Discovery of varlaxins, new aeruginosin-type inhibitors of human trypsins†
Received
21st December 2021
, Accepted 3rd March 2022
First published on 9th March 2022
Abstract
Low-molecular weight natural products display vast structural diversity and have played a key role in the development of novel therapeutics. Here we report the discovery of novel members of the aeruginosin family of natural products, which we named varlaxins. The chemical structures of varlaxins 1046A and 1022A were determined using a combination of mass spectrometry, analysis of one- and two-dimensional NMR spectra, and HPLC analysis of Marfey's derivatives. These analyses revealed that varlaxins 1046A and 1022A are composed of the following moieties: 2-O-methylglyceric acid 3-O-sulfate, isoleucine, 2-carboxy-6-hydroxyoctahydroindole (Choi), and a terminal arginine derivative. Varlaxins 1046A and 1022A differ in the cyclization of this arginine moiety. Interestingly, an unusual α-D-glucopyranose moiety derivatized with two 4-hydroxyphenylacetic acid residues was bound to Choi, a structure not previously reported for other members of the aeruginosin family. We sequenced the complete genome of Nostoc sp. UHCC 0870 and identified the putative 36 kb varlaxin biosynthetic gene cluster. Bioinformatics analysis confirmed that varlaxins belong to the aeruginosin family of natural products. Varlaxins 1046A and 1022A strongly inhibited the three human trypsin isoenzymes with IC50 of 0.62–3.6 nM and 97–230 nM, respectively, including a prometastatic trypsin-3, which is a therapeutically relevant target in several types of cancer. These results substantially broaden the genetic and chemical diversity of the aeruginosin family and provide evidence that the aeruginosin family is a source of strong inhibitors of human serine proteases.
Introduction
Advanced solid cancers are often difficult to treat because of the ability of tumour cells to escape from the primary tumour and form distant metastases.1 Proteases play a critical role in tumour progression, especially in the process of metastatic dissemination of cancer cells and the promotion of tumour growth.2,3 Trypsin is one of the best-characterized proteolytic enzymes.4,5 The PRSS1, PRSS2 and PRSS3 genes encode trypsinogen-1, -2 and -3 trypsin precursors, respectively, which are especially abundant in pancreatic secretions.5 These precursors can be proteolytically activated (e.g., by enteropeptidase) to trypsin-1, -2, and -3 (also known as cationic and anionic trypsin, and mesotrypsin, respectively). Trypsin-3 is an enigmatic minor component of pancreatic secretions with a proposed role of modulating overall trypsin activity, as it efficiently degrades proteinaceous trypsin inhibitors.6,7 Elevated levels of trypsin-3 are associated with several human diseases. Trypsin-3 has also been identified as a potential therapeutic target for the reduction of tumour growth and metastasis in several cancers, such as prostate, breast and pancreatic cancer.8–12 However, the high sequence identity between trypsins extends to the active sites of the enzymes and makes selective targeting of trypsin-3 challenging.7,13–16 Nevertheless, human trypsin isoenzymes have slight differences in substrate specificity, which suggests that the development of selective trypsin inhibitors is possible.17
Aeruginosins are a group of tetrapeptides produced by cyanobacteria.18 They often possess potent inhibitory activity on trypsin-type serine proteases and, thus, represent potential lead molecules for pharmaceutical applications.18 This class of non-ribosomal peptides was originally discovered in Microcystis aeruginosa.18,19 Aeruginosins have also been identified in other cyanobacterial genera, such as Nodularia, Planktothrix, and Nostoc. Aeruginosin subgroups include pseudoaeruginosins,20 nostosins,21 suomilide,22,23 and banyasides.24 They have a complex chemical structure and typically contain the non-proteogenic 2-carboxy-6-hydroxyoctahydroindole (Choi) moiety and the C-terminal arginine derivatives argininal, argininol, agmatine, 1-amidino-2-ethoxy-3-aminopiperidine, and more rarely a 1-amino-2-(N-amidino-Δ3-pyrrolinyl)-ethyl (Aaep) moiety (Fig. S1–S3†). Aeruginosins are sometimes decorated with sugars, which themselves may be further derivatized with fatty acids.22,24,25 Aeruginosins are the products of a highly variable non-ribosomal peptide synthetase (NRPS) biosynthetic pathway.23,26–31 The diversity of the aeruginosin family is quite broad and over 130 variants have been described to date (34, Table S1†). However, the chemical diversity of the aeruginosin family of natural products is underestimated given the known genetic diversity of this family.28,33,34
Members of the aeruginosin natural product family are often strong serine protease inhibitors.18 It is noteworthy that all the previously reported aeruginosin groups contain well-known trypsin inhibitors.18,20–24 The most potent microbial trypsin inhibitor reported from this family of natural products to date is chlorodysinosin A with an IC50 of 37 nM (Fig. S3 and Table S2†). Most reported biochemical assays employ nonhuman trypsin, such as porcine and bovine trypsin. However, the primary structures of porcine and bovine trypsins differ significantly from those of human trypsins.35 Thus far, there has been only one study that examined aeruginosins (suomilide) as human trypsin inhibitors.23 Notably, suomilide was also found to inhibit invasion of trypsin-3 expressing prostate cancer cells.23 Here we report the discovery of two new members of the aeruginosin family of natural products, varlaxins 1022A and 1046A, that inhibit human trypsin isoenzymes in sub-nanomolar concentrations.
Experimental
Discovery of varlaxins
Varlaxins were discovered serendipitously from Nostoc sp. UHCC 0870 during routine screening of strains of the UHCC culture collection for the production of secondary metabolites. Varlaxins were subsequently identified from Nostoc spp. UHCC 0840, UHCC 0817, UHCC 0758 and UHCC 0757 (Table 1). Nostoc spp. UHCC 0840 and 0870 were collected 2014 from Varlaxudden, Porvoo from the brackish Gulf of Finland waterfront (N 60°12.225′, E 025°37.95′) and isolated into pure culture. Nostoc sp. UHCC 0817 was collected in 2012 from Kobben, Hanko, Finland from the rock surface of Gulf of Finland waterfront (N 59°49.92′, E 023°5.20′). Nostoc sp. UHCC 0758 was collected in 2003 from a mixture of gastropods collected from marina of Jurmo island, Finland (N 59°49.62′, E 021°35.05′). Nostoc sp. UHCC 0757 was collected from an undescribed location in Finland. Strains were grown for 3 weeks at ambient temperature (20–22 °C) under continuous photon irradiance of 15 μmol photons per m2 per s in 40 mL of saline Z8 medium36 lacking a source of combined nitrogen. Biomass was collected by centrifugation at 7000g for 7 min. The pellets were freeze-dried with a BETA 2–8 LSC plus freeze dryer with a LYO CUBE 4–8 chamber (Christ). Freeze-dried pellets were extracted with 1 mL of MeOH in 2 mL plastic tubes containing 0.5 mm glass beads by shaking with a FastPrep cell disrupter (Thermo Electron Corporation, Qbiogene, Inc.) for 30 s at a speed of 6.5 m s−1. The extract was centrifuged at 10
000g for 5 min, and the supernatant was analysed with a high-resolution UPLC-QTOF (Acquity I-Class UPLC-Synapt G2-Si, Waters Corp., Milford, MA) system. A 1 μl aliquot was injected into a Kinetex C8 column (50 × 2 mm, 1.7 μm, Phenomenex Inc.), which was eluted at 40 °C with a flow rate of 0.3 mL min−1 from 5% of solvent B (acetonitrile or 1
:
1 acetonitrile/isopropanol with formic acid added to 0.1%) in 0.1% formic acid (solvent A) to 95% of B in 5 min, held for 2 min, then back to 5% of B in 0.5 min, and finally kept for 2.5 min before the next run. In MS/MS mode, the column was eluted from 5% of solvent B (1
:
1 acetonitrile/isopropanol with formic acid added to 0.1%) in 0.1% formic acid (solvent A) to 60% of B in 10 min, then in 0.01 min to 100% of B, held for 3.99 min, then back to 5% of B in 0.5 min, and finally kept for 5.5 min before the next run. QTOF was calibrated using sodium formate and Ultramark® 1621, which yielded a calibrated mass range from m/z 91 to 1921. Leucine Enkephalin was used at 10 s intervals as a lock mass reference compound. Data were collected in positive electrospray ionization Resolution Mode at scan range of m/z 50–2000. In MS/MS mode, parameters were same as in MS mode and Trap Collision Energy Ramp proceeded from 40.0 eV to 80.0 eV.
