A sustainable photochemical aerobic sulfide oxidation: access to sulforaphane and modafinil†
Received
9th June 2022
, Accepted 7th July 2022
First published on 8th July 2022
Abstract
Sulfoxide-containing molecules are an important class of compounds in the pharmaceutical industry and many efforts have been made to develop new and green protocols, targeting the chemoselective transformation of sulfides into sulfoxides. Photochemistry is a rapidly expanding research field employing light as the energy source. Photochemical aerobic processes possess additional advantages to photochemistry and may find applications in the chemical industries. Herein, a 370 nm catalyst-free aerobic protocol was developed, using 2-Me-THF as the green solvent. At the same time, two low-catalyst-loading anthraquinone-based processes (under a CFL lamp or 427 nm irradiation) in 2-Me-THF were developed. Furthermore, a broad range of substrates was tested. We also implemented our protocols towards the synthesis of the pharmaceutical active ingredients (APIs) sulforaphane and modafinil.
Introduction
Historically, sulfoxides comprise an important class of molecules in the pharmaceutical industry, beginning with the rise of antibacterial ingredients at the beginning of the 20th century.1 A number of sulfoxide-containing molecules are known to display an excellent pharmacological profile and are marketed as drugs, including omeprazole, lansoprazole, modafinil, sulforaphane, sulindac and fipronil (Scheme 1). Omeprazole and lansoprazole are proton-pump inhibitors (PPIs) and are widely used in pharmaceuticals treating gastric acid hypersecretion.2 Modafinil, another sulfoxide-containing drug, is used for the treatment of narcolepsy symptoms.3 Sulforaphane is a naturally occurring product, found in broccoli and Brussels sprouts, that has been proven to possess anti-cancer properties.4 Sulindac acts as a prodrug, which is metabolised in the human body to the corresponding sulfide, and it is well known as an anti-inflammatory and analgesic agent,5 while fipronil is an insecticide, used to protect crops against specific soil and foliar pests.6
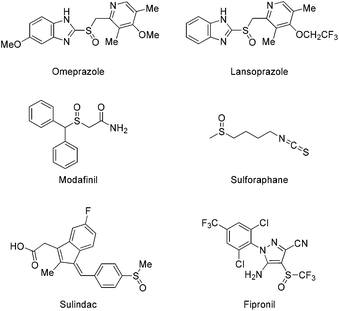 |
| Scheme 1 Selected examples of pharmaceuticals’ active ingredients or natural products containing the sulfoxide moiety. | |
Over the past years, many researchers worldwide, both in industry and in academia, have put great effort into developing a variety of industrially applicable oxidation methodologies, converting sulfides into the corresponding sulfoxides. In most of the protocols reported in the literature, hydrogen peroxide is the oxidant which holds the lion's share.7 The use of peroxides in general is highly beneficial due to their availability, low cost, and benign by-products. The major drawback of the use of peroxides appears to be their activation, which in most cases requires either heat or metal catalysts. Another widely used class of oxidants in the pharmaceutical industry is organic peracids,8 such as m-chloroperbenzoic acid (m-CPBA). The latter has been exploited in numerous sulfoxide syntheses,9 but is lacking chemoselectivity. In all the reported sulfoxide syntheses, over-stoichiometric amounts of oxidants are needed to increase the reaction yield. As a consequence, in many cases, sulfide overoxidation may occur, leading to sulfone by-products, which in the case of an active pharmaceutical ingredient (API) is restrictive.
In 2004, Avrutov and coworkers described the synthesis of Lansoprazole using tert-butyl hydroperoxide (TBHP) activated by a vanadium catalyst (Scheme 2A).10 In their patent application, the employed oxidation system was used to suppress the formation of the sulfone by-product.10 The use of peracids towards sulfide oxidation was extensively studied in the synthesis of Cephalosporins as well (Scheme 2B).11 The major drawback in their use, apart from the usual safety issues, is the need to purge the resulting organic acid, which in many cases is a difficult task in a manufacturing plant. To avoid this difficulty, inorganic oxidants were employed. In 2001, omeprazole was proposed to be produced using sodium percarbonate and a molybdenum catalyst (Scheme 2C).12
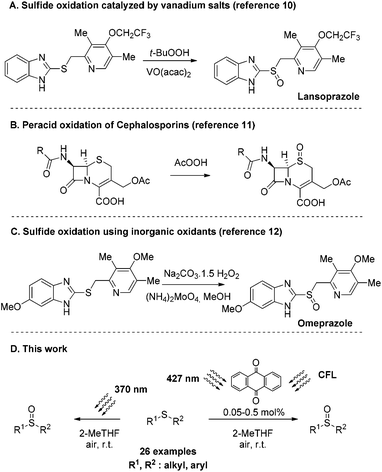 |
| Scheme 2 Sulfide oxidation in API synthesis. | |
Another mild oxidant that lately has been widely employed in oxidation protocols is oxygen.13 It is an abundant and ambient gas, considered the “greenest” option for oxidation reactions. Oxygen activation can occur via metal catalysis14 or organocatalysis.15 In 2020, Zografos and coworker proposed the use of proline-based organocatalysts for the aerobic oxidation of sulfides to sulfoxides, using HFIP as the solvent.16 However, expensive metal-based catalysts14 or expensive and hazardous solvents (e.g. HFIP)16 increase significantly the manufacturing cost, while the final purification of the product can be troublesome.
