
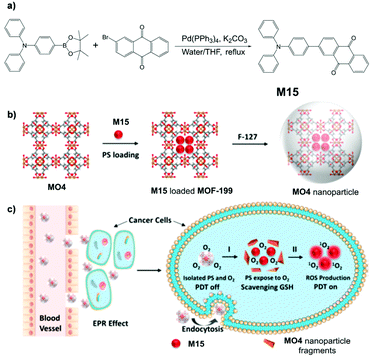 |
| Fig. 35 (a) Synthetic route of M15. (b) Synthetic route to MO4 and F127-coated MO4 nanoparticles. (c) Quench and trigger of photosensitization originated from MO4 NPs in a tumor microenvironment. Process I: MOF-199 reacts with GSH to collapse the framework. Process II: light illumination to produce ROS. Adapted with permission from ref. 134. Copyright 2019 American Chemical Society. | |
3. Applications
Since discovering AIE in 2001, two decades of enthusiastic AIE-related research have brought numerous successes to AIE material applications. For AIE-active macromolecules, the combination of properties of small-molecule AIEgens and advantages of polymers amplifies the strengths of both, endowing AIE macromolecules with superior performances compared to traditional materials. For instance, the conventional fluorescent chromophores in bioimaging applications usually suffer from ACQ, which severely hinders their effective application. By utilizing photostable AIE macromolecules as bioimaging agents, the ACQ and photobleaching problems related to ACQ can be eliminated in one go.45,46,138 Moreover, the realization of more sophisticated designed macromolecule structures for AIE materials has escalated the boundaries of functionalization and application to another new level. In this section, we will discuss the applications (and potential applications) of several recently developed AIE macromolecule materials. Note that the applications we summarize here are just carefully chosen examples and do not represent all possible applications.
3.1 Sensors
The same as many light-emitting materials, AIE materials find fluorescent sensing as one of their major applications. With well-designed polymer structures and various functional moieties, AIE macromolecules can exhibit high sensitivity and fast and strong response signal because of their inherited AIE nature.
Ion detecting fluorescent sensors such as L1 are some of the major AIE sensors under exploration.39 Designed with a built-in binding site (Fig. 2) on the polymer backbone, L1 serves as a good Cu2+ fluorescent sensor. As a “turn-off” type sensor for Cu2+, upon detecting Cu2+, L1 shows an obvious PL intensity drop (Fig. 36a and b). The weakening of PL intensity is believed to be caused by the ligand-to-metal charge transfer-based heavy metal ions effect. However, the intriguing part about fluorescent sensor L1 is yet to be discussed. After forming a complex with a large amount (∼30 equivalents) of Cu2+, the fluorescence of L1 was heavily quenched. But if histidine was added, the fluorescence of the resultant mixture will gradually increase, making L1 an “on–off–on” fluorescent sensor. As can be seen from Fig. 36c and d, upon the addition of histidine, the fluorescence intensity goes up. The L1–Cu2+ complex was also proven to be highly selective towards histidine, and the addition of other amino acids will not trigger the fluorescence. In addition to the “on–off–on” behavior, L1 also shows the CD property. P1 is another metal ion-detecting fluorescent “turn-off” type sensor designed to not only detect but also adsorb Ru3+.35SS1 is an “on–off–on” type fluorescent sensor, designed to work with Cu2+ and S2−.113Fig. 37 visualizes the PL intensity change of SS1 with concentration change of Cu2+ and S2−. Quenching of SS1 fluorescence is highly selective towards Cu2+, and the Cu2+-quenched fluorescence of SS1 can be restored by the addition of S2− and S2− only. Based on the highly selective and reversible sensing of SS1, the designer of SS1, Murugesapandian and co-workers, then tested whether SS1 can be used as an implication logic gate,113 and the results were satisfactory. This means SS1 can be potentially applied as a molecular switch. In addition to all these, SS1 is also a mechanoresponsive AIEgen, which means its emission can be triggered by mechanical forces (more discussion about stimuli-responsive AIE macromolecules in section 3.7).
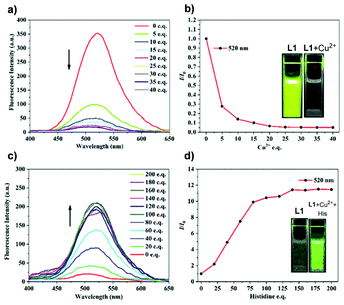 |
| Fig. 36 (a) Fluorescence spectral changes of L1 (1.0 × 10−5 mol−1L1 in THF/H2O mixtures, fw = 90%, λex = 360 nm) upon the addition of increasing amounts of Cu2+. (b) Plot of fluorescence quenching efficiencies (I/I0) versus the concentration of Cu2+ at 520 nm. I0 = fluorescent intensity of L1 in the absence of Cu2+. Inset: visible fluorescence of the L1 solution before (left) and after (right) the addition of 30 equivalents of Cu2+ under 365 nm UV lamp. (c) Fluorescence spectral changes of L1 with 30 equivalents Cu2+ (1.0 × 10−5 mol−1L1 in THF/H2O mixtures, fw = 90%, λex = 360 nm) upon the addition of increasing amounts of histidine. (d) Plot of fluorescence enhancement (I/I0) versus the concentration of histidine at 520 nm. I0 = fluorescence intensity of L1 with 30 equivalents Cu2+ in the absence of histidine. Inset: visible fluorescence of the L1 solution with 30 equivalents Cu2+ before (left) and after (right) the addition of 200 equivalents of histidine under 365 nm UV lamp. Reprinted from ref. 39. Copyright 2020, with permission from Elsevier. | |
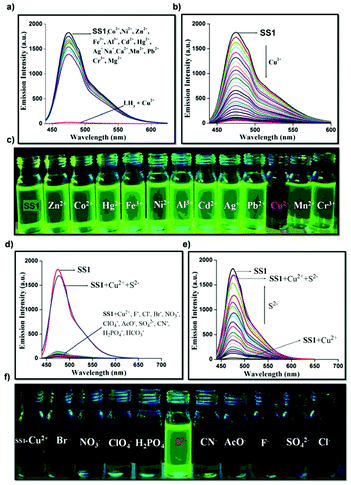 |
| Fig. 37 (a) Fluorescence spectra of SS1 (CH3CN/H2O; 9 : 1, v/v; Tris-HCl, pH = 7.2) with various metal ions (∼5 equivalents) (λex = 430 nm). (b) Fluorescence spectra of LH3 (CH3CN/H2O; 9 : 1, v/v; 10 mM Tris-HCl, pH = 7.2) with increasing concentration of Cu2+ (λex = 430 nm). (c) Photograph of SS1 with various metal ions under UV light at 365 nm. (d) Emission changes of SS1 with different anions (5 equivalents) in the presence of Cu2+. (e) Emission titration of SS1-Cu2+ with S2− anion. (f) Colorimetric comparison of S2− with SS1-Cu2+. Adapted with permission from ref. 113. Copyright 2019 American Chemical Society. | |
Since 2009, explosives sensing has become a hot topic among AIE researchers,40–43 due to safety concerns around the world.40L2 is one of the results of the scientific pursuit for AIE-active explosive sensing.44 The same as L1, L2 is also a “turn-off” type sensor. Designed to detect trinitrotoluene (TNT), L2 solution shows a sharp cut in PL intensity when TNT is detected (Fig. 38). Even a concentration of TNT as low as 1.7 × 10−4 M can lead to a great drop in PL intensity for L2 solution (2.5 × 10−5 g mL−1), showing its high sensitivity. At 2.9 mM, the PL intensity of L2 solution was reduced by 80%. L10a and L10b are also explosive detectors, which can detect explosive compound picric acid (PA).68 As can be seen from Fig. 39, L10a and L10b are both “turn-off” type sensors, showing a decrease in PL intensity when the concentration of PA in the solution is increased.
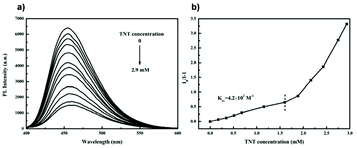 |
| Fig. 38 (a) Emission spectra of L2 in THF/90 vol% water containing different concentrations of TNT. (b) Plot of (I0/I − 1) values versus TNT concentration (I = PL intensity, I0 = intensity at [TNT] = 0 mM). Reprinted from ref. 44. Copyright 2020, with permission from Elsevier. | |
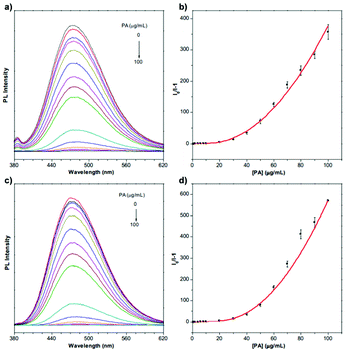 |
| Fig. 39 PL spectra of L10a (a, b) and L10b (c, d) in THF/water mixtures (fw = 90%) containing different amounts of picric acid (PA). Concentration: 10 μM; excitation wavelength: 319 nm for L10a and 314 nm for L10b. Adapted from ref. 68. Copyright 2020 The Royal Society of Chemistry. | |
Sensing specific biochemical identities is of great importance when monitoring and tracking biochemical processes. Among common biochemical materials, pyrophosphate is a vital one owing to its roles in many cellular biochemical systems.75,92,93 Hyperbranched macromolecule H1 is a metal-free,94 organic solvent-free, “turn-on” type sensor designed to detect PPi. The terminal NH2 groups render H1 water-soluble for more convenient PPi detection. Fig. 40 shows that in a neutral solution, H1 works as a proper “turn-on” sensor for PPi. In an acidic environment, the addition of PPi only results in slight emission enhancement, whereas, under basic conditions, H1 has its emission turned on even without the presence of PPi.
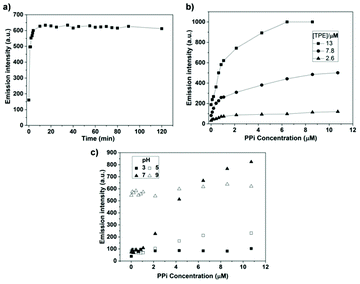 |
| Fig. 40 (a) Time-dependent emission intensity at 475 nm of H1 with the addition of 4.2 μM of PPi (H1 = 13 μM, pH = 7.4). (b) Emission intensity at 475 nm of H1versus PPi concentration at different TPE concentration (pH = 7.4). (c) Emission intensity at 475 nm of H1versus PPi concentration at different pH condition (H1 = 13 μM; λex = 360 nm; slit widths of excitation and emission are 10 and 10 nm, respectively). Reprinted from ref. 94. Copyright 2019, with permission from Elsevier. | |
For some heavy metal ions, removal of the ions is as important as sensing them to prevent further environmental contamination.35,139 Boasting a large surface area, porous structure, stable chemical and physical properties, porous polymers are capable of not only working as fluorescent sensors but also as adsorbing agents for heavy metal ions. Accomplishing multiple tasks with the same material has always been a pursuit of high priority when designing new materials, and AIE materials are no exception to this. CO1 is a representative AIE macromolecule that can perform concurrent “turn-off” type detection and adsorption of Ru3+.35Fig. 41a–d illustrates that with an increase of Ru3+ concentration, PL intensity of CO1 shows a drastic drop, indicating its capability to act as a sensitive “turn-off” sensor. Also, various common cations and anions have been included to test the selectivity of CO1, and the results were satisfactory. There is no obvious change in PL intensity when other ions are added. One of the keys that lead to the high selectivity towards Ru3+ is the partial overlapping of the CO1 emission peak and the Ru3+ absorption peak. Also, possessing an extensive aromatic system, CO1 is an electron-rich species. On the other hand, Ru3+ is electron-deficient, and the electron transfer from CO1 to Ru3+ again results in quenching of the fluorescence. The combination of these two factors makes CO1 not only sensitive but also a highly selective sensor for Ru3+. Moreover, the PL intensity change shows a linear trend versus Ru3+ concentration, indicating the potential of CO1 to act as a quantitative sensor. High porosity is not the only characteristic that allows CO1 to adsorb Ru3+ efficiently. As for the adsorbing performance, the high porosity of the structure and the presence of numerous lone pair electrons on oxygen and nitrogen atoms provide good electrostatic interaction between the CO1 skeleton and cationic Ru3+ for anchoring of the ions. The maximum equilibrium adsorption capacity was calculated to be 208 mg g−1. The Ru3+ equilibrium adsorption isotherm of CO1 is shown in Fig. 41e and f. Hg2+ is also a poisonous cation that usually requires detecting and removal.