Table 1 Varlaxin variants identified from five strains in the UHCC culture collection. Mgs = 2-O-methylglyceric acid 3-O-sulfate, Choi = 2-carboxy-6-octahydroindole, Agma = 4-amidinobutylamide, Aaep = 1-amidino-3-(2-aminoethyl)-3-pyrroline, Hpaa = 2-(4-hydroxyphenyl)acetic acid. Δ = difference in parts per million (ppm) between calculated and experimental [M + Na]+. RI = percentage relative intensities of varlaxin variants in a strain. [M − 80 + H]+ = desulfated (80 = SO3) protonated ion
No |
Vara |
t
R (min) |
[M − 80 + H]+ |
[M + Na]+ |
Structural units |
UHCC 0870 |
UHCC 0840 |
UHCC 0817 |
UHCC 0758 |
UHCC 0757 |
Core |
Side group |
[M + Na]+ |
[M − 80 + H]+ |
[M + Na]+ |
[M − 80 + H]+ |
[M + Na]+ |
[M − 80 + H]+ |
[M + Na]+ |
[M − 80 + H]+ |
[M + Na]+ |
[M − 80 + H]+ |
Calculated (m/z)b |
1 |
2c |
3 |
4 |
Sugard |
Hpaa |
Δ (ppm) |
RI (%) |
Δ (ppm) |
RI (%) |
Δ (ppm) |
RI (%) |
Δ (ppm) |
RI (%) |
Δ (ppm) |
RI (%) |
Numbering base to exact mass of the neutral molecule. Bold font = product ion spectrum annotated.
M − 80 originates from neutral loss of SO3 from M.
According to aa analysis of 1022A and 1046A aa is Ile. In other variants could also be isobaric Leu.
Analyzed from 1022A/1046A, Hpaa = number of Hpaa (4-hydroxyphenylacetic acid) units.
From [M − 80 + H]+.
|
1 |
740 |
1.00 |
661.3767 |
763.3154 |
Mgs |
Val |
Choi |
Agma |
D-Glc |
— |
|
|
|
|
−2.3e |
0.1 |
−0.4 |
0.1 |
0.9 |
0.4 |
2 |
764
|
1.04 |
685.3767
|
787.3154 |
Mgs |
Val |
Choi |
Aaep |
D-Glc |
— |
|
|
|
|
−0.9e |
1.5 |
−0.7
|
1.8
|
−0.5 |
0.5 |
3 |
754
|
1.24 |
675.3923
|
777.3311 |
Mgs |
Ile |
Choi |
Agma |
D-Glc |
— |
−3.5 |
0.05 |
7.7 |
0.04 |
−2.4 |
0.3 |
−0.7
|
2.0
|
1.6 |
5.6 |
4 |
778
|
1.30 |
699.3923
|
801.3311 |
Mgs |
Ile |
Choi |
Aaep |
D-Glc |
— |
−1.5 |
0.1 |
−3.3 |
0.2 |
0.1 |
10.5 |
0.8
|
39
|
2.2 |
7.9 |
5 |
874A |
1.56 |
795.4135 |
897.3522 |
Mgs |
Val |
Choi |
Agma |
D-Glc |
1 |
−1.9 |
0.02 |
|
|
−1.2 |
0.1 |
−2.5 |
0.02 |
−3.5 |
0.02 |
6 |
898A |
1.61 |
819.4135 |
921.3522 |
Mgs |
Val |
Choi |
Aaep |
D-Glc |
1 |
−0.7 |
0.05 |
|
|
−1.3 |
1.9 |
−0.7 |
0.4 |
−3.3 |
0.1 |
7 |
888A |
1.64 |
809.4291 |
911.3679 |
Mgs |
Ile |
Choi |
Agma |
D-Glc |
1 |
−3.0 |
0.3 |
|
|
−1.7 |
0.3 |
−1.8 |
0.4 |
−0.7 |
0.4 |
8 |
912A
|
1.67 |
833.4291
|
935.3679 |
Mgs |
Ile |
Choi |
Aaep |
D-Glc |
1 |
−1.6 |
1.1 |
−6.3 |
0.2 |
−0.1 |
11.9 |
0.4
|
12
|
0.8 |
1.9 |
9 |
874B |
1.69 |
795.4135 |
897.3522 |
Mgs |
Val |
Choi |
Agma |
D-Glc |
1 |
−0.6 |
0.1 |
|
|
−1.6 |
0.1 |
−4.1 |
0.1 |
−0.6 |
0.1 |
10 |
898B |
1.74 |
819.4135 |
921.3522 |
Mgs |
Val |
Choi |
Aaep |
D-Glc |
1 |
−4.1 |
0.1 |
−1.3 |
0.1 |
−0.5 |
2.9 |
−0.6 |
1.1 |
0.4 |
0.5 |
11 |
888B
|
1.75 |
809.4291
|
911.3679 |
Mgs |
Ile |
Choi |
Agma |
D-Glc |
1 |
−2.5 |
1.0 |
−3.1 |
0.6 |
−0.5 |
0.6 |
−0.8
|
1.5
|
0.1 |
2.3 |
12 |
912B |
1.80 |
833.4291 |
935.3679 |
Mgs |
Ile |
Choi |
Aaep |
D-Glc |
1 |
−1.3 |
2.2 |
−1.9 |
3.1 |
0.4 |
27.8 |
1.5 |
32 |
1.3 |
11 |
13 |
1008A |
2.02 |
929.4502 |
1031.3890 |
Mgs |
Val |
Choi |
Agma |
D-Glc |
2 |
−1.3 |
0.7 |
0.3 |
0.2 |
0.7 |
0.1 |
|
|
0.0 |
0.1 |
14 |
1032A |
2.06 |
953.4502 |
1055.3890 |
Mgs |
Val |
Choi |
Aaep |
D-Glc |
2 |
−1.9 |
2.6 |
−3.7 |
1.1 |
0.7 |
2.9 |
−1.4 |
0.3 |
0.5 |
0.4 |
15 |
1022A
|
2.07 |
943.4659
|
1045.4047 |
Mgs |
Ile |
Choi |
Agma |
D-Glc |
2 |
−2.6 |
25 |
0.1
|
12
|
0.4 |
0.5 |
−0.8 |
0.2 |
1.7 |
7.1 |
16 |
1046A
|
2.10 |
967.4659
|
1069.4047 |
Mgs |
Ile |
Choi |
Aaep |
D-Glc |
2 |
−0.3
|
55
|
−0.8 |
76 |
0.7 |
24.3 |
0.4 |
6.4 |
1.8 |
35 |
17 |
1008B |
2.13 |
929.4502 |
1031.3890 |
Mgs |
Val |
Choi |
Agma |
D-Glc |
2 |
−2.0 |
0.2 |
−2.3 |
0.3 |
|
|
|
|
−0.5 |
0.3 |
18 |
1032B |
2.14 |
953.4502 |
1055.3890 |
Mgs |
Val |
Choi |
Aaep |
D-Glc |
2 |
−3.7 |
0.5 |
|
|
0.9 |
1.6 |
|
|
1.3 |
1.7 |
19 |
1022B |
2.20 |
943.4659 |
1045.4047 |
Mgs |
Ile |
Choi |
Agma |
D-Glc |
2 |
−3.0 |
2.8 |
−0.4 |
4.2 |
−0.4 |
0.3 |
0.4 |
0.1 |
0.6 |
4.1 |
20 |
1046B |
2.22 |
967.4659 |
1069.4047 |
Mgs |
Ile |
Choi |
Aaep |
D-Glc |
2 |
−1.8 |
8.2 |
|
|
0.8 |
12.5 |
0.7 |
2.4 |
1.3 |
20 |
21 |
1142 |
— |
1063.4870 |
1165.4258 |
Mgs |
Val |
Choi |
Agma |
D-Glc |
3 |
|
|
|
|
|
|
|
|
|
|
22 |
1166 |
— |
1087.4870 |
1189.4258 |
Mgs |
Val |
Choi |
Aaep |
D-Glc |
3 |
|
|
|
|
|
|
|
|
|
|
23 |
1156 |
2.46 |
1077.5027 |
1179.4414 |
Mgs |
Ile |
Choi |
Agma |
D-Glc |
3 |
−3.2 |
0.1 |
−5.1 |
0.1 |
|
|
|
|
|
|
24 |
1180 |
2.45 |
1101.5027 |
1203.4414 |
Mgs |
Ile |
Choi |
Aaep |
D-Glc |
3 |
−4.1 |
0.2 |
−3.6 |
0.4 |
|
|
|
|
|
|
Varlaxin purification
Nostoc sp. UHCC 0870 was cultivated in a 100 L flat-panel bioreactor in Z8 medium bubbled with air enriched with 1.5% CO2 at a constant temperature (28 °C) and illumination of 50 μmol photon per m2 per s to obtain 14 g of lyophilized biomass. Biomass was extracted three times with 50% MeOH (in H2O). The extract was then concentrated under vacuum using a rotary evaporator. The resulting extract (2.6 g) was chromatographed over normal-phase silica gel (100–200 mesh) flash column yielding 13 fractions eluted with step gradient hexane
:
diethyl ether (100
:
0, 25
:
75, 50
:
50 and 0
:
100) followed by chloroform
:
MeOH (100
:
0, 99
:
1, 98
:
2, 96
:
4, 92
:
8, 84
:
16, 68
:
32, 50
:
50 and 0
:
100). Fraction 10 (188 mg in total) eluted with 84
:
16 (chloroform
:
MeOH) was fractionated on preparative HPLC (Agilent 1260 Preparative Chromatograph equipped with quaternary pumps and MWD detector) on a Phenomenex Kinetex C18 column (250 × 21.2 mm, 5 μm) using H2O (A)/acetonitrile (B) both containing 0.1% formic acid as a mobile phase at a flow rate of 10 mL min−1. The linear gradient was as follows: A/B 85/15 (0 min), 85/15 (over 2 min), 0/100 (over 40 min), and 0/100 (over 45 min). Fraction 6 (eluted at 32–35 min, 25 mg) containing the desired compounds was further purified by semipreparative HPLC on a Phenomenex Kinetex EVO C18 column Phenomenex Kinetex EVO C18 column (250 × 10 mm, 5 μm, 100 Å) eluted with H2O (A)/acetonitrile (B) both containing 0.1% formic acid at a flow rate of 3 mL min−1 using following gradient: A/B 85/15 (0 min), 85/15 (in 1 min), 72/28 (in 8 min), and 72/28 (in 30 min). The compound elution was monitored on the MWD detector set to 220 nm, yielding 0.7 mg of purified varlaxin 1046A and 0.6 mg of purified varlaxin 1022A, eluting at 20 min and 19 min, respectively. The purified varlaxins 1046A and 1022A contained approximately 3–4% of other minor varlaxin variants.