In 2008, MacMillan,17 Stephenson18 and Yoon19 relaunched an exciting and powerful area of research called photochemistry.20,21 This partially forgotten and rather misunderstood research field very quickly created new opportunities, unlocking many reactions not easily accessed using the conventional ground state pathways. The merger of photochemical protocols with aerobic (oxygen)-mediated oxidations was recognized quite early and the oxidation of sulfides to sulfoxides was first studied in the 1960s.22,23 Throughout the years, the photochemical aerobic oxidation of sulfides received some attention, but the use of specialized apparatus for irradiation or the use of heterogeneous and in some cases homogeneous catalysts (metal-based or organocatalysts) limited its applicability.23 However, since 2017, the emerging field of photochemistry merged with organocatalysis has drawn the interest of many researchers into studying photochemical aerobic sulfide oxidation.24
In our group over the past years, we have been focused on developing novel light-driven methodologies.25 Recently, we reported a mild, green and chemoselective direct photochemical aerobic oxidation of sulfides to the corresponding sulfoxides.26 Our experience in developing operationally simple and safe protocols easily applied in industrial scale productions27 led us to upgrade our methodology to a sustainable and industry-friendly protocol. Herein, we report environmental, novel, green and sustainable protocols for the oxidation of sulfides using a mild oxidant, such as molecular oxygen, in the presence of a low-cost commercially available photocatalyst. The reaction medium comprises the use of a biomass-based solvent, serving the basic principles of green chemistry, which is highly desirable in the chemical and pharmaceutical industries (Scheme 2D).
Results and discussion
In early 2022, we reported a general, mild and green protocol to convert sulfides into sulfoxides.26 Firstly, we explored the role of wavelength irradiation and proposed two low-catalyst-loading (0.05–0.5 mol%) anthraquinone-mediated methods (CFL lamps or 427 nm) and one photocatalyst-free protocol (370 nm) for the photochemical aerobic oxidation of sulfides into sulfoxides, having the advantage of short reaction times and no overoxidation reactions. Having in our minds the importance of this transformation in the pharmaceutical industry, we envisaged an expansion of those protocols to gain a more industrial and sustainable character. Initially, we turned our attention to the nature of the reaction medium, since the industrial character of any process is directly linked to the amount of waste produced (Table 1). We examined the anthraquinone-mediated reaction output of thioanisole (1a) oxidation under CFL irradiation in a variety of green solvents (Table 1).28 Protic solvents, i.e. isopropanol or glycerol, proved to be sluggish (Table 1, entries 1 and 2), while in water the reaction did not occur due to anthranquinone's insolubility (Table 1, entry 3). In solvents bearing a carbonyl group, i.e. ethyl acetate or γ-valerolactone (GVL), the reaction was sluggish, while trying to use a novel biobased solvent called Cyrene, the reaction did not take place (Table 1, entries 4–6). Turning to ethereal solvents, i.e. t-butyl methyl ether, afforded poor results (Table 1, entry 7), while 2-methyltetrahydrofuran (2-Me-THF) was the solvent that outperformed all the others (Table 1, entry 8). 2-Me-THF is a commercially available solvent, derived from furfural or levulinic acid, both products being easily derived from renewable lignocellulosic biomass.29 2-Me-THF has a strong chemical resemblance to tetrahydrofuran, but it features different physical properties. Its low miscibility with water and its good stability to acids and bases, compared with THF, are some of the advantages offered.30 Thus, its use in the pharmaceutical industry is highly appreciated. The addition of H2O (10 equiv.) was essential for the acceleration of the reaction rate and the suppression of the formation of the sulfone by-product (Table 1, entry 9 vs. entry 8).31 Due to hydrogen bond formation, water inhibits the conversion of sulfoxide to sulfones.32 In our previous study under LED irradiation (427 nm), photochemical aerobic sulfide oxidation led to the selective formation of the corresponding sulfoxide vs. sulfone after 5 h of irradiation in the presence of 0.05 mmol% of 4.26 When the reaction was performed in 2-Me-THF, the desired product was afforded in quantitative conversion, suppressing the undesired sulfone by-product, while the reaction time was reduced to 3 h (Table 1, entry 16). Apart from ethyl acetate, which afforded high sulfoxide conversion (Table 1, entry 13), all the other green solvents mentioned above afforded lower yields (Table 1, entries 10–15). Indeed, 2-Me-THF was the solvent of choice, reducing the reaction time at the same time, in comparison with our previous results.26
Table 1 Optimization of the reaction conditions for the photochemical aerobic oxidation of 1a, using a photocatalysta
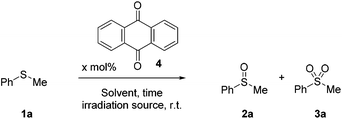
|
Entry |
Lamp (nm) |
Solvent, time (h) |
4 (mol%) |
1a b (%) |
2a/3a b (%) |
The reaction was performed with 1a (0.20 mmol) in solvent (1 mL), under open air irradiation for 18 h.
Conversion was determined by 1H-NMR.
The reaction was performed with the addition of H2O (40 μL).
0.5 mL of solvent was used instead of 1 mL.