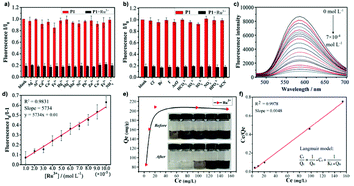 |
| Fig. 41 (a) The fluorescence ratio of CO1 in the presence of various metal ions including blank, Ag+, Al3+, Cd2+, Co2+, Fe3+, Hg2+, Mg2+, Mn2+, Ni2+, Pd2+, Zn2+, Cu2+, Fe2+, NH4+ (10−3 M) before and after the addition of Ru3+ (5 × 10−4 M). (b) The fluorescence ratio of CO1 in the presence of various anions including blank, Cl−, Br−, I−, AcO−, HCO3−, SO32−, SO42−, NO3−, HPO42−, SCN− (10−3 M) before and after the addition of Ru3+ (5 × 10−4 M). (c) Fluorescence intensity of CO1versus concentration variation of Ru3+. (d) The linearity of THPP to Ru3+ (excitation wavelength: 410 nm, speed: 1200 nm min−1, slit: 10/10, VDMF : VH2O = 5 : 5). (e) The equilibrium adsorption isotherm of CO1 for Ru3+. (f) Linearity of the equilibrium adsorption data with Langmuir model (Cadsorbent = 0.25 mg mL−1). Reprinted from ref. 35. Copyright 2020, with permission from Elsevier. | |
3.2 Optoelectronics
Apart from the conventional utilization of AIE macromolecules in the fluorescent sensing arena, the AIE effect also grants AIE-active macromolecules the possibility of being exploited in optoelectronic fields (applications like organic light-emitting diodes (OLEDs) and other electroluminescent devices).
L6 (developed by Chen et al.) is another AIE-active macromolecule with great electrofluorochromic (EFC) performance.52 EFC materials can exhibit reversible fluorescence in response to electrochemical redox.47 Much attention has been given to EFC materials due to their possible application in display, sensing, etc.48–51 As shown in Fig. 8b, when designing the polymer L6, aliphatic groups were included in the polymer backbone to prevent through-bond charge transfer. Designed as an EFC polymer, L6 can have its fluorescence switched off when a potential is applied to it. And upon applying a reverse potential, the once-gone fluorescence can be restored (Fig. 42). In addition to its EFC characteristics, L6 also possesses the highest fluorescence contrast ratio so far (417 when fully activated, 36.1 when partially activated for 20 seconds); high fluorescence quantum yield (69.1%); and high fatigue resistance (EFC active even after hundreds of cycles).
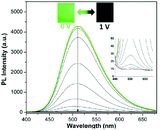 |
| Fig. 42 PL spectra changes of L6 thin film at different potentials (inset shows the optical images of polymer film under excitation of 365 nm with applied potential of 0 and 1 V). Adapted with permission from ref. 52. Copyright 2018 American Chemical Society. | |
L16a and L16b (Fig. 43a and b), which were developed by Xie and co-workers to be AIE-active liquid-crystalline polymers (LCPs), are capable of being utilized as OLEDs, 3D imaging systems, optical information storage systems, etc.140 LCPs have attracted much attention due to their special attributes like anisotropic properties, self-assembling capabilities, etc. By introducing AIE moieties into LCPs, AIE-active LCPs can be designed and synthesized. L16a and L16b are confirmed to have a smectic phase by polarized light microscopy and wide-angle X-ray diffraction. A special characteristic of L16a and L16b is ordering enhanced emission (OEE). OEE refers to the phenomenon whereby the emission intensity of a material can be further enhanced when the molecules adopt a certain molecular arrangement, as can be seen in Fig. 43c–e. The annealing process allowed L16a and L16b to take a more compact arrangement, and the emission intensities after annealing were approximately 2-fold higher than those before.
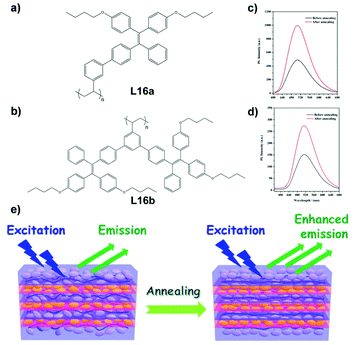 |
| Fig. 43 Structures of L16a (a) and L16b (b). A comparison of the fluorescence intensity spectra of L16a (c) and L16b (d) before and after annealing; excitation wavelength: 350 nm. (e) A schematic diagram of the possible molecular arrangements and the emissive variations of L16a and L16b before and after annealing. Adapted from ref. 140. Copyright 2019 The Royal Society of Chemistry. | |
Photodetectors detect light and convert the light into current, with wide applications like safety monitoring, medical imaging, etc.141–145 In recent years, AIE polymers have been developed for photodetector applications. Designed to be a multifunctional polymer, L17, which is capable of realizing electrochromism, electrofluorochromism, and photodetection, was reported by Wang and co-workers.146 As shown in Fig. 44, L17 has various versions, which depend on the monomers used in the synthesis. Both the syntheses of monomers and the syntheses of L17 are done via classic Suzuki coupling. The resulting polymer, when cast into films, shows a rapid rise in electrical signal when illuminated by a 500 W xenon arc lamp. The recorded photovoltaic changes are plotted in Fig. 44b–e, indicating L17 to be an excellent photodetector.
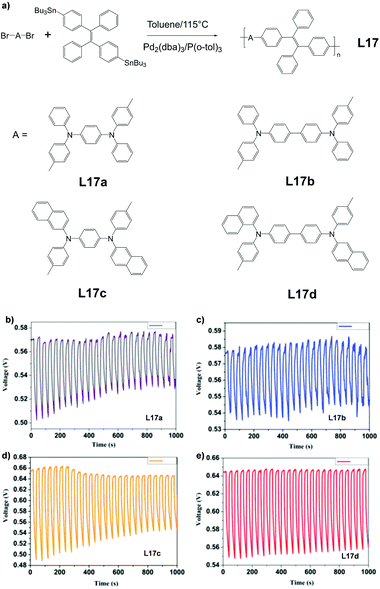 |
| Fig. 44 (a) Synthetic route of L17a-d. (b–d) Photovoltaic response of L17a-d polymer films immobilized on indium tin oxide (ITO) glass upon exposure to light with switching at room temperature. Reprinted from ref. 146, Copyright 2019, with permission from Elsevier. | |
3.3 Bioimaging
In modern medical treatments, bioimaging plays a vital role in applications such as cell tracking, visual guiding of surgeries, etc. In these fields of application, AIE materials have intrinsic advantages over traditional ACQ-dominated materials, such as high photobleaching threshold, high concentration accessibility, excellent PL intensity, long-term bioimaging capability, etc.147–149 Moreover, the EPR effect states that molecules of a certain size (usually nanoparticles and macromolecules) have a much higher tendency to accumulate in tumor tissues than in other normal tissues.150,151 Thus if a bioimaging agent is targeting tumor tissues, it will experience much higher concentration in the targeted area and hence leading to aggregation of the bioimaging material. This again leads to the conclusion that AIE materials are superior bioimaging materials compared to conventional ACQ-dominated materials. All these advantages originate from the fundamental difference between AIE and ACQ, which has been discussed in the introduction (section 1). To take these advantages into clinical applications, researchers worldwide have developed various kinds of AIE-based bioimaging agents, and some of them have proved to be quite effective and applicable.
AIE macromolecule L3 is an AIE-active polymer with two-photon fluorescence.45 Two-photon fluorescence refers to fluorescence that requires two photons rather than one photon for excitation and has a far better penetration depth than conventional one-photon fluorescence due to less absorption and scattering of less energetic photons by living tissue.148,149Fig. 45a and b shows the PL intensity, emission wavelengths, and fluorescence reversibility of L3 in different states. The emission peak of L3 can be shifted dramatically, from about 430 nm (as-synthesized sample) to around 525 nm (ground sample). This fact indicates that L3 is a stimulus-responsive AIE macromolecule (same as SS1 and H2). Because of the use of polyethylene glycol as part of the polymer, L3 possesses excellent biocompatibility, which is an essential requirement for biological applications (Fig. 45c and d). Due to the existence of hydrophilic groups in L3, its water dispersity is excellent and it is capable of self-assembling into nanoparticles with hydrophilic shells and hydrophobic cores. In Fig. 45e–f, we can see that after forming nanoparticles, its good water dispersity, biocompatibility, and photoluminescence intensity allow L3 to present a clear cell image. This proves the potential of L3 to act as a good bioimaging agent.
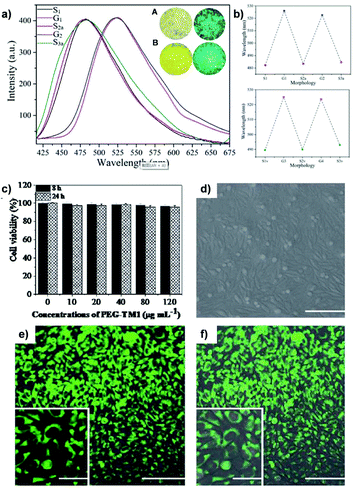 |
| Fig. 45 (a) The fluorescence spectra of L3: (S1) as-synthesized sample; (G1) ground sample; (S2a) annealed sample (the ground sample was homoisothermal at 150 °C for 0.5 h and cooled down at room temperature); (G2) re-ground sample; (S3a) re-annealed sample. The insets show the differences between the different samples: (A) as-synthesized sample of TPMA dye under ambient light (left) and UV light with an excitation of 365 nm (right); (B) ground sample of L3 under ambient light (left) and UV light with an excitation of 365 nm (right). (b) The reversibility of the two-photon fluorescence wavelengths of L3: by grinding-annealing treatments (top); and by grinding-fuming treatments (bottom): (S2v) fumed sample (ground sample in dichloromethane vapor for five minutes); (S3v) re-fumed sample. Biocompatibility evaluations and cell imaging of L3 fluorescent organic nanoparticles (FONs). (c) Cell viability of L3 FONs with L929 cells; (d–f) confocal laser scanning microscope images of L929 cells incubated with 20 mg mL−1 of L3 FONs: (d) bright field, (e) excited with a 405 nm laser, (f) merged image of (d) and (e). Scale bar = 100 mm. Inset is the local zoom image. Scale bar = 50 mm. Adapted from ref. 45. Copyright 2020 The Royal Society of Chemistry. | |
Osteogenic differentiation, an important process for bone growth and repair, is a process that requires a dynamic tracking method. Conventional ways of tracking usually require halting or fixation of osteocytes, thus not allowing consistent dynamic tracking. L5, which provides a solution for the dynamic tracking of osteogenic differentiation reported by Zhang et al., was designed to solve this problem.46 As we can see from Fig. 46a, L5 has its fluorescence turned on very specifically to the selected differentiated osteocytes (differentiated MC3T3-E1), not even responding to the parental undifferentiated cells of the detected ones. Moreover, L5 shows almost no cytotoxicity and much higher mean fluorescence intensity than commercially available imaging agents (Alizarin Red S) during the whole imaging process for MC3T3-E1 (Fig. 46b).
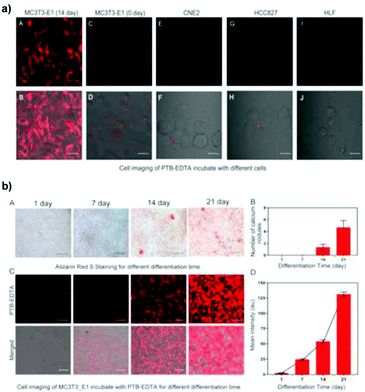 |
| Fig. 46 (a) Fluorescence performance of L5 with different cells. (b) Comparison of fluorescence performance of L5 and Alazarin Red S over 21 days. Reprinted from ref. 46. Copyright 2020, with permission from Elsevier. | |
L18, an AIE-active linear polymer (Fig. 47a), was synthesized and reported by Haddleton and co-workers.138 Synthesized by copper(0)-mediated reversible deactivation radical polymerization (Cu-RDRP) with a TPE-based initiator, L18 enjoys a simple, facile synthesis route, low polymer dispersity, good conversion rate, high biocompatibility, good fluorescence performance, etc. L18 is a block copolymer with two changeable sections. By varying the monomers used for synthesis, the properties of L18 can be tuned easily. For instance, the hydrophobic nature of L18 can be altered to be amphiphilic by changing one of the hydrophobic monomers for a hydrophilic monomer to obtain a hydrophilic section on the polymer chain. Moreover, the already synthesized L18 variant with tert-butyl groups on it can also be transformed into an amphiphilic polymer via deprotection. This feature allows pH-controlled self-assembly, which leads to the formation of nanoparticles in an aqueous solution. These AIE-active nanoparticles will have their sizes affected by the pH value of the aqueous solution, giving L18 pH-responsiveness. Further investigations show that changing the pH value affects the PL intensity and nanoparticle size of L18 drastically. Further investigations on biocompatibility (Fig. 47b) proved that L18 exhibits very low cytotoxicity. Also, comparison with commercial lysosome agent LysoTracker Green shows that L18 stains lysosomes preferably (Fig. 47c) and provides much better fluorescence after 50 scans.