Amino acid analysis
Solid purified varlaxins 1046A and 1022A and reference amino acids were transferred to 200 μL glass tubes inside a closed 4 mL glass vial containing 500 μL of 6 M HCl and hydrolysed at 110 °C for 21 h. Inner-tube HCl residues were vacuum evaporated and 100 μL of 1% Marfey's reagent (1-fluoro-2,4-dinitrophenyl-5-L-alanine or -L-leucine amide in acetone), 50 μL of H2O and 20 μL of 1 M NaHCO3 were added. The solution was incubated at 37 °C for 1 h and the reaction was stopped by adding 20 μL of 1 M HCl. Commercial reference compounds L-Ile, D-Ile, L-allo-Ile, and D-allo-Ile were obtained from Sigma-Aldrich (St. Louis, MO). The L-Choi reference amino acid was obtained from Microcystis aeruginosa NIES-298.19 Cells were grown, freeze dried, and extracted as described above. Solvent was evaporated from the crude extract and dry material was used as L-Choi reference material.
Monosaccharide analysis
D- and L-Glucose were purchased from Sigma-Aldrich. Hydrolysis of varlaxin 1022A and sample derivatizations were performed as previously described37 with the following exceptions: approximately 0.4 mg of varlaxin 1022A was hydrolysed, freeze dried instead of drying under N2 gas stream, and the reagents L-cysteine methyl ester (Sigma-Aldrich) and o-tolyl isothiocyanate (Thermo Fisher GmbH, Germany) instead of phenyl isothiocyanate were added simultaneously, and the reaction mixture was incubated at 60 °C for 1 h and diluted with MeOH before UPLC-QTOF analysis. Samples of 1 μL were analysed with two LC methods. The sample was injected into a Kinetex C8 column (50 or 100 × 2 mm, 1.7 μm, Phenomenex Inc.), which was eluted at 0.3 mL min−1 at 40 °C with 95% H2O (+0.1% formic acid, eluent A) and 5% ACN
:
isopropanol (1
:
1, +0.1% formic acid, eluent B). Eluent B was increased linearly to 100% in 5 minutes, kept at 2 minutes, then back to the initial condition in 0.5 minutes with 2.5 min post run before next injection. Additionally, the sample was eluted with Acquity UPLC® BEH C18 column (100 × 2.1 mm, 1.7 μm Waters Corp., MA) as previously described, but eluent B was increased linearly to 70% in 10 minutes, then to 100% in 0.10 minutes, kept at 3.99 minutes, and changed back to initial condition in 0.5 minutes with 5.5 min post run. QTOF was used in resolution mode with positive electrospray ionization. Leucine enkephalin was used as a lock mass, and sodium formate and Ultramark® 1621 for mass calibration. The retention time of monosaccharide thiocarbamoyl-thiazolidine derivative of D-Glc of varlaxin 1022A was 1.97 min (references D-Glc 1.97 min, L-Glc 1.88 min).
NMR and UV spectroscopy
Spectra were collected on a Bruker Avance III HD 800 MHz NMR spectrometer equipped with a cryogenically cooled TCI 1H, 13C, 15N triple-resonance probe head. Data were collected at 30 °C in DMSO-d6 (Table S3†). In addition to a 1H experiment with the solvent pre-saturation and broadband decoupled 13C experiment, homonuclear, two-dimensional (2D) TOCSY (TOtal Correlation SpectroscopY), NOESY (Nuclear Overhauser Enhancement SpectroscopY) and DQF-COSY (Double Quantum Filtered COrrelation SpectroscopY) experiments were performed. Heteronuclear 2D 1H–13C HSQC and 1H–15N HSQC (Heteronuclear Single Quantum Coherence) and 2D 1H–13C HMBC (Heteronuclear Multiple Bond Correlation) experiments (for the assignment of 1H, 13C and 15N chemical shifts) were also performed. The 2D TOCSY experiment was acquired with an isotropic mixing time of 60 ms. The mixing time for 2D NOESY spectrum was 300 ms. The 13C HMBC experiment (for observing long-range 1H–13C connectivity) was measured using nJCH transfer time optimized for 8 Hz couplings. For monosaccharide analysis, varlaxin 1046A was hydrolysed for 1 h at 100 °C in 0.5 mL of 2 M D2SO4 in D2O. The proton spectrum was collected, and anomeric signals were compared with data from a previous publication.38 UV spectra of varlaxins 1022A and 1046A, and a reference compound 4-hydroxyphenylacetic acid were measured in MeOH with a UV-1800 UV-Vis spectrophotometer (Shimadzu, Kyoto, Japan).
Trypsin inhibition
The inhibition of human trypsins was determined as described previously.23 The recombinant trypsinogens-1, -2 and -3 were produced in Escherichia coli and activated to trypsin-1, -2, and -3 as described previously.39 Enzyme inhibitory activity of varlaxins was determined in 96-well plates by adding 10 μL of varlaxins diluted in ultra-purified H2O (containing up to 0.027% DMSO), 15 μL of 0.1% bovine serum albumin in 50 mM Tris-buffered saline (BSA/TBS) and 25 μL of each trypsin (1.8 nM). Ultra-purified H2O (10 μL) was used as a control. DMSO at the concentrations used did not have any effect on trypsin activity (data not shown). The varlaxins were pre-incubated with trypsins at room temperature for 15 min before addition of 50 μL of 0.2 mM chromogenic substrate S-2222 (ChromogeniX) in ultra-purified H2O. The tested varlaxin 1046A and 1022A concentrations, after the addition of substrate, ranged from 0.01 nM, 1076 nM to and from 0.73 nM to 7339 nM to 0.73 nM, respectively. The change of absorbance at 405 nm was followed for 15 min (VICTOR X4 Multiple plate reader, PerkinElmer). The experiment was performed three times, each with 2 replicates (n = 3). The absorbance change was determined at the phase of the substrate reaction during which the absorbance of the control reaction increased linearly over time. The absolute IC50 values were estimated using Quest Graph™ (https://www.aatbio.com/tools/ic50-calculator). The porcine trypsin assay was performed as previously described.22
Genome sequencing and gene cluster analysis
The cyanobacterial strain Nostoc sp. UHCC 0870 was grown for 8 days at 20–21 °C under photon irradiance of 15 μmol m−2 s−1 in 150 mL of Z8 culture medium in a 500 mL Erlenmeyer flask. Genomic DNA was isolated using phenol–chloroform extraction as previously described.40 The quality and concentration of isolated DNA samples were assessed using an Agilent TapeStation (Agilent Technologies) and a NanoDrop 1000 spectrophotometer (Thermo Scientific), respectively. Whole-genome sequencing was performed using a combination of PacBio RSII and Illumina MiSeq. Nextera adapters were removed from Illumina data by cutadapt v1.14. PacBio data were assembled with HGAP3. Illumina data were assembled with SPAdes v3.11.1 with the “careful” option. Gap4 was used to combine assemblies and check sequence circularity. Bwa 0.7.12-r1039 was used to map Illumina reads against the combined assembly. Homopolymer errors were corrected using Pilon v1.16. The complete sequence was annotated using Prokka v1.13 and PANNZER2. All sequencing, assembly, and annotation were performed at the DNA Sequencing and Genomics laboratory, Institute of Biotechnology, University of Helsinki, Finland. The complete genome sequence of Nostoc sp. UHCC 0870 was deposited in the GenBank database (CP091913–CP091924). We analysed the substrate specificity of adenylation domains using NRPSpredictor2 analysis website (http://nrps.informatik.uni-tuebingen.de/). The condensation domain of VarB was analyzed with Natural Product Domain Seeker (NaPDoS) and compared with other known aeruginosin AerB.