|
1 |
CFL |
Glycerol, 18 |
0.5 |
75 |
25/0 |
2 |
CFL |
i-PrOH, 18 |
0.5 |
88 |
12/0 |
3 |
CFL |
H2O, 18 |
0.5 |
100 |
0/0 |
4 |
CFL |
EtOAc, 18 |
0.5 |
95 |
5/0 |
5 |
CFL |
GVL, 18 |
0.5 |
75 |
25/0 |
6 |
CFL |
Cyrene, 18 |
0.5 |
100 |
0/0 |
7 |
CFL |
MTBE, 18 |
0.5 |
86 |
14/0 |
8 |
CFL |
2-Me-THF, 18 |
0.5 |
0 |
70/30 |
9 c |
CFL
|
2-Me-THF, 18
|
0.5
|
0
|
99/1
|
|
10d |
427 |
Glycerol, 3 |
0.05 |
94 |
6/0 |
11d |
427 |
i-PrOH, 3 |
0.05 |
79 |
21/0 |
12d |
427 |
H2O, 3 |
0.05 |
91 |
9/0 |
13d |
427 |
EtOAc, 3 |
0.05 |
11 |
89/0 |
14d |
427 |
GVL, 3 |
0.05 |
61 |
39/0 |
15d |
427 |
Cyrene, 3 |
0.05 |
100 |
0/0 |
16 d |
427
|
2-Me-THF, 3
|
0.05
|
1
|
99/0
|
Next, we turned our attention to the catalyst-free protocol for the photochemical aerobic oxidation of 1a (Table 2). To our delight, when the reaction was performed under UVA-LED irradiation (370 nm) in 2-Me-THF, quantitative formation of the desired product was obtained after 2 h, under catalyst-free conditions (Table 2, entry 8). Again, this is a slightly decreased reaction time compared with our previous studies (2 h vs. 3 h).26 Quantitative formation of the desired sulfoxide was also obtained when GVL or water was used as the solvent (Table 2, entries 3 and 5); however, lower yields were obtained upon isolation (60% and 68%, respectively). Furthermore, GVL and water might pose difficulties when the catalyst-free protocol is used in the pharmaceutical industry. The use of GVL will add an extra purification step to the procedure, which raises the manufacturing cost, while the use of water as the reaction medium will narrow down the substrate scope, excluding intermediates bearing easily hydrolysed functional groups. Again, solvent optimization did not reveal any medium leading to better yields, compared with 2-Me-THF (Table 2, entries 1, 2, 4, 6, 7 vs. 8).
Table 2 Optimization of the reaction conditions for the photocatalyst-free photochemical aerobic oxidation of 1aa
Having found the optimum reaction conditions, we studied the substrate scope (Scheme 3). We probed all three approaches, using air as the sole oxidant. The first process is the catalyst-free approach, using irradiation at 370 nm in 2-Me-THF (45 W, protocol A). Also, the anthraquinone-mediated photochemical reaction, using a low catalyst loading (0.05 mol%) under 427 nm irradiation, was examined (45 W, protocol B). Finally, a CFL-mediated approach in 2-Me-THF was envisaged, using anthraquinone at 0.5 mol% (protocol C). Initially, we examined a variety of alkyl aryl sulfides, employing catalyst-free conditions upon irradiation at 370 nm. The oxidation step proceeded smoothly, affording the corresponding sulfoxides in good to excellent yields (Scheme 3). Along with thioanisole (1a), other phenyl-substituted sulfides having an alkyl moiety afforded the desired sulfoxides in high to quantitative yields (Scheme 3, protocol A, 2a–e). Aryl or substituted aryl sulfides with an alkyl group having various functional groups, i.e. double or triple bonds, hydroxy or ester moieties, afforded in all cases the desired products in good to excellent yields (Scheme 3, protocol A, 2f–j). Phenyl benzyl, naphthyl methyl, heteroaryl methyl, diaryl or fused aryl sulfides also afforded the corresponding sulfoxides in good to excellent yields (Scheme 3, protocol A, 2k–o). Additionally, NMR monitoring of the crude reaction mixture did not reveal any by-product (either from overoxidation or from the C–S fragmentation).
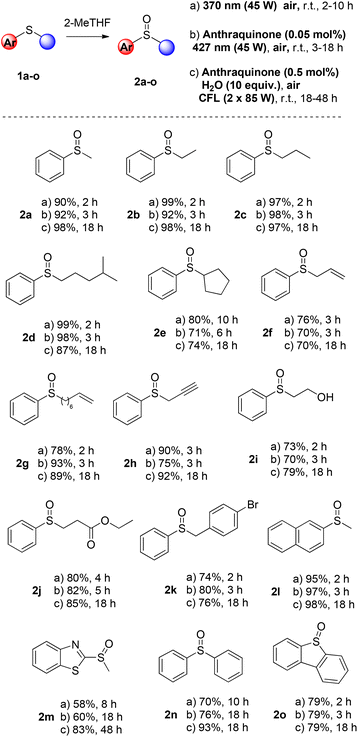 |
| Scheme 3 Substrate scope: aryl or heteroaryl substituted sulfides. | |
When the same substrates were subjected to LED irradiation (427 nm), the presence of a catalyst was required. The catalyst loading of 4 was 0.05 mol% and the reaction time varied from 3 to 18 h (Scheme 3, protocol B). When alkyl aryl sulfides were employed, the reaction time was usually 3 h, while good to excellent yields were obtained in all cases (Scheme 3, protocol B, 2a–o). Deviation in the reaction time was observed in the cases of phenyl secondary alkyl sulfide 1e, alkyl phenyl sulfide bearing an ester moiety (1j), heteroaryl methyl (1m) and diphenyl sulfide (1n). A prolonged reaction time was required (up to 18 h) for the reaction to take place. In the cases of sulfides 1f and 1h, no oxidation by-products on the double or the triple bond were detected. However, if a higher catalyst loading of 4 was employed, then a mixture of other oxidized products was obtained in both cases. Similarly, under the optimised reaction conditions, in the case of 1m, no other oxidation product was isolated. Next, we examined the substrate scope under CFL irradiation, increasing the catalyst loading (0.5 mol%) (Scheme 3, protocol C). Alkyl aryl sulfides afforded the corresponding sulfoxides in good to excellent yields in 18 h (Scheme 3, protocol C, 2a–e). Furthermore, functional groups, like double or triple bonds, hydroxy and ester moieties or aryl aryl sulfides were well tolerated (Scheme 3, protocol C, 2f–o).