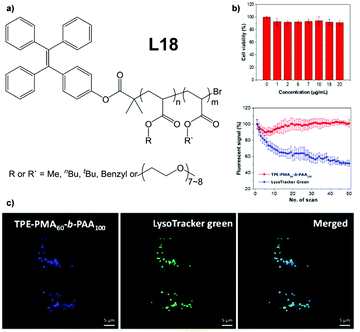 |
| Fig. 47 (a) Structure of L18. (b) Cell viability of 4 T1 cells in the presence of L18 at different concentrations. (c) Change in the fluorescent signal from 4 T1 cells stained with L18 (10 μg mL−1, 3 h) and LysoTracker Green (1 μM, 30 min) with the scan time. Excitation wavelength: 405 nm. (d) Colocalization images of 4 T1 cells stained with L18 (10 μg mL−1, 3 h) and LysoTracker Green (1 μM, 30 min) and their merged image. Excitation wavelength: 405 nm (L18) and 488 nm (LysoTracker Green); emission filter: 410–500 nm (L18) and 500–550 nm (LysoTracker Green). Scale bar = 5 μm. Adapted with permission from ref. 138. Copyright 2020 American Chemical Society. | |
Due to less tissue autofluorescence and photon scattering, second near-infrared region (NIR-II, about 1000–1700 nm wavelength) emission is highly preferred for in vivo fluorescence bioimaging over the visible (380–700 nm wavelength) and NIR-I region (700–900 nm wavelength), owing to better bioimaging quality and depth.152–155 In 2020, the first AIE-active polymer, L19, emitting in the NIR-II region was reported by Tang and co-workers.156 The structure of L19 is illustrated in Fig. 48a. During the research, Tang and co-workers found that for linear AIE polymers, highly coplanarity of aromatic moieties (e.g., planar polymer) causes strong absorption but poor quantum yield. On the contrary, low coplanarity of aromatic moieties (e.g., twisted polymer) exhibits good quantum yield but weak absorption. Ideally, AIE polymers for bioimaging applications need to show good performance in both absorption and quantum yield. In light of the relation between planarity and quantum yield/absorption, L19 was designed to have a planar and twisted structure. This design strategy successfully led to both high quantum yield and absorption. Moreover, the fact that the emission tail of L19 reaches into the NIR-IIa region (1300–1400 nm, Fig. 48b) makes it the first reported NIR-II-emitting AIE polymeric material. When combined with a pH-responsive poly(β-amino ester), the resultant L19 nanoparticle shows enhanced accumulation in tumor tissue, making it a good bioimaging material for surgery visualization, as illustrated in Fig. 48c.
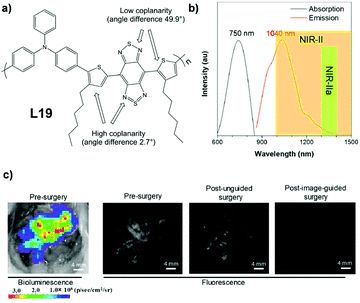 |
| Fig. 48 (a) Structure of L19. (b) Absorption and emission spectra of L19. (c) Bioluminescence and NIR-II fluorescence images of L19 in abdominal cavity before and after tumor resection. Adapted with permission from ref. 148. Copyright 2020 American Chemical Society. | |
3.4 Therapeutics
Owing to their exceptional emission properties in a high concentration/aggregated state, AIE macromolecules integrated with diagnostic and therapeutic functions have drawn great research attention. Compared to normal drugs, AIE polymers provide a pivotal handle for clinical monitoring, which is their fluorescence. The diffusion, delivery, and pathogen-killing processes can be recorded, monitored, and visualized much easier with the fluorescent signals of AIE macromolecules. The AIE characteristics allow AIE-active compounds to be applied in much higher concentrations than traditional ACQ-dominated therapeutic/diagnostic compounds, emphasizing the importance of AIE compounds in therapeutic applications.9,89,157–160
PDT is a localized and spatiotemporally controlled treatment of cancerous cells, bacteria, and other harmful microorganisms via cytotoxic ROS generated by PSs under exposure to light stimulus, which is advantageous for selectivity, low systematic toxicity, minimized drug resistance, etc.55,161,162 Due to AIEgens with facile ways to tune exciton decay pathways (nonradiative and radiative decay), this new class of fluorophores has been customized to highly effective PSs that lead to strong ROS generation and bright fluorescence emission, which has attracted increasing research attention in recent years.163,164 For instance, L20, a drug for PDT and bacterial infection, was designed and synthesized by Tang et al. (Fig. 49).157 Along with the long history of antibiotic usage, many antibiotic-resistant and multi-antibiotic-resistant bacteria strains have appeared. To fight against these bacteria, the development of new therapeutics is of great importance. ROS are effective weapons to fight off bacteria. Hence, ROS-generating chemicals have attracted a lot of interest. AIE polymers with good D–π-A interaction that could work as PSs to generate ROS are good candidates for PDT, and L20 is a representative example. By incorporating the conjugated backbone and 4-azidoperfluorobenzoate (APFB) on the side chain (Fig. 49a), L20 successfully actualized effective generation of ROS and radicals in its aggregated state. Besides its ROS-generating ability, L20 binds to bacteria selectively (Fig. 49b) due to the existence of quaternary ammoniums in the middle of side chains, which renders it hydrophilic. Further investigation showed that L20 generates ROS under both white light and sunlight, and the ROS generation sees a drastic increase if L20 aggregates. Based on this, in vitro antibacterial tests and bacteria inhibition tests were conducted for L20, and the results are illustrated in Fig. 49c–i. Almost 100% inhibition efficiency was recorded, and the in vitro antibacterial test results are satisfactory as well.
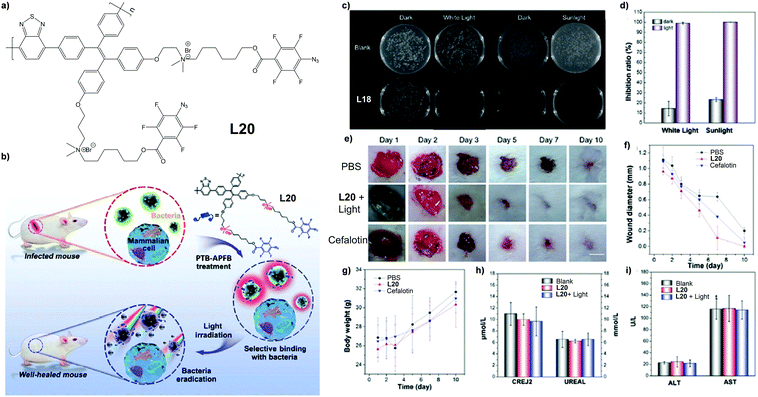 |
| Fig. 49 (a) Structure of L20. (b) Selective binding of L20 onto bacteria. (c) Photographs of NB agar plate and (d) biocidal activity of S. aureus with L20 treatment in dark, and under white light and sunlight. (e) Photographs of the S. aureus-infected skin of mice during treatment with the different formulations, and (f) the size of the infected area as well as (g) the body weights of the mice. Scale bar = 1 cm. (h) Levels of CREJ2 and UREAL, and (i) ALT and AST in blood samples from mice with different treatments. Adapted from ref. 157. Copyright 2020 Wiley-VCH. | |
Compared to the traditional one-photon PDT, as an alternative, two-photon PDT has a superior attribute, which is better penetration strength. One-photon PDT usually requires visible light excitation, which has insufficient penetration depth for deep targets.162,165 For two-photon PDT, PSs can absorb two photons of lower energy to achieve the excited state. The excitation by two photons in the near-infrared (NIR) region greatly cuts down tissue absorption and scattering, thus making possible PDT for deep tissue.164,166 To take advantage of two-photon PDT, many AIE-active PSs have been developed.163,167,168 A good example of a polymeric AIE material that is capable of performing two-photon PDT, SM7, has recently been reported by Liu et al.158SM7 consists of three components, of which one is an AIE-active two-photon PS, M16, one is an amphiphilic polymer, DSPE-PEG-MAL, and the final one is an HIV-1 trans-activator (RKKRRQRRRC) for surface functionalization, as shown in Fig. 50. Due to the advantage of two-photon fluorescence (two-photon fluorescence basically has the same advantage as two-photon PDT, providing better penetration), at 8 mg kg−1 concentration in mouse, the fluorescence managed to provide a clear image at a depth of 200 μm. As for the PDT efficiency, when incubated with HeLa cells at 10 μg mL−1 concentration, it takes 20 scans of 800 nm, 20 mW radiation to kill 50% of cells, indicating a high PDT efficiency.
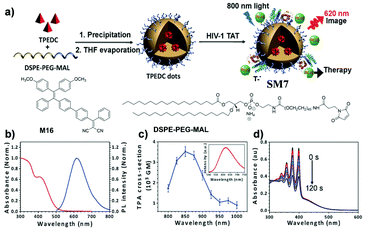 |
| Fig. 50 (a) The synthetic route of SM7. (b) Normalized UV–visible absorption (red) and PL (blue) spectra of SM7. (c) Two-photon absorption cross section of SM7 at different wavelengths. The inset shows the two-photon-induced fluorescence spectrum. (d) UV–visible spectra of ABDA in the presence of SM7 under light irradiation (60 mW cm−2, 400–700 nm) in water. Adapted from ref. 158. Copyright 2017 Wiley-VCH. | |
Apart from the drug itself, the drug delivery agent is also essential for successful therapy. Utilizing the AIE characteristic, AIE macromolecules can be fabricated into multifunctional drug delivery agents, which serve as excellent delivery process visualizers as well. SM2 is a good example of such a drug delivery agent.9 Thanks to its carefully designed structure, as carrier of anticancer drug DOX, SM2 realized pH/redox dual response, enabling it to perform quick and selective drug release in cancer cells (Fig. 51a–d). Through the FRET effect between drug molecule and SM2, detailed, dynamic monitoring of drug delivery and release processes is also made possible (Fig. 51e–f). In addition, the inclusion of PEG moieties in the structure increases its biocompatibility, facilitating the intake of the drug carrier into cells via endocytosis rather than permeation.
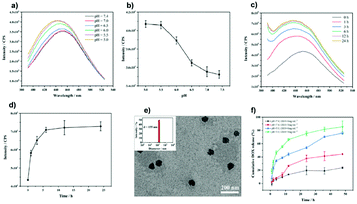 |
| Fig. 51 (a) Fluorescence spectra of SM2 in phosphate-buffer saline (PBS) solution at different pH values and (b) intensity at 445 or 450 nm. (c) Fluorescence spectra of SM2 in aqueous solution with the addition of glutathione (GSH) (3 mg mL−1) and (d) intensity at 440 or 455 nm against time. (e) TEM image and DLS analysis (inset) of SM2/CD@DOX. (f) Release of DOX from DOX-loaded SM2 with different pH values (5.0 or 7.4) and GSH concentrations (0 or 3 mg mL−1). Adapted with permission from ref. 9. Copyright 2019 American Chemical Society. | |
From the exploration of cancer treatment options, lysosomal cell death has been found to have a significant therapeutic effect on apoptosis and drug-resistant cancer.169,170 The development of lysosomal targeted drugs is a new type of cancer treatment strategy. Similar to SM2, SM8 is cleverly designed to integrate all cancer cell targeting, tumor microenvironmental stimulus response, fluorescence imaging, lysosomal targeted chemotherapy, and nuclear-targeted chemotherapy functions for effective cancer treatment.171 The construction of the SM8 system involves two steps (Fig. 52a). First, the amphiphilic polymers Biotin-PEG-HydPCL and mPEG-Hyd-PCL-CIN self-assemble to form nanoparticles in water, and then the AIE-active lysosomal targeted drug M17 and anticancer drug DOX are encapsulated into nanoparticle cores by hydrophobic interactions and π–π interactions. Benefiting from the spectral overlap between the emission of M17 and the absorption of DOX and the close distance between M17 and DOX in the core of SM8, FRET occurs between M12 and DOX to allow accurate tracking of the distribution and release of the drug (Fig. 52b–g). Moreover, M17 can also promote the escape of DOX lysosomes and avoid lysosome isolation. SM8 shows not only ideal biocompatibility and controllable drug release behavior, but also good fluorescence imaging and synergistic chemotherapy effects.
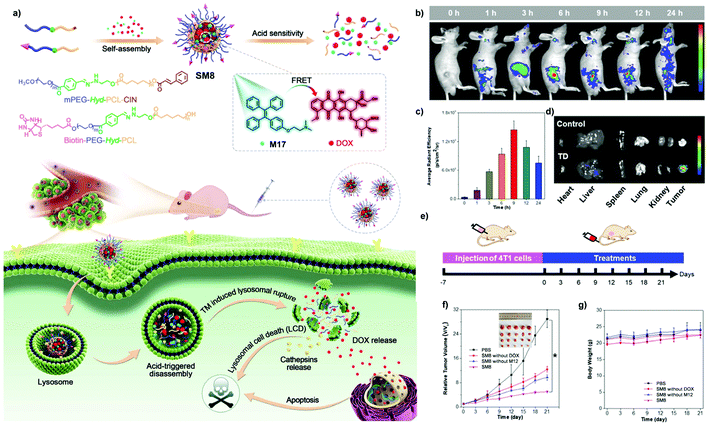 |
| Fig. 52 (a) Schematic illustration of the preparation process of visualized therapy nano-system SM8, and extracellular and intracellular processes for anticancer therapy. (b) In vivo fluorescence images of tumor tissues at different monitoring times after intravenous injection with SM8. (c) Quantitative analysis of mean FL intensity of tumor tissues in (b). (d) In vivo fluorescence images of tumor and major organs after intravenous injection with SM8 for 24 h. (e) The treatment process of tumor-bearing mice. (f) Time-dependent tumor growth curves and (g) body weight of tumor-bearing mice with various treatments. Adapted from ref. 171. Copyright 2021 Wiley-VCH. | |
Among all kinds of pathogens, ICB are especially harmful which can grow and multiply within the cells of a host. Like other bacteria, ICB have generally developed antibiotic resistance due to the overuse of antibiotics worldwide, so new drugs are urgently needed. A large number of chemicals have been developed to either kill ICB effectively and selectively or deliver drugs into cells. Scientists and researchers in the AIE area have also made many contributions to this. AIE polymer L4 is an anti-ICB drug carrier capable of delivering the drug deferoxamine-ciprofloxacin conjugated Fe3+ (DFeC) with high efficiency and selectivity.8 Same as SM2, as a carrier, L4 is designed to enable the FRET effect between the carrier and drug molecule as well. Hence the dynamic monitoring of the delivery and release process can be achieved (Fig. 53a ). By inclusion of mannose in the polymer structure, drug-loaded L4 nanoparticles can be taken up effectively by ICB-infected macrophages via endocytosis and then undergo degradation due to the existence of enzyme-responsive moieties in them. Along with the release of drug DFeC and degradation of L4, the FRET effect will gradually weaken, resulting in the restoration of the AIE effect of TPE units. According to further tests, low cell toxicity and rapid uptake are promised (Fig. 53b–f). The drug release rate and PL intensity data are demonstrated in Fig. 53g and h.