Results
Analysis of methanol extracts of Nostoc sp. UHCC 0870 using UPLC-QTOF mass spectrometry led to the serendipitous discovery of two novel aeruginosins, which we named varlaxins 1046A and 1022A, after Varlaxudden on the Gulf of Finland from where the strain was isolated. Total ion current chromatogram from the methanol extract showed prominent peaks (retention time 2.07 and 2.10 min) at the peptide region (Fig. S4A†). The upper-range mass spectrum from these peaks contained two pairs of mass peaks, representing sodiated ions. The same compounds in desulfated protonated form showed the presence of a single sulfate group (Fig. S4B†). A broader-range mass spectrum showed neutral loss of methylglyceric acid (MgA)-Ile and ions matching to one of the common aeruginosin structures (Fig. S4C†). Low-mass nonspecific product ion spectrum (MSE) from these peaks showed diagnostic ions for aeruginosin substructures as Choi, Aaep, and MgA-Ile (Fig. S4D†). Analysis of product ion spectra (MS2) from the desulfated protonated ions m/z 967.46 and 943.46 gave preliminary structures (Fig. 1 and 2) without the exact locations of 4-hydroxyphenylacetic acid (Hpaa) residues or clarifying the nature of the hexose sugar (Fig. S5 and Table S4†).
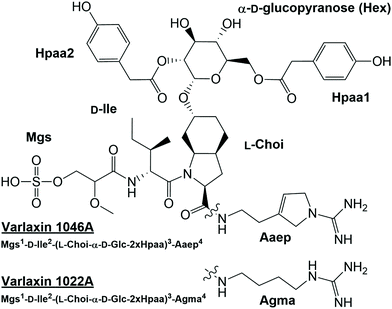 |
| Fig. 1 Structures of varlaxins 1046A and 1022A from Nostoc sp. UHCC 0870. Mgs=2-O-methylglyceric acid 3-O-sulfate, Choi = 2-carboxy-6-octahydroindole, Agma = 4-amidinobutylamide, Aaep = 1-amidino-3-(2-aminoethyl)-3-pyrroline, Hpaa = 4-hydroxyphenylacetic acid. | |
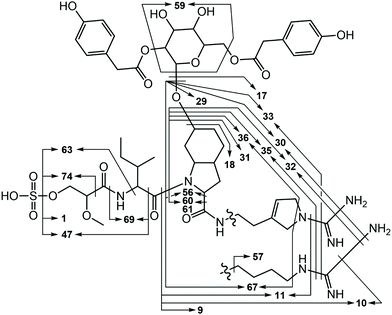 |
| Fig. 2 Major varlaxin MS2 fragments from desulfated variants 1046A, 1022A, 912A, 888B, 778, 764, and 754. Fragment numbers are given in Table S3.† | |
Further analysis of strains from the UHCC culture collection led to identification of 22 varlaxin chemical variants from five strains (Table 1). Mass spectrometric analyses (Fig. S5 and Table S4†) and retention time data showed that the chemical variation originates from the following three positions: Ile or Val in position 2, Aaep or Agma in position 4, and the number and location of Hpaa residues in the structure. Two variants with identical masses, but with different retention times, were detected from varlaxin chemical variants with one and two Hpaa residues (Table 1). Two variants with three Hpaa residues were detected, but low abundance prevented further characterization of these variants. This variation was not observed when Hpaa was absent from the structure. These observations suggest that variants with identical masses differ with respect to the positions of the Hpaa residues in the hexose unit. Therefore, the subunit sequence for the 22 varlaxins can be summarized as Mgs1-Ile/Val2-(Choi-Glc-(0–3 Hpaa))3-Agma/Aaep4 (Table 1). In all strains, Ile and Aaep dominated in positions 2 and 4, respectively, and in three strains variants with two Hpaa residues dominated. The Choi moiety was decorated with a hexose esterified with 0–3 Hpaa residues. UV spectra of varlaxin 1046A and 1022A near their maximum of 278 nm matched closely with spectrum of reference Hpaa, which is consistent with the existence of Hpaa substructure in varlaxins (Fig. S6†).
The structures of purified varlaxins 1046A and 1022A were completed and verified with 1D 1H and 13C spectra and 2D DQF-COSY, TOCSY, NOESY, 1H–1C HSQC, 1H–15N HSQC, and HMBC NMR spectra (Fig. S7, S8 and Tables S5, S6†). Two sets of δC/H values were recognized from both 1046A and 1022A data originating from cis/trans Ile-Choi rotamers in a 4
:
6 ratio (Table S5†). DQF-COSY correlations and HMBC/NOESY correlations were used for subunit connections (Fig. 3). HMBC correlations connected the two Hpaa residues to hexose positions 2 and 6 (Fig. 3). The hexose connected to Choi-OH was analysed from the hydrolysis mixture with NMR and LC-MS to be D-glucose (Fig. S8 and S9†). The configuration of the two amino acids in the varlaxin substructure were analysed with LC-MS of the Marfey's derivatives of purified varlaxin 1046A and 1022A acid hydrolysates. Comparison to commercial amino acid reference Ile isomers and Choi from Microcystis aeruginosa NIES-298 raw extract showed that Ile was in D-configuration and Choi was in the L-configuration in both varlaxins, which is the same configuration as Choi from Microcystis aeruginosa NIES 298 (Fig. S10†).
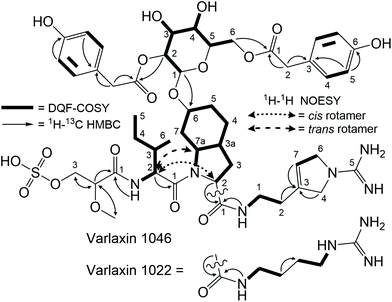 |
| Fig. 3 DQF-COSY, HMBC, and NOESY correlations of varlaxins 1022A and 1046A. | |
We sequenced the complete 7.17 Mb genome of Nostoc sp. UHCC 0870, which was organized in a 6.42 Mb chromosome, 10 plasmids, and a plasmid prophage. Bioinformatic analysis identified the putative 36 kb varlaxin (var) biosynthetic gene cluster, which contained 22 ORFs of which 10 shared similarity to gene products residing in other aeruginosin biosynthetic gene clusters (Table 2 and Fig. 4). VarB and VarG encode two bimodular NRPS enzymes, which form the varlaxin peptide backbone (Fig. 4). The first module of VarB consists of an O-methylglyceric acid transferase (OMT), a FkbH domain, peptidal carrier protein (PCP) and sulfotransferase (ST) (Fig. 4). The second module consists of condensation (C), adenylation (A), PCP and epimerization (E) domains (Fig. 4). The first module of this NRPS likely incorporate glycerate, which is further methylated and sulfated (Fig. 4), the second module loads allo-isoleucine. A loading module with an identical set of catalytic domains from the suomilide and dysinosin biosynthetic pathways is also proposed to synthesize Mgs.23,30 The VarB second adenylation domain binding pocket Stachelhaus sequence is DALWMGGVFK, which predicts, with a moderate 80% match, Val in the second position (Table S7†). However, according to chemical analysis by Marfey's method, this amino acid in varlaxins 1022A and 1046A was D-Ile. Biosynthetic gene clusters for ulleungmycin and desotamide cyclic peptides contain identical adenylation domain binding pocket sequence DALWMGGVFK in genes UlmA4 and DsaH4 without following epimerase.41,42 This resulted in incorporation and existence of L-allo-Ile in these peptides. Notably, the second domain of VarB contains an epimerase domain. When the substrate of this domain is L-allo-Ile, the C2 epimerization results in D-Ile, which is found in varlaxins 1022A and 1046A. Based on these three cases, the Stachelhaus sequence DALWMGGVFK is more specific for L-allo-Ile than for Val. The binding pocket of second adenylation domain of AerB from aeruginosins 98-A, -B, and -C is DAFFLGVTFK, of which amino acid prediction is Ile with strong 100% match (Tables S7 and S28†). Thus, the action of these two VarB second domains resulted in the incorporporation of D-Ile or D-allo-Ile in varlaxins or aeruginosins 98-A, -B, and -C, as adenylation domains recognize different amino acids (L-allo-Ile or L-Ile, respectively), and C2 epimerization in both cases generate the final Ile configurations (Fig. 4 and Table S7†). VarG, consists of C, A, PCP, C, and PCP domains, which load the Choi and arginine to the preceding moiety (Fig. 4). VarI glycosylates the Choi hydroxyl group. VarO is an adenylation (A) domain that could recognize the Hpaa substrate, which would be bound to acyl carrier protein (ACP) VarP. VarQ belongs to the MBOAT enzyme family, which could catalyse the addition of Hpaa to the glucose (Fig. 4).