To expand the industrial feasibility of our process, we also performed the photooxidation of thioanisole 1a, replacing the CFL lamps with sunlight (April 27, 2022, 10:00–14:00, Athens Greece, 37.97° N, 23.72° E, temperature range 23–25 °C), leading to a similarly excellent yield (97%) after 4 h. We also checked the scalability of our protocol, performing a gram-scale reaction (June 25, 2022, 09:00–17:00, Athens Greece, 37.97° N, 23.72° E, temperature range 28–35 °C), leading to an excellent yield (82%) after 8 h.31 Since the environmental conditions vary from time to time, we also performed aerobic photooxidation, applying our photocatalyst-free protocol and increasing the reaction scale (10 g). The reaction time was prolonged and 2 × LED 370 nm lamps were employed; however the formation of the corresponding sulfoxide was excellent (81% yield) after 13 h.31
Next, we examined our protocols in a broad range of dialkyl sulfides (Scheme 4). In this case (for the 370 nm protocol), prolonged reaction times were required (18 h) due to the reactivity of the substrates. Dialkyl sulfoxides were easily accessed in good to excellent yields when the photocatalyst-free aerobic oxidation protocol in 2-Me-THF was employed (Scheme 4, protocol A, 2p–x). The anthraquinone-mediated protocols were also applied to sulfide photooxidation, affording in all cases the corresponding sulfoxides in excellent yields. Upon CFL irradiation and using 0.5 mol% anthraquinone, the photooxidation reaction time was 18 h. Altering the irradiation source, using LED irradiation (427 nm), the amount of anthraquinone was 0.05 mol% and the oxidation reaction was completed after 3–5 h.
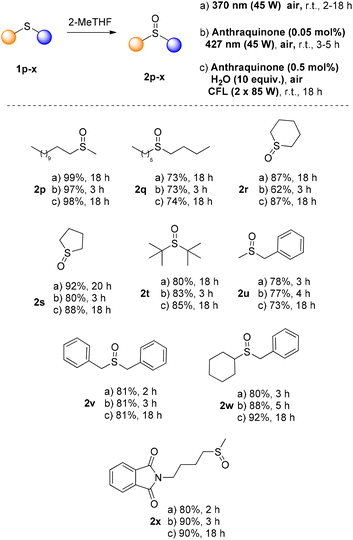 |
| Scheme 4 Substrate scope: dialkyl sulfides. | |
Synthetic application
Our desire to demonstrate the synthetic utility of our protocol in real-life applications led us to use our optimized reaction conditions in the oxidation of sulfide intermediates that appear in pharmaceutical active ingredients, i.e. sulforaphane and modafinil (Scheme 5). In 1992, Talalay and coworkers isolated sulforaphane [4-(methylsulfinyl)butylisothiocyanate] 6.33 Sulforaphane is the product of the enzymatic hydrolysis of glucophanin, which is found in the Brassicaceae family and in broccoli, in particular, and was recently found to exhibit anti-cancer properties.4 Recently, sulforaphane was also recognized as a potential inhibitor of the in vitro replication of SARS-CoV-2.34 Sulforaphane interacts synergistically with remdesivir, inhibiting coronavirus infection in vitro. We commenced our synthesis from intermediate 1x, which can be easily synthesized from 1,4-dibromobutane in two steps.35 Deprotection of the phthalimide group and subsequent treatment of the corresponding amine with carbon disulfide afforded isothio-cyanate 5, which is the most common intermediate for sulforaphane synthesis (Scheme 5, top).35 Applying our photochemical aerobic oxidation protocols in the oxidation of sulfide 5 afforded sulforaphane in good to excellent yields (Scheme 5, top). Contrary to all the reported methods described in the literature, where the oxidation was performed either using hydrogen peroxide or m-CPBA, the sulfone derivative was not detected. Applying our photocatalyst-mediated protocols, the reaction proceeded smoothly, affording sulforaphane in good to excellent yields. Under LED irradiation using (427 nm), sulforaphane was obtained in 96% in 5 h. One of the major issues in the preparation of sulforaphane is its decomposition under open air. We faced this problem when the photooxidation took place under household lamp irradiation, when we attained sulforaphane in a moderate yield (78%). To circumvent this problem in our catalyst-free conditions, we employed 0.5 mol% of anthraquinone (4) to minimize the reaction time, increasing the yield to 87%. To avoid purification by column chromatography, a liquid–liquid extraction–purification afforded sulforaphane in 80% yield (reaction performed at 370 nm).31 Thus, we believe this constitutes an even more industry-friendly approach for the synthesis of sulforaphane.
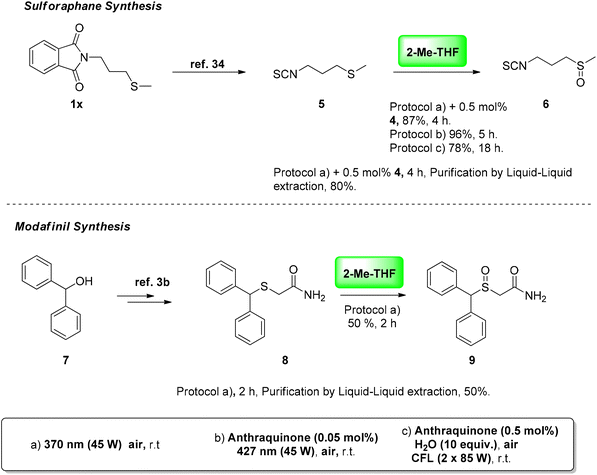 |
| Scheme 5 Synthetic application: late-stage photochemical aerobic oxidation for the synthesis of sulforaphane and modafinil. | |
Our initial intention in developing an industrially applicable photochemical protocol led us to search for more challenging substrate targets. For this reason, we questioned whether our photochemical protocols could be applied towards the synthesis of modafinil (Scheme 5, bottom). Modafinil 9 acts as a CNS stimulant, which promotes vigilance and wakefulness without any side effects.3b It is generally used for the treatment of narcolepsy and ADHD. As presented in the literature, sulfide 8 appears to be the most common intermediate for the synthesis of modafinil.3b Sulfide 8 contains an active benzylic position, which poses some difficulties in applying a chemoselective photochemical oxidation protocol. Indeed, when we examined the application of our photochemical protocols in the oxidation of sulfide 8, we observed that in both anthraquinone-mediated protocols the decomposition of sulfide 8 took place. Probably, the photocatalyst-mediated process initiates a C–S fragmentation in the highly reactive benzylic position. Careful monitoring of the reaction showed that the parasitic decomposition pathway is much faster than the oxidation one, since we did not observe any product formation. On the contrary, under catalyst-free conditions, the photochemical aerobic oxidation of sulfide 8 proceeded smoothly, affording the desired product in 50% yield. Similar to before, a liquid–liquid extraction–purification was also possible.