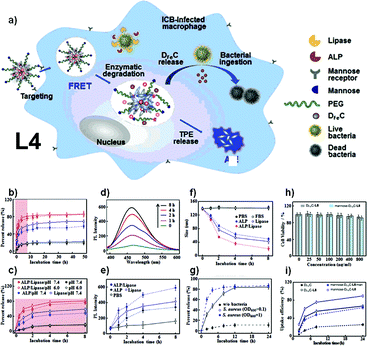 |
| Fig. 53 (a) DFeC-loaded L4 nanoparticles are internalized into macrophages via mannose-mediated endocytosis, followed by polymer degradation and DFeC release triggered by intracellular lipase and phospholipase of infected macrophages. DFeC is ingested along with iron flow into bacterial cytoplasm to destroy ICB, and the polymer degradation and DFeC release cause FRET alleviation and AIE restoration. (b) Percent release of DFeC from L4 after incubation for 48 h and (c) 4 h in buffers of pH 7.4 and pH 6.0 and in the presence of lipase and alkaline phosphate (ALP) (n = 3). (d) Fluorescence spectra (λex: 365 nm) after incubation for 8 h with lipase and ALP. (e) Time-dependent fluorescence intensities (λex: 365 nm; λem: 453 nm) after incubation in PBS and in the presence of lipase and ALP (n = 3). (f) Size change of nanoparticles after incubation for 8 h with lipase and ALP and in the presence of FBS (n = 3). (g) Percent release of DFeC after incubation with S. aureus suspensions with OD600 value of 0.1 and 1.0, using LB media without bacteria as control (n = 5). (h) Cell viability after incubation with DFeC-loaded L4 (DFeC-L4) and DFeC-loaded non-mannose L4 (mannose-DFeC-L4) nanoparticles up to 800 μg mL−1 (n = 5). (i) Cellular uptake efficiency of DFeC after incubation with free DFeC and DFeC-L4 and mannose-DFeC-L4 nanoparticles. Mannose was added as competitor for mannose-DFeC-L4 internalization (n = 5, mannose-DFeC-L4/man). Reprinted from ref. 8. Copyright 2020, with permission from Elsevier. | |
Inflammation is the response of the body towards harmful stimuli, like pathogen infection, irritation, etc. However, traditional drugs for inflammation treatment usually bring side effects with them or target the inflammation site precisely. To solve this problem, Wang and co-workers developed the AIE-active supramolecular assembly SM9 (Fig. 54).159SM9 consists of two main components: AIEgen-functionalized prednisolone (a common anti-inflammatory drug) M18,172 and amphiphilic block copolymer M19, which is produced by RAFT polymerization (Fig. 54a). With a well-programmed structure, M19 self-assembles into nanoparticles that can encapsulate M18 inside to produce SM9 (Fig. 54b). Upon contact with ROS, the nanoparticles will have part of their structures oxidized, thus releasing M18. M18 will, in turn, be oxidized and broken down into AIE-active fluorophores and drug prednisolone for inflammation treatment. This process allows the precise release of the drug at the inflammation site, release process tracking, and bioimaging via fluorescence. SM9 is thus regarded as a ‘theranostic nanoplatform’ by its inventors.
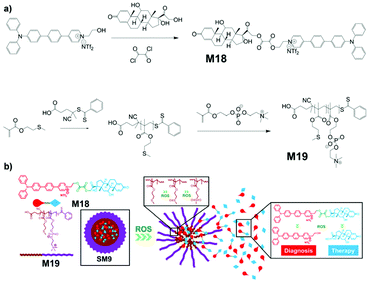 |
| Fig. 54 (a) Demonstration of structure of SM9 and its ROS response mechanism. (b) Synthetic route of M18 and M19. Adapted from ref. 159. Copyright 2020 American Chemical Society. | |
Gene therapy is a treatment that utilizes genetic materials as the drug for disease curing.84,86,173 As for many other drugs like DOX, genetic materials such as DNA and RNA also require a good carrier (gene vector) to be applied effectively. L21 is a photoactive AIE polymer that can be used for light-controlled gene delivery.89Fig. 55 shows the mechanistic illustration of gene carrying and delivery by L21. DNA for the therapy will bind to L21via electrostatic force then be encapsulated into the self-assembled nanoparticles of L21 formed in water. Subsequently, the nanoparticles will be engulfed into targeted cells via endocytosis. With visible light irradiation, generated ROS will destroy both the endo/lysosome capsule and L21 itself (L21 contains ROS-cleavable linkers). Upon destruction of the gene vector, the DNA molecules will be released and enter the nuclei for gene therapy. Moreover, the AIE characteristic of L21 means dynamic tracking of the release process is possible as well.
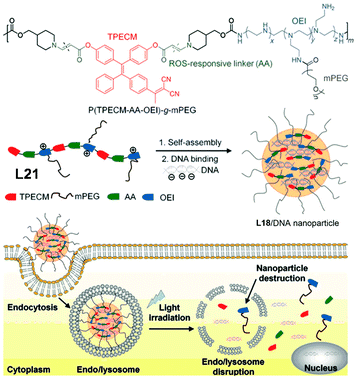 |
| Fig. 55 Mechanistic illustration of gene carrying and delivery by L21. Adapted from ref. 89. Copyright 2015 Wiley-VCH. | |
3.5 Printing
Printing of confidential information with chemicals only visible under specific conditions (e.g., UV radiation) has been utilized in fields like banknote identification, anti-counterfeiting, etc.174–176 Due to the excellent luminescence performance of AIE materials in their aggregated state, the possibility of applying them as printing ink has been investigated.
As one of the most popular printing techniques, inkjet printing has found use in almost every printing-related area due to its advantages like excellent resolution, good ink material tolerance, low pollution, etc.174,177,178 Recently, Chen et al. successfully synthesized multicolour AIE-active polymeric nanoparticles as colorants for inkjet printing.13 The AIEgens are actually encapsulated inside the polymeric nanoparticles rather than chemically bonded to them despite being called AIE nanoparticles. The encapsulation method allows facile syntheses of colorants to exhibit different colours by simply changing the AIEgen that will be encapsulated (Fig. 56a). In other words, the polymer obtained from miniemulsion polymerization is non-AIE-active and serves as a physical capsule for the AIEgens inside it, and the AIEgens are responsible for the PL properties of the nanoparticles and the final ink.179–182 The polymer used for encapsulation was styrene-butyl acrylate copolymer, with styrene at 80% of polymer weight and butyl acrylate at 20% polymer weight. Utilizing the synthesis strategy in Fig. 56b, multicolour AIE-active polymeric nanoparticles can be prepared from an emulsion of styrene monomer, butyl acrylate monomer, and AIEgens M20r, M20g, and M20b. When M20r, M20g, and M20b as ink are applied to the printing surface, their patterns are only visible under UV radiation, and exhibit high PL intensity and stability.
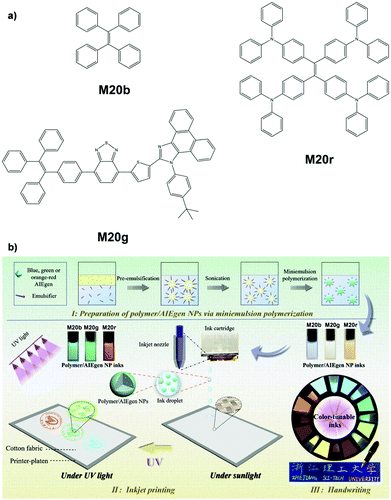 |
| Fig. 56 (a) Structures of M20r, M20g, and M20b. (b) Synthetic route and application methods of multicolour AIE-active polymeric nanoparticles. Reprinted from ref. 13. Copyright 2020, with permission from Elsevier. | |
3.6 Pollution elimination
Pollution has always been a big problem that needs to be tackled by all countries. One of the many ways to remove unwanted pollutants is by using specific chemicals, and AIE-active compounds find utility in this area as well.10,139,183
MO1, an AIE-active MOF reported by Liu et al., can be used to remove specific pollutants from an aqueous solution.10 It was confirmed that MO1 maintained its chemical properties well in an aqueous solution over a wide range of pH values from 0 to 11, and thermogravimetric analysis found MO1 has approximately 35% of its mass lost below 300 °C. Gas adsorption tests carried out with MO1 proved that it has a highly porous structure, which can take up N2 gas up to 345 cm3 g−1 at 77 K. Since hydroxides bonded on zirconium should have a high affinity towards Cr2O72−, this porous MOF should have high performance in Cr2O72− adsorption. Tests of Cr2O72− adsorption onto MO1 gave the result that the theoretical maximum Cr2O72−adsorption capacity of MO1 is 149 mg g−1, and the adsorption is quick and highly selective (Fig. 57a and b). The PL intensity of MO1 sees a great reduction upon adsorbing Cr2O72−, which makes it capable of serving as a “turn-off” sensor for Cr2O72− as well (Fig. 57c and d). With all these properties combined, MO1 is an excellent candidate for concurrent Cr2O72− detection and removal in water.
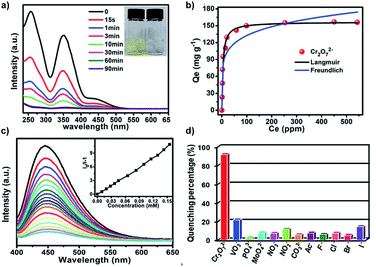 |
| Fig. 57 (a) UV-visible spectra of Cr2O72− in aqueous solution at different times during adsorption (inset: photographs of the Cr2O72− solution before and after adsorption). (b) Langmuir and Freundlich isotherm fitting plots of the adsorption amounts. (c) Emission spectra of MO1 with the incremental addition of Cr2O72− solution (inset: SV plot of the corresponding analyte). (d) Luminescence quenching percentage of MO1 in aqueous solutions with different anions. Adapted from ref. 10. Copyright 2019 The Royal Society of Chemistry. | |
3.7 Stimulus-responsive materials
Stimulus-responsive materials have their chemical/physical properties changed according to stimuli applied to them, including heat, light, chemicals, and mechanical force, and many AIE macromolecules have been designed to make use of this feature.95–97,184
3.7.1 Multi-stimuli-responsive materials.
Multi-stimuli-responsive materials can respond to more than one kind of stimuli, thus providing them with better performance for an existing application or expanding the application scope into another new area.96,97 Usually, stimuli-responsive materials change their properties in accordance with stimuli like temperature (macromolecule L20), specific chemicals (chemoresponsive),185,186 mechanical forces (section 3.7.2), etc.
AIE macromolecule H2 is a multi-stimuli-responsive AIE material. Upon contact with different organic solvents,37H2 exhibits different fluorescence intensities and emission wavelengths, as can be seen from Fig. 58a and b. Generally, the PL intensity of H2 decreases as the polarity of the solvent increases. The underlying mechanism arises from the high polarity of H2 itself, which results in better solubility in more polar solvents, thus leading to weaker AIE effect in polar solvents. Also, H2 shows a PL intensity increase along with temperature decrease. This can be explained by the destruction of supramolecular assemblies and higher degree of free motion in both intra- and intermolecular aspects at higher temperature. Higher free motions lead to more energy dissipation via the non-radiative pathway, thus cutting down fluorescence emission. In addition, H2 can also respond to specific metal ions via a change of its PL intensity (Fig. 58c and d). Fe3+ quenches the PL intensity of H2 greatly, while other metal ions appear to not quench the PL intensity of H2 to any significant level.