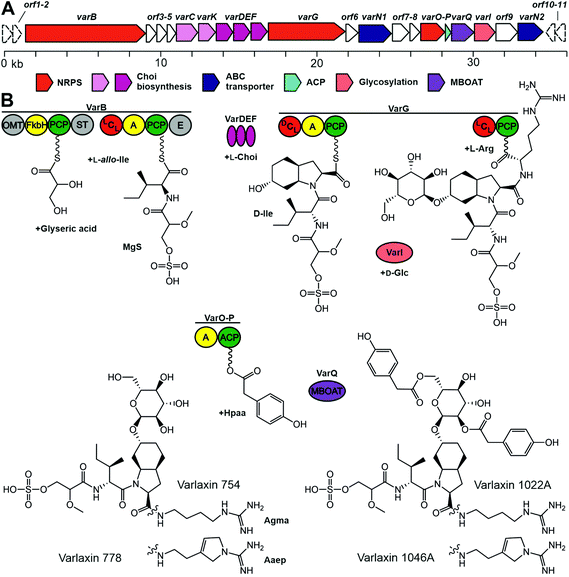 |
| Fig. 4 The varlaxin (var) biosynthetic gene cluster and putative biosynthetic scheme in Nostoc sp. UHCC 0870. A – Organization of predicted biosynthetic genes. B – Proposed biosynthetic pathway of varlaxin 1046A. FkbH (domain of unknown function), OMT (O-methylglyceric acid transferase); PCP (peptidyl carrier protein); ST (sulfotransferase); C (condensation domain); A (adenylation domain); E (epimerization domain); and MBOAT (membrane bound O-acyl transferase). | |
Table 2 Predicted varlaxin (var) biosynthetic gene cluster gene products
Protein |
Accession number |
Predicted function |
Length (aa) |
BLASTp top hit |
Description |
Scientific name |
Identity % |
Accession |
ORF1 |
UKO95746 |
|
156 |
Tetratricopeptide repeat protein |
Anabaena minutissima
|
96 |
WP_190695341 |
ORF2 |
UKO95747 |
|
449 |
FAD-binding oxidoreductase |
Anabaena minutissima
|
94 |
WP_190696554 |
VarB |
UKO95748 |
NRPS |
2853 |
Amino acid adenylation domain-containing protein |
Nostoc sp. FACHB-145 |
85 |
WP_190754768 |
ORF3 |
UKO95749 |
|
274 |
Hypothetical protein |
Nostoc sp. KVJ20 |
86 |
WP_069074352 |
ORF4 |
UKO95750 |
|
126 |
Nuclear transport factor 2 family protein |
Nostoc sp. |
84 |
WP_199337282 |
ORF5 |
UKO95751 |
Sulfo-transferase |
192 |
Adenylyl-sulfate kinase |
Scytonema sp. NIES-4073 |
90 |
WP_096564148 |
VarC |
NA |
|
720 |
Rieske 2Fe-2S domain-containing protein |
Nostoc sp. B(2019) |
86 |
WP_162397143 |
VarK |
UKO95752 |
|
351 |
Type 2 isopentenyl-diphosphate Delta-isomerase |
Scytonema sp. NIES-4073 |
92 |
WP_096564146 |
VarD |
UKO95753 |
Choi formation |
198 |
Bacilysin biosynthesis protein BacABacA |
Nostoc sp. LEGE 12450 |
90 |
WP_193945672 |
VarE |
UKO95754 |
Choi formation |
235 |
Cupin domain-containing protein |
Scytonema sp. NIES-4073 |
84 |
WP_096564145 |
VarF |
UKO95755 |
Choi formation |
264 |
SDR family oxidoreductase |
Nostoc sp. B(2019) |
90 |
WP_162397147 |
VarG |
UKO95756 |
NRPS |
1615 |
Non-ribosomal peptide synthetase |
Scytonema sp. NIES-4073 |
88 |
WP_096564143 |
ORF6 |
UKO95757 |
|
267 |
SDR family oxidoreductase |
Nostoc sp. ‘Peltigera membranacea cyanobiont’ 213 |
93 |
WP_094328879 |
VarN1 |
UKO95758 |
ABC transporter |
596 |
ABC transporter substrate-binding protein |
Anabaena sp. 4-3 |
83 |
WP_066426904 |
ORF7 |
UKO95759 |
|
385 |
Hypothetical protein |
Anabaena sp. CA = ATCC 33047 |
78 |
WP_066382146 |
ORF8 |
UKO95760 |
|
214 |
Hypothetical protein |
Anabaena
|
84 |
WP_066382147 |
VarO |
UKO95761 |
NRPS |
541 |
Amino acid adenylation domain-containing protein |
Nostoc linckia
|
82 |
WP_096542302 |
VarP |
UKO95762 |
ACP |
88 |
Acyl carrier protein |
Anabaena
|
80 |
WP_066382151 |
VarQ |
UKO95763 |
Hpaa transfer |
504 |
MBOAT family protein |
Anabaena sp. 4-3 |
89 |
WP_066426900 |
VarI |
UKO95764 |
Glycosylation |
423 |
Glycosyltransferase family 4 protein |
Anabaena sp. 4-3 |
85 |
WP_066426897 |
ORF9 |
UKO95765 |
|
457 |
Major facilitator superfamily transporter |
Nostoc sp. ‘Peltigera membranacea cyanobiont’ N6 |
87 |
AVH65385 |
VarN2 |
UKO95766 |
ABC transporter |
600 |
ATP-binding cassette domain-containing protein |
Anabaena sp. CA = ATCC 33047 |
83 |
WP_066382156 |
ORF10 |
UKO95767 |
|
294 |
Hypothetical protein |
Anabaena minutissima
|
89 |
WP_190700187 |
ORF11 |
UKO95768 |
|
273 |
3-Deoxy-7-phosphoheptulonate synthase |
Anabaena minutissima
|
97 |
WP_190700184 |
The trypsin inhibitory activity of varlaxins 1046A and 1022A was tested using both porcine and human trypsins. Inhibition of porcine trypsin was very effective with both varlaxin variants, with IC50 values of 14 nM and 740 nM, respectively (Fig. 5). This makes the varlaxin 1046A the most potent porcine trypsin inhibitor among the reported aeruginosins (Table S2†). As varlaxins are strong porcine trypsin inhibitors, the three human trypsin isoenzymes were also tested for inhibition. Both varlaxin variants inhibited all human trypsin isoenzymes (Fig. 5). Varlaxin 1046A inhibited human trypsin-2 and -3 with an IC50 values of 0.62 nM and 0.96 nM, respectively (Fig. 5), while trypsin-1 was inhibited at higher concentrations (IC50 3.6 nM).