Mechanistic studies
The most intriguing part of photooxidation is understanding the reaction mechanism. In the literature, two different mechanistic pathways for photochemical aerobic sulfide oxidation are reported.23 In the first mechanism singlet oxygen, 1O2, is generated using a photosensitizer (Scheme 6). Singlet oxygen performs the oxygenation of the sulfide, leading to persulfoxide intermediate I (Scheme 6). This intermediate reacts with another sulfide, affording two molecules of the desired sulfoxide. In the second mechanistic pathway, a single electron transfer (SET) occurs between the excited photocatalyst and the sulfide, affording sulfide radical cation II (Scheme 6). Reaction with either the superoxide radical anion or triplet state oxygen 3O2 leads to the sulfoxide. In our work, the use of different irradiation sources in our protocols, along with the use of alkyl aryl, aryl aryl or dialkyl sulfides, may suggest that a different reaction mechanism may be in place in each case. In general, anthraquinone (4a) is known from the literature to be able to generate singlet oxygen via energy transfer.36 In our previous studies, we performed a detailed study of the reaction mechanism.26 Utilizing fluorescence quenching studies and the appropriate quenchers shed light on the reaction mechanism.26 In the photocatalyst-free process (370 nm), via the appropriate quenchers, we identified that dodecyl methyl sulfide (dialkyl sulfides) are oxidized via singlet oxygen (Scheme 6C), while when anthraquinone is employed (427 nm or CFL), the singlet oxygen pathway is dominant (Scheme 6A). In the case of thioanisole (alkyl aryl sulfides), in the photocatalyst-free protocol (370 nm), the singlet oxygen mechanism is in place (Scheme 6C). Under the use of anthraquinone (427 nm or CFL), the singlet oxygen pathway generated by anthraquinone is followed (Scheme 6A). Finally, in the case of diphenyl sulfide (aryl aryl sulfides), in the photocatalyst-free protocol (370 nm), the sulfide radical cation pathway is in place (Scheme 6D). With the use of anthraquinone (427 nm or CFL), the sulfide radical cation is produced (Scheme 6B).
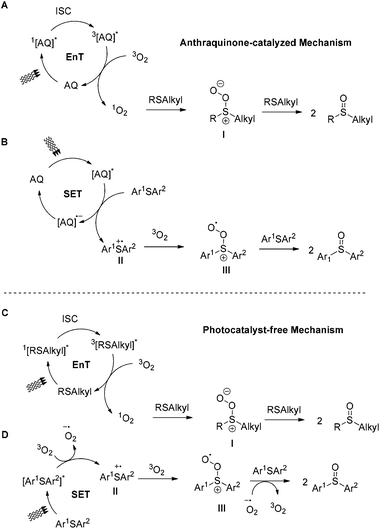 |
| Scheme 6 Proposed mechanistic pathways for the photochemical aerobic oxidation of sulfides. | |
Conclusions
The basic principles of green chemistry have been known since 1990, but real application in the pharmaceutical industry is rather limited. During the last decade, the photochemical aerobic oxidation of sulfides to sulfoxides has attracted exponential amounts of attention. Herein, we married our previously developed photochemical oxidation protocols with the basic principles of green chemistry, providing a general, fast, mild, green and easy-to-operate industry-friendly procedure to perform these reactions. We exploited 2-Me-THF, a commercially available green solvent, derived from furfural or levulinic acid, both products being easily derived from renewable lignocellulosic biomass, in our low-catalyst-loading (0.05–0.5 mol%) anthraquinone-mediated protocols (CFL lamps or 427 nm) and in our photocatalyst-free aerobic protocol (370 nm). Among the different protocols developed, the photocatalyst-free aerobic protocol (370 nm) appears to be the most efficient, since it is fast, it can be scaled up and it can be easily applied to more challenging substrates bearing easily oxidized C–H bonds; see the case of modafinil. However, the 427 nm protocol can be employed in cases where organic compounds bear chromophores below 400 nm, since in this case, they do not interfere with the oxidation process. Finally, the cheapest alternative is the CFL-mediated procedure. A broad range of substrates was successfully tested under the optimum conditions. In some cases, the reaction proved to be faster than those performed in common organic solvents. We also applied our photooxidation protocols towards the synthesis of two APIs: sulforaphane, a promising anti-cancer agent and a potential SARS-CoV-2 inhibitor, and modafinil, a drug used to treat narcolepsy symptoms. We sincerely hope these processes can find application in real life.
Author contributions
C. G. K. conceived the study, E. S., P. L. G. and C. G. K. designed the experiments and analyzed the data. E. S. and P. L. G. performed the experiments. P. L. G. and C. G. K. prepared the draft of the manuscript and C. G. K. performed the final editing. The manuscript was written through contributions from all authors.