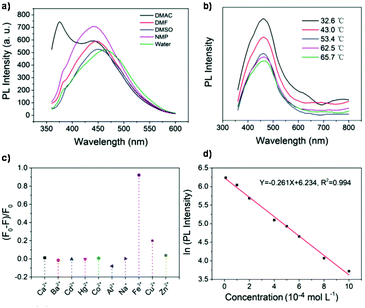 |
| Fig. 58 (a) Fluorescence spectra of H2 in various solvents at a concentration of 5 mg mL−1 (excited at 335 nm). (b) PL spectra of aqueous H2 solution at a concentration of 5 mg mL−1 with temperature increasing from 32.6 to 65.7 °C under excitation at 335 nm. (c) Fluorescence change ((F0 − F)/F0) of H2 (5 mg mL−1) in the presence of various metal ions (10−3 mol L−1) in aqueous solution. (d) The dependence of ln(PL intensity) on the concentration of Fe3+ in the range of (1–10) × 10−4 mol L−1 (excited at 335 nm; F0 and F are PL intensities at 464 nm in the absence and presence of Fe3+, respectively). Adapted from ref. 37. Copyright 2020 Wiley-VCH. | |
Multi-stimuli-responsive AIE-active supramolecular polymer AI-SM10 was reported by Lin and co-workers (Fig. 59 ).187 The ligand monomers SM10 in this polymer are linked together by Al3+ rather than linked by covalent bonds, and polymer chains π–π stack with each other to afford the final supramolecular assembly AI-SM10, which is a metallogel. As for the multi-stimuli responsiveness, AI-SM10 is thermo- and chemo-responsive. The same as H2, AI-SM10 shows fluorescence quenching along with increased temperature and recovers its fluorescence with decreased temperature. Contact with acids (trifluoroacetic acid or HCl) leads to fading of fluorescence, which can be restored by the addition of base (triethylamine or NaOH). Same as SS1, AI-SM10 can serve as an “on–off–on” type sensor to construct a new type of non-fluorescence bimetallic gel Fe-SM10 when responding to Fe3+/F−, with the “turn-off” process selectively working for Fe3+, and the “turn-on” process specifically working for F−. The fluorescence intensity of Fe-SM10 shows an approximately 4-fold increase from off state to on state when responding to Fe3+/F−, and an approximately 9-fold increase from off state to on state when responding to acid/base. Moreover, since AI-SM10 supramolecular assembly appears to be a metallogel, its adsorption rate towards Fe3+ was assessed, and the result came out to be 96.8%.
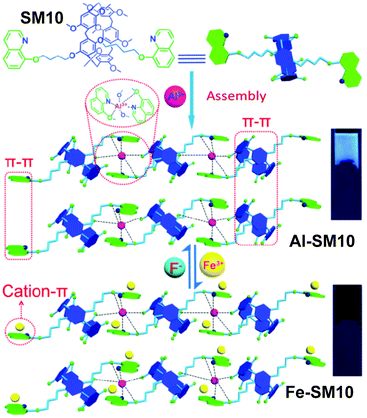 |
| Fig. 59 Schematic illustration of assembly process for supramolecular polymer metallogel SM10 and its sensing mechanism to ions. Adapted from ref. 187. Copyright 2020 Wiley-VCH. | |
AIE macromolecules L22a and L22b, which exhibit multi-stimuli-responsive FRET effect, were reported by Lin and co-workers (Fig. 60).184 The intriguing structures of L22a and L22b can be photoisomerized into each other (Fig. 60a). When activated by UV radiation, L22a emits green fluorescence at 517 nm, changing to L22b by photoisomerization. In L22b, the FRET process happens between the TPE moiety and SP moiety; and TPE and SP moieties are both fluorescent, leading to ratiometric fluorescence between 517 nm (TPE) and 627 nm (SP) (Fig. 60b–d). This highly reversible process can be triggered by various UV and visible light irradiation and interaction with cyanide ions (Fig. 60e and f). Upon combining with cyanide ions, the FRET process between the TPE moiety and SP moiety is prohibited, strengthening TPE moiety emission and loss of SP moiety emission. These processes contribute to L22b exhibiting high sensitivity towards cyanide ions as a chemosensor.
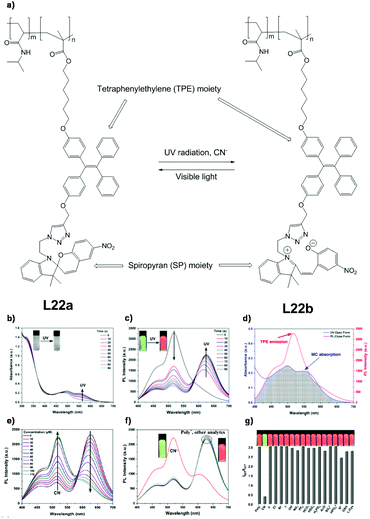 |
| Fig. 60 (a) Structure of L22a, L22b and how they are converted into each other. Time-dependent (b) absorption and (c) PL spectra (λex = 365 nm) of L22a and L22b in water upon UV exposure (0–90 s). Insets: photo-images of naked-eye observation and PL colour changes. (d) Spectral overlap of absorption spectra of L22b and emission spectra of L22a. (e) Titration profile change and hypsochromic shift of L22b after addition of different CN− concentrations. (f) PL spectra (insets: PL photo-images of polymer solution with/without cyanide ions), and (g) fluorescence intensity ratio response of L22b upon addition of various analytes (200 μM). Inset: PL photographs of polymer solutions in the presence of various analytes. Concentration: 1 g L−1, λex: 365 nm, MOPS buffer solution. Poly* = L22b. Adapted from ref. 184. Copyright 2020 American Chemical Society. | |
3.7.2 Mechanoresponsive materials.
A mechanoresponsive material, as its name implies, is a material that has its properties (e.g., colour, PL intensity, quantum yield) changed in response to a mechanical force applied to it, like stretching, scratching, compressing, etc.188,189 Introducing mechano-responsiveness into AIE-active macromolecules would provide a convenient way to alter their fluorescence emissions.
AIE macromolecule SS1 is not only a fluorescence “on–off–on” sensor but also a stimuli-responsive material.113 Classified as a “mechanoresponsive” material, SS1 changes its PL intensity and emission wavelength upon application of external force. As can be seen from Fig. 61, a pristine sample emits weakly while a ground sample emits strongly. Further investigation found that the emission wavelength experiences a bathochromic shift from 497 nm (before grinding) to 513 nm (after grinding), and the quantum yield of fluorescence increased to 13.54% from 0.92%. If the ground sample were further disturbed (e.g., scraped by a spatula), the PL intensity would decrease again. Moreover, if the SS1 sample was recrystallized, all the fluorescence-related properties will be restored to those of the pristine sample. The mechanistic cause of this interesting change is that grinding leads to disruption of the fragile intermolecular forces and change of morphology, thus resulting in the change of PL intensity and emission wavelength.
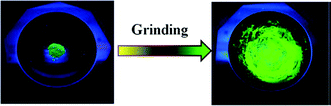 |
| Fig. 61 Photographic images of pristine and ground SS1 samples under a UV lamp. Adapted from ref. 113. Copyright 2019 American Chemical Society. | |
3.8 Microchips
Microchips have found applications in almost every area, along with the development and advancement of electronic technologies. Naturally, researchers started to investigate the possibility of combining certain chemicals with microchips to realize more specific and sophisticated functions, which stays true for AIE-active macromolecules. H5, a covalently co-crosslinkable AIEgens/epoxy macromolecular material, can be formed via femtosecond laser direct writing (FsLDW) or photolithography, as developed and reported by Sun and co-workers (Fig. 62).190 Designed for sensing volatile organic compounds (VOCs), H5 can be integrated onto microchips as optics units via FsLDW or photolithography to enable quick, easy, and sensitive VOC detection. Through stimulus-fluorescence transduction, the FRET-aided fluoro-chromatic effect, evanescent/refractive index waveguide effect, high recognizability, and sensitivity toward VOCs are made possible for H5. The multiple crosslinking-capable functional groups on the AIEgens used in H5 enable a high AIEgen doping ratio (max. 10 wt%). Combining with the AIE characteristics of H5, the blue, green, and red emitting H5 have fluorescence quantum yields of 68.8%, 57.7%, and 33.8%, respectively (in sold film state). In the presence of VOCs such as solvent vapours (e.g., tetrahydrofuran, acetone, ethanol), H5 will exhibit “turn-off” type detection, and the quenching ratio will vary based on different types of VOC and type of AIEgen integrated into H5.
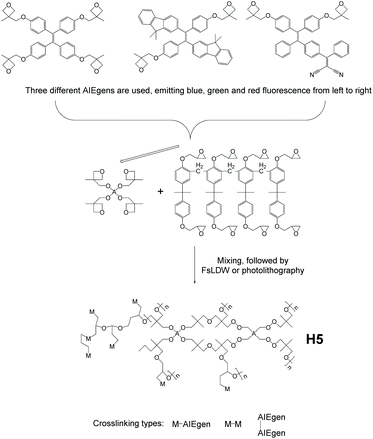 |
| Fig. 62 Synthetic route of epoxy composite H5 by FsLDW or photolithography. Adapted from ref. 190. Copyright 2020 The Royal Society of Chemistry. | |
3.9 Information storage
With the rapid development in information technology, the demand for information storage materials with large storage capacity and high-security performance has become increasingly urgent.191 As a new type of information storage, information coding has been widely used in everyday life.192 Generally, the current information coding usually can only store one type of information rather than a large amount of information. To improve the storage capacity of information coding, Tang et al. designed four AIE supramolecular hydrogels H6–9, which creatively applied AIE-active macromolecules to realize the storage of a large amount of information utilizing “code-in-code” (Fig. 63).193 AIE supramolecular hydrogels H6–9 are formed by self-assembly of PVA with three different fluorescent color molecules, M21r (red), M21y (yellow), and M21b (blue), through the formation of intermolecular hydrogen bonds with a large number of hydroxyl groups on the PVA chain (Fig. 63a). Because the movement of these AIE molecules in the hydrogel is restricted, the hydrogel is endowed with the ability to develop vibrant colors under ultraviolet radiation. Moreover, the black hydrogel H9 is obtained by covering the surface of hydrogel H8 with black tape. Due to the presence of many hydroxyl groups on the surface of the hydrogels, these hydrogels can afford assembled hydrogels H10 through interfacial supramolecular adhesion (Fig. 63b). H10 has a three-dimensional color code pattern, which displays various fluorescent colors under the irradiation of ultraviolet light, and its stored information can be scanned by mobile phones. Meanwhile, H10 can be introduced with one-dimensional and two-dimensional codes simultaneously by the form of “code-in-code”, to accomplish the centralized storage of rich one-dimensional, two-dimensional, and three-dimensional information (Fig. 63c).
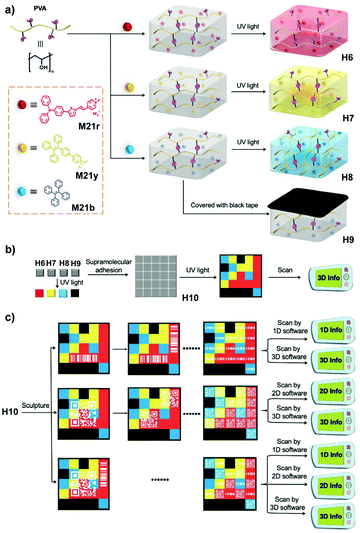 |
| Fig. 63 (a) Chemical structures of PVA, M21r, M21y, and M21b, and schematic representations of hydrogels H6, H7, H8, and H9 prepared by mixing PVA with M21r, M21y, and M21b, respectively. (b) Schematic representations of the formation of H10 through the interfacial supramolecular assembly of H6, H7, H8, and H9. (c) Schematic representations formed by incorporating 1D barcode patterns, 2D code patterns, and both 1D and 2D code patterns into H10. Adapted from ref. 193. Copyright 2021 Wiley-VCH. | |
4. Conclusions and future perspectives
In the past two decades, AIE research has grown in a booming fashion, thanks to the contribution of researchers all around the world. With their flexibility in designing, diversity in functionality, ease of tunability and seemingly infinite possibility, AIE macromolecules have already enjoyed much attention. So far, AIE macromolecules have been used in many areas like bioimaging, therapeutics, optoelectronics, sensing, etc. These areas are by no means the full collection of possible application areas for AIE macromolecules, as AIE-related technologies are still expanding their boundaries into more and more fields.
Nowadays, many AIE macromolecules follow the design protocol summarized as a “functional modular structure”. Under this protocol, monomers/segments with different targeted functionalities are fabricated together to realize the final multi-functional macromolecule. By swapping different modules, fine-tuning of properties of the polymers (e.g., tuning of absorption/emission wavelengths, hydrophilicity, analyte selectivity, PL intensity) can be achieved. This strategy provides AIE macromolecules with enormous flexibility compared to AIE small molecules, thus making them readily multi-functional. Another representative design protocol can be summarized as a “function through subunit collaboration”. Under this protocol, two or more subunits need to be joined together to realize a certain function. The advantage of this method is that functions that cannot be actualized via a single component can be achieved. Synthesis-wise, simplification of reaction process and improvement of purity and yield have been pursued and successfully achieved by many scientists. As the complexity and number of AIE macromolecules continue to rise, it can be predicted that more and more simple, facile, yet highly efficient syntheses will be demanded and developed to fulfill the growing needs for more AIE macromolecules.