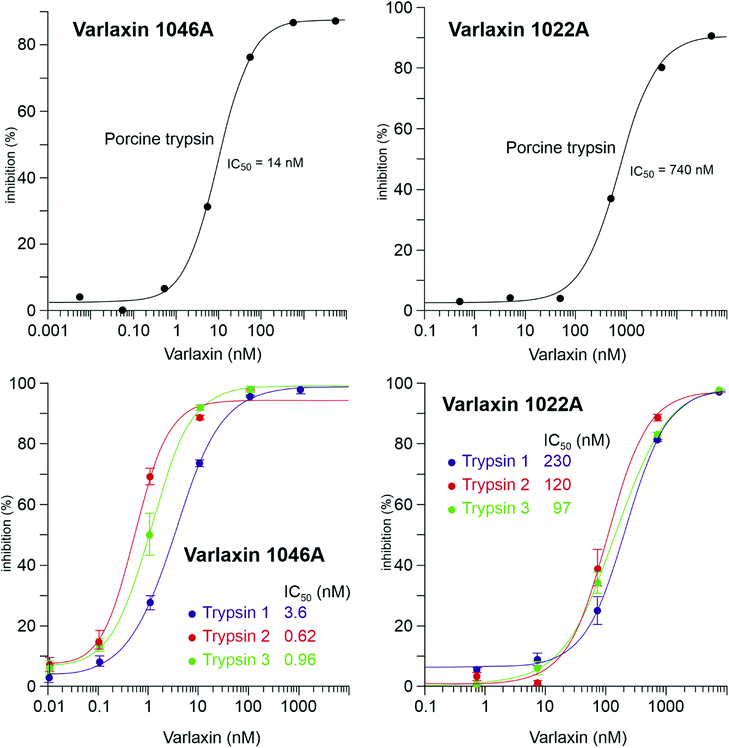 |
| Fig. 5 Dose response curves and the IC50 values of varlaxins 1046A and 1022A on porcine trypsin and three human trypsin-isoenzymes. | |
Discussion
We report here the identification and structural characterization of novel members of the aeruginosin family of natural products, varlaxin 1046A and 1022A. All identified varlaxin variants contain Choi at the third amino acid, a moiety which is characteristic for most of the aeruginosins, except for subclasses such as pseudoaeruginosins, suomilide, banyasides and nostosins (Table 1 and Table S1†). Thus, we propose that varlaxins are members of the aeruginosin family of natural compounds. The Choi moiety of the varlaxins reported here is decorated with a sugar, a decoration previously identified in about one quarter of the aeruginosins that contain a Choi moiety (Table S1†). The varlaxin α-D-glucopyranose moiety is esterified by 0–3 carboxylic acids (Table 1 and Fig. 1, 4). Approximately half of the other aeruginosin sugar units are decorated with carboxylic or sulfate groups.32 However, the Hpaa residue of varlaxins is part of the Choi-glucose subunit, making this substructure markedly different and unique among aeruginosins. This unique sugar decoration has been reported in plant sesquiterpene lactones, where glucose is decorated with three Hpaa residues.43 The N-terminus moiety of varlaxin is Mgs, which is rare among aeruginosins (Table 1 and Table S1†); only suomilide, banyasides, and four dysinosins contain this moiety.22,24,32 While the second moiety of varlaxins is primarily allo-Ile (identified from variants 1046A and 1022A, but could be isobaric Leu in other variants), Val was also found (Table 1). Both are nonpolar amino acids, which are the typical second amino acids in aeruginosins (Table S1†). The fourth substructure unit of varlaxins is either Aaep or Agma, of which Aaep is rarer than Agma in aeruginosins (Table S1†). In summary, the Mgs and Aaep structure and the Choi-Glu-Hpaa moiety make varlaxins unique amongst the aeruginosin family of natural products and justifies naming these compounds as a separate aeruginosin subclass.
The 36 kb varlaxin biosynthetic gene cluster is similar to previously reported aeruginosin biosynthetic gene clusters.23,26–31 Varlaxin biosynthesis begins with the incorporation of Mgs, catalyzed by a FkbH domain, which tailors O-methylglyceric acid (OMT) and sulfotransferase (ST) domains (Fig. 5). A similar organization of Mgs-incorporating domains is seen in the biosynthesis of cyanopeptolin 1138,48 dysinosin30 and suomilide.23 Phormidolide and oscillapeptin G both contain similar glyceric acid units that are incorporated by FkbH domains.49,50 FkbH domains use phosphoglycerate as their substrate.51 The predicted substrate for sulfotransferase is 3′-phosphoadenylyl sulfate, produced by ORF5 (adenylyl-sulfate kinase). The varlaxin biosynthetic gene cluster encodes the three Choi-forming enzymes, AerDEF (VarDEF in varlaxin BGC).27 The varlaxin biosynthetic gene cluster also includes both AerC (VarC) and AerK (VarK), which are predicted to participate in Choi formation. However, Choi-containing aeruginosin NAL2 is produced without either of these enzymes.29 No study to date has provided a reasonable model for formation of Aaep and this is likely to involve novel biochemistry. However, when comparing aeruginosin biosynthetic gene clusters and their products, AerC (VarC) can be found exclusively in biosynthetic gene clusters that produce aeruginosins with Aaep.25 AerH has been predicted to act in Aaep formation.27 However, no AerH homolog was found in the varlaxin biosynthetic gene cluster (Fig. 5). The varlaxin biosynthetic gene clusters encodes three unique proteins, VarO-Q, that are not found in other aeruginosin biosynthetic gene clusters and predicted here to attach Hpaa residues to the glucose (Fig. 5). MBOAT enzymes have also been shown to act on saccharides.52 The timing of individual biosynthetic steps is unclear but glycosylation of Choi may occur prior to release of the final product because it appears in all of the varlaxins detected. The fact that there are mono-, di- and tri-Hpaa-ester derivatives suggest that this step occurs subsequent to release from VarG. Varlaxin 1046A is produced in two-fold higher abundance than varlaxin 1022A, indicating that varlaxin 1046A is the main product of the varlaxin biosynthetic gene cluster (Table 1). The other varlaxin chemical variants are likely to be side products from unspecific substrate specificities of the VarB adenylation domains and incomplete action of the possible tailoring reactions.
The aeruginosins have frequently been observed to inhibit serine proteases.18 Varlaxin 1046A appears to be the most potent cyanobacterial trypsin inhibitor reported and is considerably more potent than commercial peptidic inhibitors leupeptin and antipain (Fig. 5 and Table S2†). The high potency of varlaxin 1046A in inhibiting trypsin extends to human trypsin isoenzymes. Furthermore, varlaxins exhibit similar inhibition profile as suomilide, inhibiting trypsin-2 and -3 more potently than trypsin-1 (23, Fig. 5). Significantly, varlaxin 1046A was approximately 50 to 200 times more potent than varlaxin 1022A in inhibiting the trypsin isoenzymes (Fig. 5). The only difference between these two varlaxins is the fourth moiety, which is Aaep in varlaxin 1046A and Agma in varlaxin 1022A. In previous studies, the presence of Aaep was shown to impact the trypsin-inhibiting ability of aeruginosins, as studied using natural and synthetic aeruginosin analogues.40,44 Furthermore, molecular-dynamics simulation has shown that the Aaep moiety of suomilide is important for interaction with trypsins.23 Some other potent trypsin inhibitors (i.e., aeruginosins 205A and 205B) have Agma in that position,44 which is consistent with our results that Agma-containing varlaxin 1022A is also a potent human trypsin inhibitor, although not as potent as varlaxin 1046A with Aaep. Crystal structures of trypsin-aeruginosin 98-B and thrombin-aeruginosin 298-A complexes have been reported.45,46 In these studies, the arginine derivative is recognized in an active site and a guanidinyl group derivative of Aaep appears to bind more strongly than other Arg derivatives, again suggesting the importance of Aaep for the inhibitory activity. However, the other three moieties (hydroxy acid, hydrophobic amino acid and Choi) in the aeruginosins may also interact with the catalytic site-surrounded surface (Table S2†). It should be noted that the aeruginosins in the studies above were studied with undefined trypsin,44–48 which is most likely of non-human origin as in most of such studies. Since our results indicate that there are significant differences in the potency of varlaxins inhibiting highly similar human trypsins, the results reported in the literature with different trypsins should be interpreted with caution. Furthermore, it would be important to describe the trypsin preparations used in more detail.