Conflicts of interest
There are no conflicts to declare.
Acknowledgements
The authors gratefully acknowledge the Hellenic Foundation for Research and Innovation (HFRI), since this research project was supported by the Hellenic Foundation for Research and Innovation (H.F.R.I.) under the “1st Call for H.F.R.I. Research Projects to support Faculty Members & Researchers and the Procurement of High-cost research equipment grant” (grant number 655).
Notes and references
-
(a) M. Mitchard, Drug Metab. Drug Interact., 1988, 6, 183–202 CAS;
(b) E. A. Ilardi, E. Vidaku and J. T. Njardarson, J. Med. Chem., 2014, 57, 2832–2842 CrossRef CAS PubMed;
(c) M. Feng, B. Tang, S. H. Liang and X. Jiang, Curr. Top. Med. Chem., 2016, 16, 1200–1216 CrossRef CAS PubMed;
(d) R. A. Hartz, A. G. Arvanitis, C. Arnold, J. P. Rescinito, K. L. Hung, G. Zhang, H. Wong, D. R. Langley, P. J. Gilligan and G. L. Trainor, Bioorg. Med. Chem. Lett., 2006, 16, 934–937 CrossRef CAS PubMed.
-
(a) D. J. Hetzel, W. D. Reed, F. M. Narielvala, M. Mackinnon, J. H. MacCarthy, B. Mitchell, B. R. Beveridge, B. H. Laurence and G. G. Gibson, Gastroenterology, 1998, 95, 903–912 CrossRef;
(b) L. B. Barradell, D. Faulds and D. McTavish, Drugs, 1992, 44, 225–250 CrossRef CAS PubMed.
-
(a) C. A. Czeisler, J. K. Walsh, T. Roth, R. J. Hughes, K. P. Wright, L. Kingsbury, S. Arora, J. R. L. Schwartz, G. E. Niebler and D. F. Dinges, N. Engl. J. Med., 2005, 353, 476–486 CrossRef CAS PubMed;
(b) T. Prisinzano, J. Podobinski, K. Tidgewell, M. Luo and D. Swenson, Tetrahedron: Asymmetry, 2004, 15, 1053–1058 CrossRef CAS.
-
(a) N. Juge, R. F. Mithen and M. Traka, Cell. Mol. Life Sci., 2007, 64, 1105–1127 CrossRef CAS PubMed;
(b) M. G. Kokotou, P. K. Revelou, C. Pappas and V. Constantinou-Kokotou, Food Chem., 2017, 237, 566–573 CrossRef CAS PubMed.
- R. N. Brogden, R. C. Heel, T. M. Speight and G. S. Avery, Drugs, 1978, 16, 97–114 CrossRef CAS PubMed.
- D. Hainzl, L. M. Cole and J. E. Casida, Chem. Res. Toxicol., 1998, 11, 1529–1535 Search PubMed.
- For a review, see:
(a) I. Triandafillidi, D. I. Tzaras and C. G. Kokotos, ChemCatChem, 2018, 10, 2521–2535 Search PubMed. For a recent contribution on the oxidation of sulfides, see:
(b) E. Voutyritsa, I. Triandafillidi and C. G. Kokotos, Synthesis, 2017, 49, 917–924 CAS.
-
(a)
A. E. Braendstroem, WO9118895, 1991;
(b)
R. L. M. Broeckx, D. De Smaele and S. M. H. Leurs, WO2003008406, 2003.
-
(a) N. K. Jana and J. G. Verkade, Org. Lett., 2003, 5, 3787–3790 CrossRef CAS PubMed;
(b) R. J. Griffin, A. Henderson, N. J. Curtin, A. Echalier, J. A. Endicott, I. R. Hardcastle, D. R. Newell, N. E. Noble, L. Z. Wang and T. B. Golding, J. Am. Chem. Soc., 2006, 128, 6012–6013 CrossRef CAS PubMed.
-
I. Avrutov, M. Mendelovici and N. Finkelstein, U.S. Patent, US2004138466, 2004 Search PubMed.
- E. Bernasconi, J. Lee, J. Rolleto, L. Sogli and B. Walker, Org. Process Res. Dev., 2002, 6, 152–157 CrossRef CAS.
-
M. R. Berenguer, P. J. Campon and L. Coppi, WO2001068594, 2001.
- X. Zhang, K. P. Rakesh, L. Ravindar and H.-L. Qin, Green Chem., 2018, 20, 4790–4833 RSC.
- Z. Shi, C. Zhang, C. Tang and N. Jiao, Chem. Soc. Rev., 2012, 41, 3381–3430 RSC.
-
(a) R. V. Ottenbacher, E. P. Talsi and K. P. Bryliakov, Russ. Chem. Rev., 2018, 87, 821–830 CrossRef CAS;
(b) S. P. Brown, M. P. Brochu, C. J. Sinz and D. W. C. MacMillan, J. Am. Chem. Soc., 2003, 125, 10808–10809 CrossRef CAS PubMed.
- M. Petsi and A. L. Zografos, ACS Catal., 2020, 10, 7093–7099 CrossRef CAS.
- D. A. Nicewicz and D. W. C. MacMillan, Science, 2008, 322, 77–80 CrossRef CAS PubMed.
- J. M. R. Narayanan, J. W. Tucker and C. R. J. Stephenson, J. Am. Chem. Soc., 2009, 131, 8756–8757 CrossRef PubMed.
- M. A. Ischay, M. E. Anzovino, J. Du and T. P. Yoon, J. Am. Chem. Soc., 2008, 130, 12886–12887 CrossRef CAS PubMed.
