DOI:
10.1039/D2NR01817A
(Paper)
Nanoscale, 2022,
14, 11152-11161
Effects of hydrophilic fullerene nanoarchitectured structures on the behaviour of neural stem cells†
Received
1st April 2022
, Accepted 2nd July 2022
First published on 25th July 2022
Abstract
The interaction between nanoarchitectonic fullerenes and cells is essential for their applications in the biological field. Herein we reported the preparation and investigation of the function of different types of water-dispersible self-assembled fullerenes. The hydrophobic self-assembled fullerenes were either surface-modified or chemically etched to become water dispersible. Different types of fullerenes were then examined for their effects on the behavior of neural stem cells (NSCs). Our results indicated that only the hydrophilic fullerene nanotubes (FNTs, diameter ∼480 nm) created by chemically etching were endocytosed by NSCs, which showed a spindle-like morphology after the uptake. Meanwhile, the FNTs did not increase the reactive oxygen species (ROS) production of the cells. The expression levels of neural-related genes (CNPase and β-tubulin) were upregulated 1.5-fold in the presence of FNTs. The differentiation of NSCs depended on the size, shape, and surface functional group of various fullerenes. Besides, the addition of FNTs in a chitosan self-healing hydrogel did not influence the integrity, injectability, and self-healing properties of the composite hydrogel. These results revealed that FNTs induced the neural differentiation of NSCs in the composite hydrogel. The addition of FNTs at a low concentration (50 μg mL−1) was enough to create such effects in the composite hydrogel. The expression levels of the oligodendrocytic marker gene CNPase and the neuronal marker gene β-tubulin were increased remarkably by ∼14.5- and ∼8.4-fold, respectively, by the composite self-healing hydrogel containing 50 μg mL−1 FNTs. The fullerene nanoarchitectured structures may have potential for use as nanovehicles and in neural tissue engineering in the future.
1. Introduction
Nanocarbons, including fullerenes, carbon nanotubes, and graphene, are attractive materials because of their unique photoelectric, thermal, chemical, and mechanical properties.1 Among them, fullerenes have a highly symmetric cage with abundant carbon–carbon double bonds and fewer unfilled molecular orbitals, which may scavenge free radicals.2,3 Fullerenes with unique morphologies and physical properties have been developed for biomedical applications, such as photodynamic therapy, antiviral/anti-bacterial agents, biosensors, antioxidants, imaging, and drug delivery.4–6 Moreover, fullerenes as zero-dimensional molecules can be prepared to build higher dimensional constructs.7 However, conventional fullerenes are often limited by hydrophobicity and compatibility.6 Hydrophilic fullerenes are preferred to hydrophobic fullerenes for biomedical applications.
Fullerenes and their derivatives demonstrate neuroprotective effects in the central nervous system.3,8 Hydrophobic fullerenes can self-assemble into various nanoarchitectured forms and structures including nanorods, nanosheets, cubes, and so on.9–11 Self-assembled fullerene nanosheets were found to improve the neural differentiation of human fibroblasts with the transfection of photosensitive protein plasmids.12 Aligned fullerene nanowhiskers promoted the orientation and neural differentiation of neural stem cells.13 There are few studies on utilizing nanocarbons of different shapes to control stem cell behavior. Meanwhile, these fullerene crystals have limited hydrophilicity and the surface of these compounds has to be modified further before culture with cells on a two-dimensional (2D) tissue culture plate. Besides, these fullerene crystals are hardly applicable to three-dimensional (3D) cell culture. Self-assembled fullerene nanoarchitectured structures with hydrophilic properties were further fabricated and characterized.14 The unique hydrophilic modification process provides an opportunity for investigating the direct effect of such fullerene nanoarchitectonics on 2D or 3D cultured cells.
Hydrogels are of great interest for clinical applications, particularly in 3D neural regeneration.15 Hydrogels may affect the fate of grafted cells in neural systems by supporting their attachment, neurite outgrowth, proliferation, migration, differentiation, and viability.16,17 Chitosan is a biocompatible material broadly used in the field of biomedicine, and in many occasions, it can promote the stemness of various types of stem cells.18 Chitosan-based self-healing hydrogels have tunable mechanical properties through network adjustment by dynamic covalent bonding within the hydrogels.19,20 In addition, chitosan self-healing hydrogels are injectable and have been used to enhance the survival rate of neural progenitors to repair the damaged central nervous system.21–23 Chitosan self-healing hydrogels, with a modulus similar to that of neural tissues, are thus suitable materials for the 3D culture of neural stem cells.