With AIE characteristics and many other superior attributes brought by the AIE phenomenon, AIE macromolecules are anticipated to be promising candidates for many materials, like long-term cell imaging agents, OLEDs, sensors, etc. Through extensive research in recent years, more and more novel characteristics of AIE macromolecules have been revealed and exploited in terms of both laboratory theoretical aspects and practical applications. The designing and programming of AIE macromolecules have become more and more multifunctional, efficient, environmentally friendly, and reaching into many once unimaginable fields. We have reasons to believe that AIE macromolecules will continue to prosper and march forward with this trend. Also, the types and applications of AIE macromolecules, their design and synthesis strategies recapitulated in this review, are only representative ones instead of complete collections, as new ones are still being developed, thanks to the devotion of all researchers. We hope this review can provide researchers interested in AIE materials with a general picture of the most recent advances in AIE macromolecules, thus facilitating the deepening of understanding and keeping the speedy advance of this topic.
Conflicts of interest
There are no conflicts to declare.
Acknowledgements
This work was supported by National Natural Science Foundation of China (51673117, 51973118), the Science and Technology Innovation Commission of Shenzhen (JCYJ20170818093832350, JCYJ20170818112409808, JSGG20170824112840518, JCYJ20180507184711069, JCYJ20170818100112531, JCYJ20170817094628397, JCYJ20180305125319991), Key-Area Research and Development Program of GuangDong Province (2019B010929002, 2019B010941001), the Shenzhen Excellent Science and Technology Innovation Talents Training Project (RCBS20200714114910141), the Special Foundation for General Basic Research Program of Shenzhen (JCYJ20210324132816039), the Start-up Grant Harbin Institute of Technology (Shenzhen), China (HA45001108), and the Start-up Talent Grant at Harbin Institute of Technology (Shenzhen) (HA11409049).
References
- J. Luo, Z. Xie, J. W. Y. Lam, L. Cheng, B. Z. Tang, H. Chen, C. Qiu, H. S. Kwok, X. Zhan, Y. Liu and D. Zhu, Chem. Commun., 2001, 1740–1741 RSC
.
- Y. Hong, J. W. Y. Lam and B. Z. Tang, Chem. Commun., 2009, 29, 4332–4353 RSC
.
- G. F. Zhang, Z. Q. Chen, M. P. Aldred, Z. Hu, T. Chen, Z. Huang, X. Meng and M.-Q. Zhu, Chem. Commun., 2014, 50, 12058–12060 RSC
.
- Z. He, L. Shan, J. Mei, H. Wang, J. W. Y. Lam, H. H. Y. Sung, I. D. Williams, X. Gu, Q. Miao and B. Z. Tang, Chem. Sci., 2015, 6, 3538–3543 RSC
.
- N. L. C. Leung, N. Xie, W. Yuan, Y. Liu, Q. Wu, Q. Peng, Q. Miao, J. W. Y. Lam and B. Z. Tang, Chem. – Eur. J., 2014, 20, 15349–15353 CrossRef CAS PubMed
.
- R. Hu, N. L. C. Leung and B. Z. Tang, Chem. Soc. Rev., 2014, 43, 4494–4562 RSC
.
- X. Zhang, K. Wang, M. Liu, X. Zhang, L. Tao, Y. Chen and Y. Wei, Nanoscale, 2015, 7, 11486–11508 RSC
.
- M. Chen, J. He, S. Xie, T. Wang, P. Ran, Z. Zhang and X. Li, J. Controlled Release, 2020, 322, 326–336 CrossRef CAS PubMed
.
- Z. Dong, Y. Bi, H. Cui, Y. Wang, C. Wang, Y. Li, H. Jin and C. Wang, ACS Appl. Mater. Interfaces, 2019, 11, 23840–23847 CrossRef CAS PubMed
.
- J. Liu, Y. Ye, X. Sun, B. Liu, G. Li, Z. Liang and Y. Liu, J. Mater. Chem. A, 2019, 7, 16833–16841 RSC
.
- X. Chen, H. Gao, Y. Deng, Q. Jin, J. Ji and D. Ding, ACS Nano, 2020, 14, 5121–5134 CrossRef CAS PubMed
.
- F. Chen, J. Hu, X. Wang, S. Shao, L. Wang, X. Jing and F. Wang, Sci. China: Chem., 2020, 63, 1112–1120 CrossRef CAS
.
- X. Liang, M. Tao, D. Wu, B. Yu, Y. Mi, Z. Cao, Z. Zhao and D. Chen, Dyes Pigm., 2020, 177, 108287 CrossRef CAS
.
- R. Hu, A. Qin and B. Z. Tang, Prog. Polym. Sci., 2020, 100, 101176 CrossRef CAS
.
- M. Hu, H. T. Feng, Y. X. Yuan, Y. S. Zheng and B. Z. Tang, Coord. Chem. Rev., 2020, 416, 213329 CrossRef CAS
.
- J. Li, J. Wang, H. Li, N. Song, D. Wang and B. Z. Tang, Chem. Soc. Rev., 2020, 49, 1144–1172 RSC
.
- Z. Long, L. Mao, M. Liu, Q. Wan, Y. Wan, X. Zhang and Y. Wei, Polym. Chem., 2017, 8, 5644–5654 RSC
.
- X. Cai and B. Liu, Angew. Chem., 2020, 132, 9952–9970 CrossRef
.
- C. Gao and D. Yan, Prog. Polym. Sci., 2004, 29, 183–275 CrossRef CAS
.
- M. Jikei and M. Kakimoto, Prog. Polym. Sci., 2001, 26, 1233–1285 CrossRef CAS
.
- C. R. Yates and W. Hayes, Eur. Polym. J., 2004, 40, 1257–1281 CrossRef CAS
.
- B. I. Voit and A. Lederer, Chem. Rev., 2009, 109, 5924–5973 CrossRef CAS
.
- M. Calderón, M. A. Quadir, S. K. Sharma and R. Haag, Adv. Mater., 2010, 22, 190–218 CrossRef PubMed
.
- G. Feng, J. Liang and B. Liu, Macromol. Rapid Commun., 2013, 34, 705–715 CrossRef CAS PubMed
.
- V. Coessens, T. Pintauer and K. Matyjaszewski, Prog. Polym. Sci., 2001, 26, 337–377 CrossRef CAS
.
- J. M. Ren, T. G. McKenzie, Q. Fu, E. H. H. Wong, J. Xu, Z. An, S. Shanmugam, T. P. Davis, C. Boyer and G. G. Qiao, Chem. Rev., 2016, 116, 6743–6836 CrossRef CAS
.
- D. A. Tomalia, H. Baker, J. Dewald, M. Hall, G. Kallos, S. Martin, J. Roeck, J. Ryder and P. Smith, Polym. J., 1985, 17, 117–132 CrossRef CAS
.
- S. Kitagawa, R. Kitaura and S. Noro, Angew. Chem., Int. Ed., 2004, 43, 2334–2375 CrossRef CAS PubMed
.
- J. R. Li, R. J. Kuppler and H. C. Zhou, Chem. Soc. Rev., 2009, 38, 1477–1504 RSC
.
- S. R. Caskey, A. G. Wong-Foy and A. J. Matzger, J. Am. Chem. Soc., 2008, 130, 10870–10871 CrossRef CAS PubMed
.
- N. B. McKeown and P. M. Budd, Macromolecules, 2010, 43, 5163–5176 CrossRef CAS
.
- M. Köberl, M. Cokoja, W. A. Herrmann and F. E. Kühn, Dalton Trans., 2011, 40, 6834–6859 RSC
.
- Y. Cui, Y. Yue, G. Qian and B. Chen, Chem. Rev., 2012, 112, 1126–1162 CrossRef CAS PubMed
.
- D. Yuan, W. Lu, D. Zhao and H. C. Zhou, Adv. Mater., 2011, 23, 3723–3725 CrossRef CAS
.
- Y. Yan, H. Yang and H. Liu, Sens. Actuators, B, 2020, 319, 128154 CrossRef CAS
.
- M. Feng, L. Fang, F. Guan, S. Huang, Y. Cheng, Y. Liang and H. Zhang, Polymers, 2018, 10, 722 CrossRef
.
- L. Bai, H. Yan, L. Wang, T. Bai, L. Yuan, Y. Zhao and W. Feng, Macromol. Mater. Eng., 2020, 305, 2000126 CrossRef CAS
.
- L. Fang, C. Huang, G. Shabir, J. Liang, Z. Liu and H. Zhang, ACS Macro Lett., 2019, 8, 1605–1610 CrossRef CAS
.
- G. Wei, Y. Jiang and F. Wang, Tetrahedron Lett., 2020, 61, 151722 CrossRef CAS
.
- Y. Salinas, R. Martínez-Máñez, M. D. Marcos, F. Sancenón, A. M. Costero, M. Parra and S. Gil, Chem. Soc. Rev., 2012, 41, 1261–1296 RSC
.
- W. Z. Yuan, H. Zhao, X. Y. Shen, F. Mahtab, J. W. Y. Lam, J. Z. Sun and B. Z. Tang, Macromolecules, 2009, 42, 9400–9411 CrossRef CAS
.
- A. Qin, C. K. W. Jim, W. Lu, J. W. Y. Lam, M. Häussler, Y. Dong, H. H. Y. Sung, I. D. Williams, G. K. L. Wong and B. Z. Tang, Macromolecules, 2007, 40, 2308–2317 CrossRef CAS
.
- A. Qin, L. Tang, J. W. Y. Lam, C. K. W. Jim, Y. Yu, H. Zhao, J. Sun and B. Z. Tang, Adv. Funct. Mater., 2009, 19, 1891–1900 CrossRef CAS
.
- Q. Yin, J. Lu, Q. Lv and B. Han, Mater. Lett., 2020, 269, 127645 CrossRef CAS
.
- Z. Huang, Y. Chen, R. Wang, C. Zhou, X. Liu, L. Mao, J. Yuan, L. Tao and Y. Wei, RSC Adv., 2020, 10, 5704–5711 RSC
.
- Z. Zheng, T. Zhou, R. Hu, M. Huang, X. Ao, J. Chu, T. Jiang, A. Qin and Z. Zhang, Bioact. Mater., 2020, 5, 1018–1025 CrossRef PubMed
.
- P. Audebert and F. Miomandre, Chem. Sci., 2013, 10, 1018–1025 Search PubMed
.
- S. Seo, Y. Kim, Q. Zhou, G. Clavier, P. Audebert and E. Kim, Adv. Funct. Mater., 2012, 22, 3556–3561 CrossRef CAS
.
- A. Beneduci, S. Cospito, M. La Deda, L. Veltri and G. Chidichimo, Nat. Commun., 2014, 5, 3105 CrossRef PubMed
.
- H. Al Kutubi, H. R. Zafarani, L. Rassaei and K. Mathwig, Eur. Polym. J., 2016, 83, 478–498 CrossRef CAS
.
- J. Sun, Y. Chen and Z. Liang, Adv. Funct. Mater., 2016, 26, 2783–2799 CrossRef CAS
.
- N. Sun, K. Su, Z. Zhou, Y. Yu, X. Tian, D. Wang, X. Zhao, H. Zhou and C. Chen, ACS Appl. Mater. Interfaces, 2018, 10, 16105–16112 CrossRef CAS
.
- D. Schmaljohann, Adv. Drug Delivery Rev., 2006, 58, 1655–1670 CrossRef CAS
.
- X. Zhang, P. Yang, Y. Dai, P. Ma, X. Li, Z. Cheng, Z. Hou, X. Kang, C. Li and J. Lin, Adv. Funct. Mater., 2013, 23, 4067–4078 CrossRef CAS
.
- R. Lv, P. Yang, F. He, S. Gai, G. Yang, Y. Dai, Z. Hou and J. Lin, Biomaterials, 2015, 63, 115–127 CrossRef CAS
.
- W. Guan, S. Wang, C. Lu and B. Z. Tang, Nat. Commun., 2016, 7, 11811 CrossRef CAS
.
- C. Ma, G. Xie, Y. Tao, H. Zhu, Y. Zhang, Z. Chi, S. Liu and J. Xu, Dyes Pigm., 2021, 184, 108776 CrossRef CAS
.
- J. Mei, N. L. C. Leung, R. T. K. Kwok, J. W. Y. Lam and B. Z. Tang, Chem. Rev., 2015, 115, 11718–11940 CrossRef CAS PubMed
.
- J. Peng, A. P. Tomsia, L. Jiang, B. Z. Tang and Q. Cheng, Nat. Commun., 2021, 12, 4539 CrossRef CAS PubMed
.
- J. J. Griebel, S. Namnabat, E. T. Kim, R. Himmelhuber, D. H. Moronta, W. J. Chung, A. G. Simmonds, K.-J. Kim, J. van der Laan, N. A. Nguyen, E. L. Dereniak, M. E. Mackay, K. Char, R. S. Glass, R. A. Norwood and J. Pyun, Adv. Mater., 2014, 26, 3014–3018 CrossRef CAS PubMed
.
- M. E. Sosa Torres, A. R. Morales, A. Solano Peralta and P. M. H. Kroneck, Met. Ions Life Sci., 2020, 19–50 Search PubMed
.
- J. Zhang, Q. Zang, F. Yang, H. Zhang, J. Z. Sun and B. Z. Tang, J. Am. Chem. Soc., 2021, 143, 3944–3950 CrossRef CAS
.