- For selected reviews, see:
(a) K. L. Skubi, T. R. Blum and T. P. Yoon, Chem. Rev., 2016, 116, 10035–10074 CrossRef CAS PubMed;
(b) N. A. Romero and D. A. Nicewicz, Chem. Rev., 2016, 116, 10075–10166 CrossRef CAS PubMed;
(c) D. Cambié, C. Bottecchia, N. J. W. Straathof, V. Hessel and T. Noël, Chem. Rev., 2016, 116, 10276–10341 CrossRef PubMed;
(d) M. N. Hopkinson, A. Tlahuext-Aca and F. Glorius, Acc. Chem. Res., 2016, 49, 2261–2272 CrossRef CAS PubMed;
(e) J. Twilton, C. Le, P. Zhang, M. H. Shaw, R. W. Evans and D. W. C. MacMillan, Nat. Rev. Chem., 2017, 1, 0052 CrossRef CAS;
(f)
C. R. J. Stephenson, T. P. Yoon and D. W. C. MacMillan, Visible Light Photocatalysis in Organic Chemistry, Wiley, Weinheim, 2018 CrossRef;
(g) J. Schwarz and B. König, Green Chem., 2018, 20, 323–361 RSC;
(h) I. K. Sideri, E. Voutyritsa and C. G. Kokotos, Org. Biomol. Chem., 2018, 16, 4596–4614 RSC;
(i) M. Silvi and P. Melchiorre, Nature, 2018, 554, 41–49 CrossRef CAS PubMed;
(j) S. Reischauer and B. Pieber, iScience, 2021, 24, 102209–102224 CrossRef CAS PubMed;
(k) N. F. Nikitas, P. L. Gkizis and C. G. Kokotos, Org. Biomol. Chem., 2021, 19, 5237–5253 RSC.
- For selected examples, see:
(a) T. P. Yoon, M. A. Ischay and J. Du, Nature, 2010, 2, 527–532 CAS;
(b) R. Brimioulle and T. Bach, Science, 2013, 342, 840–843 CrossRef CAS PubMed;
(c) Y. Y. Loh, K. Nagao, A. J. Hoover, D. Hesk, N. R. Rivera, S. L. Colletti, I. W. Davies and D. W. C. MacMillan, Science, 2017, 358, 1182–1187 CrossRef CAS PubMed;
(d) A. Fawcett, J. Pradeilles, Y. Wang, T. Mutsuga, E. L. Myers and V. K. Aggarwal, Science, 2017, 357, 283–286 CrossRef CAS PubMed;
(e) A. Trowbridge, D. Reich and M. J. Gaunt, Nature, 2018, 561, 522–527 CrossRef CAS PubMed;
(f) E. Speckmeier, T. Fische and K. A. Zeitler, J. Am. Chem. Soc., 2018, 140, 15353–15365 CrossRef CAS PubMed;
(g) S. P. Morcillo, E. D. Dauncey, J. H. Kim, J. J. Douglas, N. S. Sheikh and D. Leonori, Angew. Chem., Int. Ed., 2018, 57, 12945–12949 CrossRef CAS PubMed;
(h) J. Wu, P. S. Grant, X. Li, A. Noble and V. K. Aggarwal, Angew. Chem., Int. Ed., 2019, 58, 5697–5701 CrossRef CAS PubMed;
(i) T. Patra, S. Mukherjee, J. Ma, F. Strieth-Kalthoff and F. Glorius, Angew. Chem., Int. Ed., 2019, 58, 10514–10520 CrossRef CAS PubMed;
(j) A. Ruffoni, F. Julia, T. D. Svejstrup, A. J. McMillan, J. J. Douglas and D. Leonori, Nat. Chem., 2019, 11, 426–433 CrossRef CAS PubMed.
- For the early examples on photochemical aerobic oxidation of sulfides, see:
(a) G. O. Schenck and C. H. Krauch, Chem. Ber., 1963, 96, 517–519 CrossRef CAS;
(b)
K. Gollnick, in Advances in Photochemistry, ed. W. A. Noyes Jr., G. S. Hammond and J. N. Pitts Jr., John Wiley & Sons, Inc., 1968, vol. 6, pp. 109–110 Search PubMed;
(c) C. S. Foote and J. W. Peters, J. Am. Chem. Soc., 1971, 93, 3795–3796 CrossRef CAS.
- For a recent review, see: E. Skolia, P. L. Gkizis and C. G. Kokotos, ChemPlusChem, 2022, 87 CrossRef CAS PubMed , e202200008.
-
(a) S. M. Bonesi, S. Crespi, D. Merli, I. Manet and A. Albini, J. Org. Chem., 2017, 82, 9054–9065 CrossRef CAS PubMed;
(b) C. Ye, Y. Zhang, A. Ding, Y. Hu and H. Guo, Sci. Rep., 2018, 8, 2205–2210 CrossRef PubMed;
(c) J. Li, W. Bao, Z. Tang, B. Guo, S. Zhang, H. Liu, S. Huang, Y. Zhang and Y. Rao, Green Chem., 2019, 21, 6073–6081 RSC;
(d) Y. Li, S. A. e.-A. Rizvi, D. Hu, D. Sun, A. Gao, Y. Zhou, J. Li and X. Jiang, Angew. Chem., Int. Ed., 2019, 58, 13499–13506 CrossRef CAS PubMed;
(e) Y. Zhang, J. Lou, M. Li, Z. Yuan and Y. Rao, RSC Adv., 2020, 10, 19747–19750 RSC;
(f) K.-J. Liu, Z. Wang, L.-H. Lu, J.-Y. Chen, F. Zeng, Y.-W. Lin, Z. Cao, X. Yu and W.-M. He, Green Chem., 2021, 23, 496–500 RSC;
(g) Q. Fan, L. Zhu, X. Li, H. Ren, G. Wu, H. Zhu and W. Sun, Green Chem., 2021, 23, 7945–7949 RSC.