Neural differentiation is associated with low rigidity and geometric factor of the microenvironment.24 Different types of self-assembled fullerenes may have different effects on neural stem cell proliferation and differentiation. Besides, self-assembled fullerene nanostructures combined with low modulus chitosan self-healing hydrogels may have a potential benefit for 3D neural tissue engineering. Here, we prepared self-assembled fullerene nanotubes and fullerenes of other nanoarchitecture structures and compared their effects on neural differentiation. We also examined the cell differentiation effect from environmental cues provided by combining chitosan self-healing hydrogels and self-assembled fullerene nanotubes. We attempted to evaluate if the self-assembled form of fullerenes with hydrophilic properties and controllable nanoarchitectonics may facilitate the growth and neural differentiation of neural stem cells and if such an effect may be reserved in soft hydrogels.
2. Results and discussion
2.1. Preparation of hydrophobic or hydrophilic self-assembled fullerenes and their morphologies
Fullerene nanoarchitectured structures such as fullerene nanorods (FNRs) and fullerene nanosheets (FNSs) were produced by the self-assembly of fullerenes by the liquid–liquid interfacial precipitation (LLIP) method. Meanwhile, by selective chemical etching using ethylene diamine (EDA), hydrophilic nanotubes (FNTs) and nanosheets (eFNSs) were prepared, which were readily water dispersible. The hydrophobic FNRs were not water dispersible; but after surface modification with Pluronic P123, the modified FNRs (abbreviated as PFNRs) became water dispersible. Three different hydrophilic self-assembled fullerene structures (FNTs, eFNSs, and PFNRs) were tested.
The preparation of hydrophilic self-assembled fullerenes is illustrated in Fig. 1A. Different forms of hydrophobic and hydrophilic self-assembled fullerenes are shown in Fig. 1B. The hydrophobic self-assembled fullerenes were not well dispersed in aqueous solution. They agglomerated on the water surface or attached to the glass bottle, as shown in Fig. 1B. After EDA selective etching, FNTs and eFNSs became uniformly dispersed in the aqueous solution. The difference in the hydrophilic/hydrophobic properties was also defined quantitatively by coating an equal amount of materials on the slide and measuring the surface contact angle. The contact angle was reduced from 107.9 degrees to 35.1 degrees for FNRs and PFNRs on coated slides (Fig. S1†). Meanwhile, the contact angle was reduced from 107.9 degrees to 29.4 degrees from FNRs to FNTs on coated slides. EDA etching provided more hydrophilicity than Pluronic coating. Fig. 1C shows the scanning electron microscopy (SEM) images of the self-assembled fullerenes. The tubular structure of FNTs was acquired by selective chemical etching of FNRs using EDA. The porous surface of eFNSs was obtained after the selective chemical etching of FNSs. The size distribution of the self-assembled fullerenes obtained by LLIP, EDA chemical etching, and Pluronic coating is illustrated in Fig. S2.† The average length of FNRs was 2.1 ± 0.4 μm and the average diameter was 0.56 ± 0.11 μm. The average length of PFNRs was 2.0 ± 0.2 μm and the average diameter was 0.56 ± 0.09 μm. The average length of FNTs was 1.5 ± 0.4 μm and the average diameter was 0.48 ± 0.07 μm. The average diagonal size of FNSs was 4.9 ± 0.5 μm while that of eFNSs was about 3.8 ± 0.7 μm. Comparing the sizes of FNRs and FNTs, a decrease in the length and diameter of FNTs caused by chemical selective etching was observed. The length of FNTs was reduced by 0.6 μm, and the diameter was only reduced by 0.08 μm. Similarly, the size of eFNSs was 1.1 μm smaller than that of FNSs.