- H. C. Kolb, M. G. Finn and K. B. Sharpless, Angew. Chem., Int. Ed., 2001, 40, 2004–2021 CrossRef CAS
.
- B. He, J. Zhang, J. Wang, Y. Wu, A. Qin and B. Z. Tang, Macromolecules, 2020, 53, 5248–5254 CrossRef CAS
.
- A. B. Lowe, C. E. Hoyle and C. N. Bowman, J. Mater. Chem., 2010, 20, 4745–4750 RSC
.
- B. D. Fairbanks, T. F. Scott, C. J. Kloxin, K. S. Anseth and C. N. Bowman, Macromolecules, 2009, 42, 211–217 CrossRef CAS PubMed
.
- A. B. Lowe, Polymer, 2014, 55, 5517–5549 CrossRef CAS
.
- H. Si, K. Wang, B. Song, A. Qin and B. Z. Tang, Polym. Chem., 2020, 11, 2568–2575 RSC
.
- J. Du, D. Huang, H. Li, A. Qin, B. Z. Tang and Y. Li, Macromolecules, 2020, 53, 4932–4941 CrossRef CAS
.
- D. Huang, Y. Liu, S. Guo, B. Li, J. Wang, B. Yao, A. Qin and B. Z. Tang, Polym. Chem., 2019, 10, 3088–3096 RSC
.
- G. Qi, F. Hu, Kenry, L. Shi, M. Wu and B. Liu, Angew. Chem., Int. Ed., 2019, 58, 16229–16235 CrossRef CAS
.
- A. Han, H. Wang, R. T. K. Kwok, S. Ji, J. Li, D. Kong, B. Z. Tang, B. Liu, Z. Yang and D. Ding, Anal. Chem., 2016, 88, 3872–3878 CrossRef CAS PubMed
.
- Y. Tang, A. Kang, X. Yang, L. Hu, Y. Tang, S. Li, Y. Xie, Q. Miao, Y. Pan and D. Zhu, Sens. Actuators, B, 2020, 304, 127300 CrossRef CAS
.
- C. Zhang, C. Liu, X. Xue, X. Zhang, S. Huo, Y. Jiang, W.-Q. Chen, G. Zou and X.-J. Liang, ACS Appl. Mater. Interfaces, 2014, 6, 757–762 CrossRef CAS PubMed
.
- S. K. Kim, D. H. Lee, J.-I. Hong and J. Yoon, Acc. Chem. Res., 2009, 42, 23–31 CrossRef CAS PubMed
.
- X. Zhang, C. Ren, F. Hu, Y. Gao, Z. Wang, H. Li, J. Liu, B. Liu and C. Yang, Anal. Chem., 2020, 92, 5185–5190 CrossRef CAS PubMed
.
- P. J. O'Brien and D. Herschlag, Biochemistry, 2002, 41, 3207–3225 CrossRef PubMed
.
- D. M. Bolarin, Haematologia, 2001, 31, 51–56 CrossRef CAS
.
- E. Terpos, O. Sezer, P. Croucher and M.-A. Dimopoulos, Blood, 2007, 110, 1098–1104 CrossRef CAS PubMed
.
- M. Haarhaus, V. Brandenburg, K. Kalantar-Zadeh, P. Stenvinkel and P. Magnusson, Nat. Rev. Nephrol., 2017, 13, 429–442 CrossRef CAS PubMed
.
- M. Rooseboom, J. N. M. Commandeur and N. P. E. Vermeulen, Pharmacol. Rev., 2004, 56, 53–102 CrossRef CAS PubMed
.
- R. Poupon, Hepatology, 2015, 61, 2080–2090 CrossRef CAS PubMed
.
- G. Qi, F. Hu, Kenry, K. C. Chong, M. Wu, Y. H. Gan and B. Liu, Adv. Funct. Mater., 2020, 30, 2001338 CrossRef CAS
.
- O. Boussif, F. Lezoualc'h, M. A. Zanta, M. D. Mergny, D. Scherman, B. Demeneix and J. P. Behr, Proc. Natl. Acad. Sci. U. S. A., 1995, 92, 7297–7301 CrossRef CAS PubMed
.
- J. Panyam and V. Labhasetwar, Adv. Drug Delivery Rev., 2003, 55, 329–347 CrossRef CAS PubMed
.
- L. Naldini, U. Blomer, P. Gallay, D. Ory, R. Mulligan, F. H. Gage, I. M. Verma and D. Trono, Science, 1996, 272, 263–267 CrossRef CAS
.
- K. A. Whitehead, R. Langer and D. G. Anderson, Nat. Rev. Drug Discovery, 2009, 8, 129–138 CrossRef CAS PubMed
.
- L. Alvarez-Erviti, Y. Seow, H. Yin, C. Betts, S. Lakhal and M. J. A. Wood, Nat. Biotechnol., 2011, 29, 341–345 CrossRef CAS PubMed
.
- Y. Yuan, C.-J. Zhang and B. Liu, Angew. Chem., Int. Ed., 2015, 54, 11419–11423 CrossRef CAS PubMed
.
- F. Zeng and S. C. Zimmerman, Chem. Rev., 1997, 97, 1681–1712 CrossRef CAS PubMed
.
- A. W. Bosman, H. M. Janssen and E. W. Meijer, Chem. Rev., 1999, 99, 1665–1688 CrossRef CAS PubMed
.
- A. E. Hargrove, S. Nieto, T. Zhang, J. L. Sessler and E. V. Anslyn, Chem. Rev., 2011, 111, 6603–6782 CrossRef CAS PubMed
.
- A. Bencini, F. Bartoli, C. Caltagirone and V. Lippolis, Dyes Pigm., 2014, 110, 169–192 CrossRef CAS
.
- C. Li, Y. Chen, L. Yang, C. Zhang, B. Wang and Y. Wang, Sens. Actuators, B, 2019, 291, 25–33 CrossRef CAS
.
- L. Sun, W. M. Huang, Z. Ding, Y. Zhao, C. C. Wang, H. Purnawali and C. Tang, Mater. Des., 2012, 33, 577–640 CrossRef CAS
.
- R. Cheng, F. Meng, C. Deng, H.-A. Klok and Z. Zhong, Biomaterials, 2013, 34, 3647–3657 CrossRef CAS PubMed
.
- A. Klaikherd, C. Nagamani and S. Thayumanavan, J. Am. Chem. Soc., 2009, 131, 4830–4838 CrossRef CAS PubMed
.
- J. Xue, W. Bai, H. Duan, J. Nie, B. Du, J. Z. Sun and B. Z. Tang, Macromolecules, 2018, 51, 5762–5772 CrossRef CAS
.
- B. R. Saunders and B. Vincent, Adv. Colloid Interface Sci., 1999, 80, 1–25 CrossRef CAS
.
- C. J. Ferguson, R. J. Hughes, D. Nguyen, B. T. T. Pham, R. G. Gilbert, A. K. Serelis, C. H. Such and B. S. Hawkett, Macromolecules, 2005, 38, 2191–2204 CrossRef CAS
.
- S. C. Thickett and R. G. Gilbert, Polymer, 2007, 48, 6965–6991 CrossRef CAS
.
- Y. Li and S. P. Armes, Angew. Chem., Int. Ed., 2010, 49, 4042–4046 CrossRef CAS PubMed
.
- J. P. Rao and K. E. Geckeler, Prog. Polym. Sci., 2011, 36, 887–913 CrossRef CAS
.
- G. Zhang and C. Wu, Phys. Rev. Lett., 2001, 86, 822–825 CrossRef CAS PubMed
.
- F. Tanaka, T. Koga and F. M. Winnik, Phys. Rev. Lett., 2008, 101, 028302 CrossRef PubMed
.
- D. Mukherji, C. M. Marques and K. Kremer, Nat. Commun., 2014, 5, 4882 CrossRef PubMed
.
- A. Chremos and J. F. Douglas, J. Chem. Phys., 2015, 143, 111104 CrossRef PubMed
.
- A. Chremos, E. Glynos and P. F. Green, J. Chem. Phys., 2015, 142, 044901 CrossRef PubMed
.
-
N. Hadjichristidis, M. Pitsikalis, H. Iatrou, P. Driva, G. Sakellariou and M. Chatzichristidi, Polymer Science: A Comprehensive Reference, Elsevier, 2012, pp. 29–111 Search PubMed
.
- A. Chremos, Soft Matter, 2017, 13, 5778–5784 RSC
.
- W. Zhu, J. Ling and Z. Shen, Macromol. Chem. Phys., 2006, 207, 844–849 CrossRef CAS
.
- K. Knoll and N. Nießner, Macromol. Symp., 1998, 132, 231–243 CrossRef CAS
.
- B. Tharmalingam, M. Mathivanan, G. Dhamodiran, K. S. Mani, M. Paranjothy and B. Murugesapandian, ACS Omega, 2019, 4, 12459–12469 CrossRef CAS PubMed
.
- F. J. M. Hoeben, P. Jonkheijm, E. W. Meijer and A. P. H. J. Schenning, Chem. Rev., 2005, 105, 1491–1546 CrossRef CAS PubMed
.
- T. Aida, E. W. Meijer and S. I. Stupp, Science, 2012, 335, 813–817 CrossRef CAS PubMed
.
- X.-W. Sun, Z.-H. Wang, Y.-J. Li, H.-L. Yang, G.-F. Gong, Y.-M. Zhang, H. Yao, T.-B. Wei and Q. Lin, Soft Matter, 2020, 16, 5734–5739 RSC
.
- D. Liu, M. Chen, K. Li, Z. Li, J. Huang, J. Wang, Z. Jiang, Z. Zhang, T. Xie, G. R. Newkome and P. Wang, J. Am. Chem. Soc., 2020, 142, 7987–7994 CrossRef CAS PubMed
.
- P. Wang, X. Miao, Y. Meng, Q. Wang, J. Wang, H. Duan, Y. Li, C. Li, J. Liu and L. Cao, ACS Appl. Mater. Interfaces, 2020, 12, 22630–22639 CrossRef CAS PubMed
.
- M. Bebelman, P. Bun, S. Huveneers, G. van Niel, D. Pegtel and F. Verweij, Nat. Protoc., 2020, 15, 102–121 CrossRef CAS PubMed
.
- J. Li, K. Peng, Y. Li, J. Wang, J. Huang, Y. Yan, D. Wang and B. Z. Tang, Angew. Chem., Int. Ed., 2020, 59, 21510–21514 CrossRef CAS PubMed
.
- N. Chaoui, M. Trunk, R. Dawson, J. Schmidt and A. Thomas, Chem. Soc. Rev., 2017, 46, 3302–3321 RSC
.
- J. Komeda, R. Shiotsuki, A. Rapakousiou, R. Sakamoto, R. Toyoda, K. Iwase, M. Tsuji, K. Kamiya and H. Nishihara, Chem. Commun., 2020, 56, 3677–3680 RSC
.
- C. S. Diercks and O. M. Yaghi, Science, 2017, 355, eaal1585 CrossRef PubMed
.
- J. Dong, X. Li, S. B. Peh, Y. D. Yuan, Y. Wang, D. Ji, S. Peng, G. Liu, S. Ying, D. Yuan, J. Jiang, S. Ramakrishna and D. Zhao, Chem. Mater., 2019, 31, 146–160 CrossRef CAS
.
- H. Furukawa, K. E. Cordova, M. O'Keeffe and O. M. Yaghi, Science, 2013, 341, 1230444–1230444 CrossRef PubMed
.
- L. E. Kreno, K. Leong, O. K. Farha, M. Allendorf, R. P. Van Duyne and J. T. Hupp, Chem. Rev., 2012, 112, 1105–1125 CrossRef CAS PubMed
.
- J. Lee, O. K. Farha, J. Roberts, K. A. Scheidt, S. T. Nguyen and J. T. Hupp, Chem. Soc. Rev., 2009, 38, 1450–1459 RSC
.
- M. Eddaoudi, Science, 2002, 295, 469–472 CrossRef CAS PubMed
.
- Y. Yang, G.-B. Hu, W.-B. Liang, L.-Y. Yao, W. Huang, Y.-J. Zhang, J.-L. Zhang, J.-M. Wang, R. Yuan and D.-R. Xiao, Nanoscale, 2020, 12, 5932–5941 RSC
.
- W. Shang, X. Zhu, T. Liang, C. Du, L. Hu, T. Li and M. Liu, Angew. Chem., Int. Ed., 2020, 59, 12811–12816 CrossRef CAS PubMed
.
- F. Song, Z. Zhao, Z. Liu, J. W. Y. Lam and B. Z. Tang, J. Mater. Chem. C, 2020, 8, 3284–3301 RSC
.
- M. Louis, R. Sethy, J. Kumar, S. Katao, R. Guillot, T. Nakashima, C. Allain, T. Kawai and R. Métivier, Chem. Sci., 2019, 10, 843–847 RSC
.
- J. Han, S. Guo, H. Lu, S. Liu, Q. Zhao and W. Huang, Adv. Opt. Mater., 2018, 6, 1800538 CrossRef
.
- Y. Wang, W. Wu, J. Liu, P. N. Manghnani, F. Hu, D. Ma, C. Teh, B. Wang and B. Liu, ACS Nano, 2019, 13, 6879–6890 CrossRef CAS PubMed
.