- For contributions employing metal complexes in photoredox reactions, see:
(a) I. Triandafillidi, M. G. Kokotou and C. G. Kokotos, Org. Lett., 2018, 20, 36–39 CrossRef CAS PubMed. For contributions employing organic molecules in photochemical reactions, see:
(b) G. N. Papadopoulos, D. Limnios and C. G. Kokotos, Chem. – Eur. J., 2014, 20, 13811–13814 CrossRef CAS PubMed;
(c) N. Kaplaneris, A. Bisticha, G. N. Papadopoulos, D. Limnios and C. G. Kokotos, Green Chem., 2017, 19, 4451–4456 RSC;
(d) N. F. Nikitas, I. Triandafillidi and C. G. Kokotos, Green Chem., 2019, 21, 669–674 RSC;
(e) E. Voutyritsa and C. G. Kokotos, Angew. Chem., Int. Ed., 2020, 59, 1735–1741 CrossRef CAS PubMed;
(f) G. N. Papadopoulos, M. G. Kokotou, N. Spiliopoulou, N. F. Nikitas, E. Voutyritsa, D. I. Tzaras, N. Kaplaneris and C. G. Kokotos, ChemSusChem, 2020, 13, 5934–5944 CrossRef CAS PubMed;
(g) N. F. Nikitas, D. I. Tzaras, I. Triandafillidi and C. G. Kokotos, Green Chem., 2020, 22, 471–477 RSC;
(h) N. Spiliopoulou and C. G. Kokotos, Green Chem., 2021, 23, 546–551 RSC;
(i) C. S. Batsika, C. Mantzourani, D. Gkikas, M. G. Kokotou, O. G. Mountanea, C. G. Kokotos, P. K. Politis and G. Kokotos, J. Med. Chem., 2021, 64, 5654–5666 CrossRef CAS PubMed;
(j) N. F. Nikitas, M. K. Apostolopoulou, E. Skolia, A. Tsoukaki and C. G. Kokotos, Chem. – Eur. J., 2021, 27, 7915–7922 CrossRef CAS PubMed;
(k) I. Triandafillidi, N. F. Nikitas, P. L. Gkizis, N. Spiliopoulou and C. G. Kokotos, ChemSusChem, 2022, 15 CrossRef CAS PubMed , e202102441.
- E. Skolia, P. L. Gkizis, N. F. Nikitas and C. G. Kokotos, Green Chem., 2022, 24, 4108–4118 RSC.
-
(a) S. A. Zisopoulou, A. E. Pafili, P. Gkizis, T. Andreou, T. V. Koftis, A. Lithadioti, E. Neokosmidis and J. K. Gallos, Synthesis, 2020, 52, 2662–2666 CrossRef CAS;
(b) C. I. Stathakis, P. L. Gkizis, E. S. Alexandraki, S. Trakossas, M. Terzidis, E. Neokosmidis, C. K. Zacharis, C. Vasiliadou, E. Vastardi, T. Andreou, A. Zitrou, A.-A. Varvogli and T. V. Koftis, Org. Process Res. Dev., 2017, 21, 1413–1418 CrossRef CAS.
- S. Kar, H. Sanderson, K. Roy, E. Benfenati and J. Leszczynski, Chem. Rev., 2022, 122, 3637–3710 CrossRef PubMed.
- A. Corma, S. Iborra and A. Velty, Chem. Rev., 2007, 107, 2411–2502 CrossRef CAS PubMed.
- V. Pace, P. Hoyos, L. Castoldi, P. Dominguez de Maria and A. R. Alcantara, ChemSusChem, 2012, 5, 1369–1379 CrossRef CAS PubMed.
- For detailed experimental procedures, optimization studies and mechanistic experiments, see the ESI.†.
- P. Pitchen, E. Dunach, M. N. Deshmukh and H. B. Kagan, J. Am. Chem. Soc., 1984, 106, 8188–8193 CrossRef CAS.
- Y. Zhang, P. Talalay, C. Cho and G. H. Pooner, Proc. Natl. Acad. Sci. U. S. A., 1992, 89, 2399–2403 CrossRef CAS PubMed.
- A. A. Ordonez, C. K. Kullen, A. F. Villabone-Rueda, E. A. Thompson, M. L. Turner, V. F. Merino, Y. Yan, J. Kim, S. L. Davis, O. Komm, J. D. Powell, F. R. D'Alessio, R. H. Yolken, S. K. Jain and L. Jones-Brando, Commun. Biol., 2022, 5, 242–255 CrossRef CAS PubMed.
- X. Chen, Z. Li, X. Sun, H. Ma, X. Chen, J. Ren and K. Hu, Synthesis, 2011, 3991–3996 CrossRef CAS.
-
(a) S. C. Núñez Montoya, L. R. Comini, M. Sarmiento, C. Becerra, I. Albesa, G. A. Arguello and J. L. Cabera, J. Photochem. Photobiol., B, 2005, 78, 77–83 CrossRef PubMed;
(b) N. Liu and G. Sun, Ind. Eng. Chem. Res., 2011, 50, 5326–5333 CrossRef CAS.
Footnotes |
† Electronic supplementary information (ESI) available: Experimental data, optimization studies and 1H and 13C NMR data. See DOI: https://doi.org/10.1039/d2ob01066f |
‡ Denotes equal contribution. |
|
This journal is © The Royal Society of Chemistry 2022 |
Click here to see how this site uses Cookies. View our privacy policy here.