Conclusion
We report here the structural elucidation and activity of two novel linear peptides belonging to the aeruginosin family, varlaxins 1046A and 1022A. Their classification as members of the aeruginosin family is based on their linear nature and the presence of moieties such as Choi, Agma, Aaep, and Mgs, where Choi and Agma are shared by many aeruginosins. Bioinformatic analysis of the varlaxin biosynthetic gene cluster confirms that varlaxins are members of the aeruginosin family of natural products and share a common biosynthetic pathway. Varlaxin 1046A was found to be the most potent reported trypsin inhibitor among the aeruginosins. Importantly, varlaxins also inhibited human trypsins. Thus, to the best of our knowledge, varlaxin is the second cyanobacterial trypsin inhibitor tested with human trypsin isoenzymes after suomilide.23 Inhibition of human trypsin-3 may be particularly significant, as trypsin-3 is a potential drug target for metastatic cancers.8–10,53 As there are currently no specific small-molecule inhibitors for trypsin-3, varlaxin 1046A, as a sub-nanomolar trypsin inhibitor showing preference for trypsin-2 and -3 inhibition, is a potential lead molecule for drug development. While we call for testing of other cyanobacterial trypsin inhibitors against human trypsin isoenzymes, the varlaxin variants reported here may facilitate the development of more selective trypsin-3 inhibitors for clinical applications.
Author contributions
LH, KS, and DF designed the study. MW, MNA, PP, KS, PH, and LH performed the experiments. LH, HK, JJ, MNA, KS, PH, and DF analysed and interpreted the data. LH, MNA, JJ, HK, DF, and KS wrote the manuscript, which was corrected, revised, and approved by all authors.
Conflicts of interest
There are no conflicts to declare.
Acknowledgements
We would like to thank Annikki Löfhjelm and Lyudmila Saari for their excellent technical assistance. We would also like to acknowledge support from the Sigrid Jusélius Foundation, the Magnus Ehrnrooth Foundation, the Jane and Aatos Erkko Foundation, the Nordforsk Nordic centre of Excellency NordAqua (project number #82845), and the University of Helsinki's Doctoral Programme in Microbiology and Biotechnology funding to L. P. H. and M. N. A. We thank the Language Centre of the University of Helsinki for their assistance in language revisions.
References
- A. W. Lambert, D. R. Pattabiraman and R. A. Weinberg, Emerging Biological Principles of Metastasis, Cell, 2017, 168(4), 670–691 CrossRef CAS PubMed.
- B. Turk, Targeting proteases: successes, failures and future prospects, Nat. Rev. Drug Discovery, 2006, 5(9), 785–799 CrossRef CAS PubMed.
- L. Sevenich and J. A. Joyce, Pericellular proteolysis in cancer, Genes Dev., 2014, 28(21), 2331–2347 CrossRef PubMed.
- P. Nyberg, M. Ylipalosaari, T. Sorsa and T. Salo, Trypsins and their role in carcinoma growth, Exp. Cell Res., 2006, 312(8), 1219–1228 CrossRef CAS PubMed.
- A. Paju and U. H. Stenman, Biochemistry and clinical role of trypsinogens and pancreatic secretory trypsin inhibitor, Crit. Rev. Clin. Lab. Sci., 2006, 43(2), 103–142 CrossRef CAS PubMed.
- C. N. M. Nyaruhucha, M. Kito and S. I. Fukuoka, Identification and expression of the cDNA-encoding human mesotrypsin(ogen), an isoform of trypsin with inhibitor resistance, J. Biol. Chem., 1997, 272(16), 10573–10578 CrossRef CAS PubMed.
- M. Sahin-Tóth, Human mesotrypsin defies natural trypsin inhibitors, From passive resistance to active destruction, Protein Pept. Lett., 2005, 12(5), 457 CrossRef PubMed.
- A. Hockla, D. C. Radisky and E. S. Radisky, Mesotrypsin promotes malignant growth of breast cancer cells through shedding of CD109, Breast Cancer Res. Treat., 2010, 124(1), 27 CrossRef CAS PubMed.
- G. Jiang, F. Cao, G. Ren, D. Gao, V. Bhakta and Y. Zhang,
et al., PRSS3 promotes tumour growth and metastasis of human pancreatic cancer, Gut, 2010, 59(11), 1535–1544 CrossRef CAS PubMed.
- A. Hockla, E. Miller, M. A. Salameh, J. A. Copland, D. C. Radisky and E. S. Radisky, PRSS3/mesotrypsin is a therapeutic target for metastatic prostate cancer, Mol. Cancer Res., 2012, 10(12), 1555–1566 CrossRef CAS PubMed.
- C. Rolland-Fourcade, A. Denadai-Souza, C. Cirillo, C. Lopez, J. O. Jaramillo and C. Desormeaux,
et al., Epithelial expression and function of trypsin-3 in irritable bowel syndrome, Gut, 2017, 66(10), 1767–1778 CrossRef CAS PubMed.
- J. Oiva, O. Itkonen, R. Koistinen, K. Hotakainen, W. M. Zhang and E. Kemppainen,
et al., Specific Immunoassay Reveals Increased Serum Trypsinogen 3 in Acute Pancreatitis, Clin. Chem., 2011, 57(11), 1506–1513 CrossRef CAS PubMed.
- G. Katona, G. I. Berglund, J. Hajdu, L. Gráf and L. Szilágyi, Crystal structure reveals basis for the inhibitor resistance of human brain trypsin, J. Mol. Biol., 2002, 315(5), 1209–1218 CrossRef CAS PubMed.
- P. Wu, J. Weisell, M. Pakkala, M. Peräkyla, L. Zhu and R. Koistinen,
et al., Identification of novel peptide inhibitors for human trypsins, Biol. Chem., 2010, 391(2–3), 283–293 CrossRef CAS PubMed.
- M. A. Salameh, A. S. Soares, A. Hockla, D. C. Radisky and E. S. Radisky, The P(2)’ residue is a key determinant of mesotrypsin specificity: engineering a high-affinity inhibitor with anticancer activity, Biochem. J., 2011, 440(1), 95–105 CrossRef CAS PubMed.
- O. Kayode, Z. Huang, A. S. Soares, T. R. Caulfield, Z. Dong and A. M. Bode,
et al., Small molecule inhibitors of mesotrypsin from a structure-based docking screen, PLoS One, 2017, 12(5), e0176694 CrossRef PubMed.
- O. Schilling, M. L. Biniossek, B. Mayer, B. Elsässer, H. Brandstetter and P. Goettig,
et al., Specificity profiling of human trypsin-isoenzymes, Biol. Chem., 2018, 399(9), 997–1007 CAS.
- K. Ersmark, J. R. del Valle and S. Hanessian, Chemistry and biology of the aeruginosin family of serine protease inhibitors, Angew. Chem., Int. Ed., 2008, 47(7), 1202–1223 CrossRef CAS PubMed.
- M. Murakami, Y. Okita, H. Matsuda, T. Okino and K. Yamaguchi, Aeruginosin 298-A, a thrombin and trypsin inhibitor from the blue-green alga Microcystis aeruginosa (NIES-298), Tetrahedron Lett., 1994, 35(19), 3129–3132 CrossRef CAS.
- L. Liu, A. Budnjo, J. Jokela, B. E. Haug, D. P. Fewer and M. Wahlsten,
et al., Pseudoaeruginosins, nonribosomal peptides in nodularia spumigena, ACS Chem. Biol., 2015, 10(3), 725–733 CrossRef CAS PubMed.
- L. Liu, J. Jokela, M. Wahlsten, B. Nowruzi, P. Permi and Y. Z. Zhang,
et al., Nostosins, trypsin inhibitors isolated from the terrestrial cyanobacterium Nostoc sp. strain FSN, J. Nat. Prod., 2014, 77(8), 1784–1790 CrossRef CAS PubMed.
- K. Fujii, K. Sivonen, K. Adachi, K. Noguchi, Y. Shimizu and H. Sano,
et al., Comparative study of toxic and non-toxic cyanobacterial products: A novel glycoside, suomilide, from non-toxic Nodularia spumigena HKVV, Tetrahedron Lett., 1997, 38(31), 5529–5532 CrossRef CAS.
- M. N. Ahmed, M. Wahlsten, J. Jokela, M. Nees, U. H. Stenman and D. O. Alvarenga,
et al., Potent Inhibitor of Human Trypsins from the Aeruginosin Family of Natural Products, ACS Chem. Biol., 2021, 16(11), 2537–2546 CrossRef CAS PubMed.
- A. Pluotno and S. Carmeli, Banyasin A and banyasides A and B, three novel modified peptides from a water bloom of the cyanobacterium Nostoc sp., Tetrahedron, 2005, 61(3), 575–583 CrossRef CAS.
- A. Kapuścik, P. Hrouzek, M. Kuzma, S. Bártová, P. Novák and J. Jokela,
et al., Novel Aeruginosin-865 from Nostoc sp. as a potent anti-inflammatory agent, ChemBioChem, 2013, 14(17), 2329–2337 CrossRef PubMed.