- N. M. Mahmoodi and J. Abdi, Microchem. J., 2019, 144, 436–442 CrossRef
.
- D. J. Tranchemontagne, J. R. Hunt and O. M. Yaghi, Tetrahedron, 2008, 64, 8553–8557 CrossRef CAS
.
- D. Britt, D. Tranchemontagne and O. M. Yaghi, Proc. Natl. Acad. Sci. U. S. A., 2008, 105, 11623–11627 CrossRef CAS PubMed
.
- C. Ma, T. Han, M. Kang, E. Liarou, A. M. Wemyss, S. Efstathiou, B. Z. Tang and D. Haddleton, ACS Macro Lett., 2020, 9, 769–775 CrossRef CAS
.
- G. Zhou, X. Zhang and X.-L. Ni, J. Hazard. Mater., 2020, 384, 121474 CrossRef CAS PubMed
.
- J. C. Zhu, Y. Guan, Z. W. Luo, Z. X. Li, H. H. Huang, P. Wang, Z. Shen and H. L. Xie, Polym. Chem., 2019, 10, 6342–6349 RSC
.
- C. Soci, A. Zhang, B. Xiang, S. A. Dayeh, D. P. R. Aplin, J. Park, X. Y. Bao, Y. H. Lo and D. Wang, Nano Lett., 2007, 7, 1003–1009 CrossRef CAS PubMed
.
- O. Lopez-Sanchez, D. Lembke, M. Kayci, A. Radenovic and A. Kis, Nat. Nanotechnol., 2013, 8, 497–501 CrossRef CAS PubMed
.
- P. Peumans, A. Yakimov and S. R. Forrest, J. Appl. Phys., 2003, 93, 3693–3723 CrossRef CAS
.
- E. Zheng, X. Zhang, M. R. Esopi, C. Cai, B. Zhou, Y.-Y. Lin and Q. Yu, ACS Appl. Mater. Interfaces, 2018, 10, 41552–41561 CrossRef CAS PubMed
.
- L. Shen, Y. Fang, H. Wei, Y. Yuan and J. Huang, Adv. Mater., 2016, 28, 2043–2048 CrossRef CAS PubMed
.
- Q. Lu, C. Yang, X. Qiao, X. Zhang, W. Cai, Y. Chen, Y. Wang, W. Zhang, X. Lin, H. Niu and W. Wang, Dyes Pigm., 2019, 166, 340–349 CrossRef CAS
.
- W. Qin, D. Ding, J. Liu, W. Z. Yuan, Y. Hu, B. Liu and B. Z. Tang, Adv. Funct. Mater., 2012, 22, 771–779 CrossRef CAS
.
- F. Helmchen and W. Denk, Nat. Methods, 2005, 2, 932–940 CrossRef CAS PubMed
.
- Q. Liu, B. Guo, Z. Rao, B. Zhang and J. R. Gong, Nano Lett., 2013, 13, 2436–2441 CrossRef CAS PubMed
.
- J. Fang, H. Nakamura and H. Maeda, Adv. Drug Delivery Rev., 2011, 63, 136–151 CrossRef CAS PubMed
.
- H. Maeda, J. Wu, T. Sawa, Y. Matsumura and K. Hori, J. Controlled Release, 2000, 65, 271–284 CrossRef CAS PubMed
.
- Y. Miao, C. Gu, Y. Zhu, B. Yu, Y. Shen and H. Cong, ChemBioChem, 2018, 19, 2522–2541 CrossRef CAS PubMed
.
- Y. Tang, F. Pei, X. Lu, Q. Fan and W. Huang, Adv. Opt. Mater., 2019, 7, 1900917 CrossRef CAS
.
- C. Li and Q. Wang, Adv. Ther., 2019, 2, 1900053 CrossRef
.
- S. Zhu, R. Tian, A. L. Antaris, X. Chen and H. Dai, Adv. Mater., 2019, 31, 1900321 CrossRef PubMed
.
- S. Liu, H. Ou, Y. Li, H. Zhang, J. Liu, X. Lu, R. T. K. Kwok, J. W. Y. Lam, D. Ding and B. Z. Tang, J. Am. Chem. Soc., 2020, 142, 15146–15156 CrossRef CAS PubMed
.
- T. Zhou, R. Hu, L. Wang, Y. Qiu, G. Zhang, Q. Deng, H. Zhang, P. Yin, B. Situ, C. Zhan, A. Qin and B. Z. Tang, Angew. Chem., Int. Ed., 2020, 59, 9952–9956 CrossRef CAS PubMed
.
- B. Gu, W. Wu, G. Xu, G. Feng, F. Yin, P. H. J. Chong, J. Qu, K.-T. Yong and B. Liu, Adv. Mater., 2017, 29, 1701076 CrossRef PubMed
.
- B. Ma, H. Xu, W. Zhuang, Y. Wang, G. Li and Y. Wang, ACS Nano, 2020, 14, 5862–5873 CrossRef CAS PubMed
.
- H. Huang, M. Liu, J. Chen, L. Mao, Q. Wan, Y. Wen, F. Deng, N. Zhou, X. Zhang and Y. Wei, Cellulose, 2019, 26, 8829–8841 CrossRef CAS
.
- T. J. Dougherty, C. J. Gomer, B. W. Henderson, G. Jori, D. Kessel, M. Korbelik, J. Moan and Q. Peng, J. Natl. Cancer Inst., 1998, 90, 889–905 CrossRef CAS PubMed
.
- M. R. Hamblin and T. Hasan, Photochem. Photobiol. Sci., 2004, 3, 436–450 CrossRef CAS PubMed
.
- W. Zhuang, L. Yang, B. Ma, Q. Kong, G. Li, Y. Wang and B. Z. Tang, ACS Appl. Mater. Interfaces, 2019, 11, 20715–20724 CrossRef CAS PubMed
.
- F. Bolze, S. Jenni, A. Sour and V. Heitz, Chem. Commun., 2017, 53, 12857–12877 RSC
.
- J. P. Celli, B. Q. Spring, I. Rizvi, C. L. Evans, K. S. Samkoe, S. Verma, B. W. Pogue and T. Hasan, Chem. Rev., 2010, 110, 2795–2838 CrossRef CAS PubMed
.
- D. Gao, R. R. Agayan, H. Xu, M. A. Philbert and R. Kopelman, Nano Lett., 2006, 6, 2383–2386 CrossRef CAS PubMed
.
- Z. Zheng, H. Liu, S. Zhai, H. Zhang, G. Shan, R. T. K. Kwok, C. Ma, H. H. Y. Sung, I. D. Williams, J. W. Y. Lam, K. S. Wong, X. Hu and B. Z. Tang, Chem. Sci., 2020, 11, 2494–2503 RSC
.
- Q. Kong, B. Ma, T. Yu, C. Hu, G. Li, Q. Jiang and Y. Wang, New J. Chem., 2020, 44, 9355–9364 RSC
.
- L. Shi, W. Wu, Y. Duan, L. Xu, Y. Xu, L. Hou, X. Meng, X. Zhu and B. Liu, Angew. Chem., Int. Ed., 2020, 59, 19168–19174 CrossRef CAS PubMed
.
- M. Borkowska, M. Siek, D. Kolygina, Y. Sobolev, S. Lach, S. Kumar, Y. Cho, K. Kandere-Grzybowska and B. Grzybowski, Nat. Nanotechnol., 2020, 15, 331–341 CrossRef CAS PubMed
.
- Y. Li, Q. Wu, D. Li, J. Huang, H. Wen, Y. Wu, D. Wang and B. Z. Tang, Adv. Funct. Mater., 2021, 31, 2106091 CrossRef CAS
.
- B. Ma, W. Zhuang, H. He, X. Su, T. Yu, J. Hu, L. Yang, G. Li and Y. Wang, Nano Res., 2019, 12, 1703–1712 CrossRef CAS
.
- T. Sorlie, C. M. Perou, R. Tibshirani, T. Aas, S. Geisler, H. Johnsen, T. Hastie, M. B. Eisen, M. van de Rijn, S. S. Jeffrey, T. Thorsen, H. Quist, J. C. Matese, P. O. Brown, D. Botstein, P. E. Lonning and A. L. Borresen-Dale, Proc. Natl. Acad. Sci. U. S. A., 2001, 98, 10869–10874 CrossRef CAS PubMed
.
- Y. Liu, F. Li, L. Qiu, K. Yang, Q. Li, X. Zheng, H. Hu, T. Guo, C. Wu and T. W. Kim, ACS Nano, 2019, 13, 2042–2049 CAS
.
- F. Zhang, Z. Shi, S. Li, Z. Ma, Y. Li, L. Wang, D. Wu, Y. Tian, G. Du, X. Li and C. Shan, ACS Appl. Mater. Interfaces, 2019, 11, 28013–28022 CrossRef CAS PubMed
.
- W. K. Tsai, Y. S. Lai, P. J. Tseng, C. H. Liao and Y.-H. Chan, ACS Appl. Mater. Interfaces, 2017, 9, 30918–30924 CrossRef CAS PubMed
.
- P. Sundriyal and S. Bhattacharya, ACS Appl. Mater. Interfaces, 2017, 9, 38507–38521 CrossRef CAS PubMed
.
- H. Shahariar, I. Kim, H. Soewardiman and J. S. Jur, ACS Appl. Mater. Interfaces, 2019, 11, 6208–6216 CrossRef CAS PubMed
.
- Z. Cao, C. Xu, L. Liang, Z. Zhao, B. Chen, Z. Chen, H. Chen, G. Qu, D. Qi, G. Shan and U. Ziener, Polym. Chem., 2015, 6, 6378–6385 RSC
.
- Z. Cao, X. Liang, H. Chen, M. Gao, Z. Zhao, X. Chen, C. Xu, G. Qu, D. Qi and B. Z. Tang, Polym. Chem., 2016, 7, 5571–5578 RSC
.
- X. Liang, Y. Hu, Z. Cao, L. Xiao, J. Lou, L. Liu, Y. Wang, Z. Zhao, D. Qi and Q. Cui, Dyes Pigm., 2019, 163, 371–380 CrossRef CAS
.
- Y. Hu, X. Liang, Z. Zhuang, Z. Cao, Q. Qi, Y. Wang, Y. Mi, Z. Zhao and Q. Cui, Polym. Chem., 2019, 10, 4220–4228 RSC
.
- D. Dai, Z. Li, J. Yang, C. Wang, J. R. Wu, Y. Wang, D. Zhang and Y. W. Yang, J. Am. Chem. Soc., 2019, 141, 4756–4763 CrossRef CAS PubMed
.
- P. Q. Nhien, W. L. Chou, T. T. K. Cuc, T. M. Khang, C.-H. Wu, N. Thirumalaivasan, B. T. B. Hue, J. I. Wu, S. P. Wu and H. C. Lin, ACS Appl. Mater. Interfaces, 2020, 12, 10959–10972 CrossRef CAS PubMed
.
- J. S. Park, K. Y. Yoon, D. S. Kim, V. M. Lynch, C. W. Bielawski, K. P. Johnston and J. L. Sessler, Proc. Natl. Acad. Sci. U. S. A., 2011, 108, 20913–20917 CrossRef CAS PubMed
.
- R. C. Bailey and J. T. Hupp, J. Am. Chem. Soc., 2002, 124, 6767–6774 CrossRef CAS PubMed
.
- J. He, Y. Zhang, J. Hu, Y. Li, Q. Zhang, W. Qu, H. Yao, T. Wei and Q. Lin, Appl. Organomet. Chem., 2020, 34, e5519 CAS
.
- Y. Sagara, S. Yamane, M. Mitani, C. Weder and T. Kato, Adv. Mater., 2016, 28, 1073–1095 CrossRef CAS PubMed
.
- D. A. Davis, A. Hamilton, J. Yang, L. D. Cremar, D. Van Gough, S. L. Potisek, M. T. Ong, P. V. Braun, T. J. Martínez, S. R. White, J. S. Moore and N. R. Sottos, Nature, 2009, 459, 68–72 CrossRef CAS PubMed
.
- M. D. Qian, Y. L. Sun, Z. Y. Hu, X. F. Fang, J. L. Zhu, X. Fan, Q. Liao, C. F. Wu and H. B. Sun, Mater. Horiz., 2020, 7, 1782–1789 RSC
.
- X. Le, H. Shang, H. Yan, J. Zhang, W. Lu, M. Liu, L. Wang, G. Lu, Q. Xue and T. Chen, Angew. Chem., Int. Ed., 2021, 60, 3640–3646 CrossRef CAS PubMed
.
- Y. Choi, H. J. Bae, A. C. Lee, H. Choi, D. Lee, T. Ryu, J. Hyun, S. Kim, H. Kim, S.-H. Song, K. Kim, W. Park and S. Kwon, Adv. Mater., 2020, 32, 2001249 CrossRef CAS PubMed
.
- Y. Yang, Q. Li, H. Zhang, H. Liu, X. Ji and B. Z. Tang, Adv. Mater., 2021, 33, 2105418 CrossRef CAS PubMed
.
Footnote |
† These authors contributed equally to this paper. |
|
This journal is © The Royal Society of Chemistry 2022 |
Click here to see how this site uses Cookies. View our privacy policy here.