- L. Frangeul, P. Quillardet, A. M. Castets, J. F. Humbert, H. C. P. Matthijs and D. Cortez,
et al., Highly plastic genome of Microcystis aeruginosa PCC 7806, a ubiquitous toxic freshwater cyanobacterium, BMC Genomics, 2008, 9, 274 CrossRef PubMed.
- K. Ishida, G. Christiansen, W. Y. Yoshida, R. Kurmayer, M. Welker and N. Valls,
et al., Biosynthesis and structure of aeruginoside 126A and 126B, cyanobacterial peptide glycosides bearing a 2-carboxy-6-hydroxyoctahydroindole moiety, Chem. Biol., 2007, 14(5), 565–576 CrossRef CAS PubMed.
- K. Ishida, M. Welker, G. Christiansen, S. Cadel-Six, C. Bouchier and E. Dittmann,
et al., Plasticity and evolution of aeruginosin biosynthesis in cyanobacteria, Appl. Environ. Microbiol., 2009, 75(7), 2017–2026 CrossRef CAS PubMed.
- D. P. Fewer, J. Jokela, E. Paukku, J. Österholm, M. Wahlsten and P. Permi,
et al., New structural variants of aeruginosin produced by the toxic bloom forming cyanobacterium Nodularia spumigena, PLoS One, 2013, 8(9), e73618 CrossRef CAS PubMed.
- M. A. Schorn, P. A. Jordan, S. Podell, J. M. Blanton, V. Agarwal and J. S. Biggs,
et al., Comparative Genomics of Cyanobacterial Symbionts Reveals Distinct, Specialized Metabolism in Tropical Dysideidae Sponges, mBio, 2019, 10(3), e00821-19 CrossRef PubMed.
- D. S. May, C. M. Crnkovic, A. Krunic, T. A. Wilson, J. R. Fuchs and J. E. Orjala, 15 N Stable Isotope Labeling and Comparative Metabolomics Facilitates Genome Mining in Cultured Cyanobacteria, ACS Chem. Biol., 2020, 15(3), 758–765 CrossRef CAS PubMed.
- M. R. Jones, E. Pinto, M. A. Torres, F. Dörr, H. Mazur-Marzec and K. Szubert,
et al., CyanoMetDB, a comprehensive public database of secondary metabolites from cyanobacteria, Water Res., 2021, 196, 117017 CrossRef CAS PubMed.
- A. Calteau, D. P. Fewer, A. Latifi, T. Coursin, T. Laurent and J. Jokela,
et al., Phylum-wide comparative genomics unravel the diversity of secondary metabolism in Cyanobacteria, BMC Genomics, 2014, 15(1), 977 CrossRef PubMed.
- E. Dittmann, M. Gugger, K. Sivonen and D. P. Fewer, Natural Product Biosynthetic Diversity and Comparative Genomics of the Cyanobacteria, Trends Microbiol., 2015, 23(10), 642–652 CrossRef CAS PubMed.
- J. C. Roach, K. Wang, L. Gan and L. Hood, The molecular evolution of the vertebrate trypsinogens, J. Mol. Evol., 1997, 45(6), 640–652 CrossRef CAS PubMed.
-
J. Kotai, Instructions for preparation of modified nutrient solution Z8 for algae, Blindern B–11/69, Norwegian Institute for Water Research, Oslo, 1972 Search PubMed.
- Y. H. Wang, B. Avula, X. Fu, M. Wang and I. A. Khan, Simultaneous determination of the absolute configuration of twelve monosaccharide enantiomers from natural products in a single injection by a UPLC-UV/MS method, Planta Med., 2012, 78(8), 834–837 CrossRef CAS PubMed.
- J. L. Giner, J. Feng and D. J. Kiemle, NMR Tube Degradation Method for Sugar Analysis of Glycosides, J. Nat. Prod., 2016, 79(9), 2413–2417 CrossRef CAS PubMed.
- H. Koistinen, R. Koistinen, W. M. Zhang, L. Valmu and U. H. Stenman, Nexin-1 inhibits the activity of human brain trypsin, Neuroscience, 2009, 160(1), 97–102 CrossRef CAS PubMed.
- D. P. Fewer, J. Österholm, L. Rouhiainen, J. Jokela, M. Wahlsten and K. Sivonen, Nostophycin biosynthesis is directed by a hybrid polyketide synthase-nonribosomal peptide synthetase in the toxic cyanobacterium Nostoc sp. strain 152, Appl. Environ. Microbiol., 2011, 77(22), 8034–8040 CrossRef CAS PubMed.
- S. Son, Y. S. Hong, M. Jang, K. T. Heo, B. Lee and J. P. Jang,
et al., Genomics-Driven Discovery of Chlorinated Cyclic Hexapeptides Ulleungmycins A and B from a Streptomyces Species, J. Nat. Prod., 2017, 80(11), 3025–3031 CrossRef CAS PubMed.
- Q. Li, Y. Song, X. Qin, X. Zhang, A. Sun and J. Ju, Identification of the Biosynthetic Gene Cluster for the Anti-infective Desotamides and Production of a New Analogue in a Heterologous Host, J. Nat. Prod., 2015, 78(4), 944–948 CrossRef CAS PubMed.
- R. A. Sessa, M. H. Bennett, M. J. Lewis, J. W. Mansfield and M. H. Beale, Metabolite profiling of sesquiterpene lactones from Lactuca species, Major latex components are novel oxalate and sulfate conjugates of lactucin and its derivatives, J. Biol. Chem., 2000, 275(35), 26877–26884 CrossRef CAS.
- H. J. Shin, H. Matsuda, M. Murakami and K. Yamaguchi, Aeruginosins 205A and -B, serine protease inhibitory glycopeptides from the cyanobacterium Oscillatoria agardhii (NIES-205), J. Org. Chem., 1997, 62(6), 1810–1813 CrossRef CAS.
- B. Sandler, M. Murakami and J. Clardy, Atomic structure of the trypsin – Aeruginosin 98-B complex, J. Am. Chem. Soc., 1998, 120(3), 595–596 CrossRef CAS.
- J. L. R. Steiner, M. Murakami and A. Tulinsky, Structure of thrombin inhibited by aeruginosin 298-A from a blue-green alga, J. Am. Chem. Soc., 1998, 120(3), 597–598 CrossRef CAS.
- G. Wang, N. Goyal and B. Hopkinson, Preparation of l-proline based aeruginosin 298-A analogs: Optimization of the P1-moiety, Bioorg. Med. Chem. Lett., 2009, 19(14), 3798–3803 CrossRef CAS PubMed.
- T. B. Rounge, T. Rohrlack, A. Tooming-Klunderud, T. Kristensen and K. S. Jakobsen, Comparison of cyanopeptolin genes in Planktothrix, Microcystis, and Anabaena strains: evidence for independent evolution within each genus, Appl. Environ. Microbiol., 2007, 73(22), 7322–7330 CrossRef CAS PubMed.
- T. B. Rounge, T. Rohrlack, A. J. Nederbragt, T. Kristensen and K. S. Jakobsen, A genome-wide analysis of nonribosomal peptide synthetase gene clusters and their peptides in a Planktothrix rubescens strain, BMC Genomics, 2009, 10, 396 CrossRef PubMed.
- M. J. Bertin, A. Vulpanovici, E. A. Monroe, A. Korobeynikov, D. H. Sherman and L. Gerwick,
et al., The Phormidolide Biosynthetic Gene Cluster: A trans-AT PKS Pathway Encoding a Toxic Macrocyclic Polyketide, ChemBioChem, 2016, 17(2), 164–173 CrossRef CAS PubMed.
- Y. Sun, H. Hong, F. Gillies, J. B. Spencer and P. F. Leadlay, Glyceryl-S-acyl carrier protein as an intermediate in the biosynthesis of tetronate antibiotics, ChemBioChem, 2008, 9(1), 150–156 CrossRef CAS PubMed.
- D. Sychantha, D. J. Little, R. N. Chapman, G. J. Boons, H. Robinson and P. L. Howell,
et al., PatB1 is an O-acetyltransferase that decorates secondary cell wall polysaccharides, Nat. Chem. Biol., 2018, 14(1), 79–85 CrossRef CAS PubMed.
- H. Ma, A. Hockla, C. Mehner, M. Coban, N. Papo and D. C. Radisky,
et al., PRSS3/Mesotrypsin and kallikrein-related peptidase 5 are associated with poor prognosis and contribute to tumor cell invasion and growth in lung adenocarcinoma, Sci. Rep., 2019, 9(1), 1844 CrossRef PubMed.
Footnote |
† Electronic supplementary information (ESI) available. See DOI: 10.1039/d1ob02454j |
|
This journal is © The Royal Society of Chemistry 2022 |