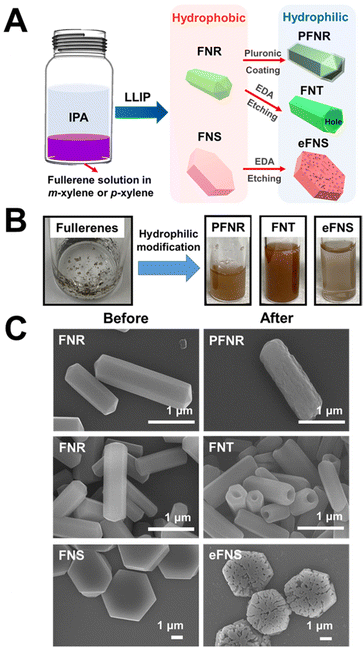 |
| Fig. 1 Synthesis of the self-assembled hydrophilic fullerenes. (A) The schematics showing the preparation of PFNTs, FNTs, and eFNSs. (B) Images of the unsolvable fullerene and the dispersion of PFNRs, FNTs, and eFNSs in water. (C) SEM images taken before and after hydrophilic modification. | |
2.2. Characterization of hydrophobic and hydrophilic self-assembled fullerenes
The Raman scattering spectra of FNRs, PFNRs, FNTs, FNSs, and eFNSs are very similar to that of pristine C60 (pC60), as displayed in Fig. 2A. The spectrum of pC60 showed two Ag bands (Ag (1) breathing mode of the peak at 495 cm−1 and the Ag (2) pentagonal pinch mode of the peak at 1464 cm−1) as well as the other six Hg bands (marked by arrows).25 Among all active peaks, the Ag (2) band is sensitive to the intermolecular binding of C60. Note that the Ag (2) peak position did not shift after self-assembly and surface modification (either by Pluronic coating or EDA etching), indicating that most of the C60 molecules still dominated in PFNR, FNT, and eFNS samples.
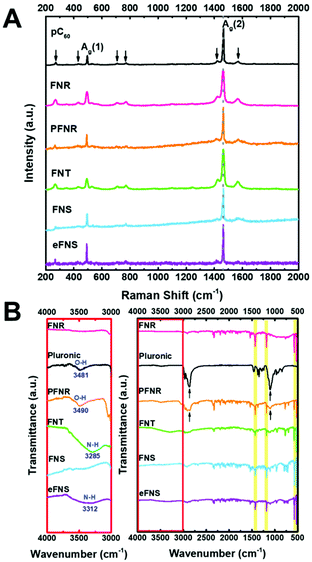 |
| Fig. 2 Characterization of the self-assembled fullerenes: (A) Raman spectra and (B) FT-IR of the self-assembled fullerenes before and after selective etching and Pluronic treatment. | |
The ATR-FTIR spectra of PNRs, PFNRs, FNTs, FNSs, and eFNSs in Fig. 2B showed four major peaks at 526, 575, 1181, and 1428 cm−1,26 confirming that C60 molecules were the main ingredient in these samples. Pluronic had two peak positions at 1103 and 2870 cm−1,27 and these two peaks were also observed in PFNRs, which indicated that the coating of Pluronic on FNRs was successful. In order to check the surface functional group of hydrophilic fullerene assemblies, the spectra in the range of 3000–4000 cm−1 were magnified. O–H groups and N–H groups were visible in the magnified spectra. The zeta potentials of FNTs, eFNSs, and PFNRs were measured, as shown in Table S1.† Because of the same surface modification, the zeta potential of FNTs was −41.29 mV while that of eFNSs was −41.21 mV. The zeta potential of PFNRs was −34.01 mV. The aqueous stability of hydrophilic fullerene assemblies was evaluated by SEM and dynamic light scattering (DLS), as shown in Fig. S3 and Table S2.† The hydrodynamic sizes listed in Table S2† showed that eFNSs and FNTs had an approximately 80% increase in the hydrodynamic size after 3 days, possibly due to the hydration of the materials. The PFNRs on the other hand demonstrated a relatively steady hydrodynamic size with an increase of 8% only, indicating that the Pluronic coating was quite stable. Meanwhile, the SEM images in Fig. S3† revealed the actual dimension and morphology of the self-assembled structures after incubation. The size and shape revealed little change after three days. These data suggested that the fullerene nanoarchitectures were stable after incubation in aqueous solution.
2.3. The endocytosis and intracellular distribution of self-assembled fullerenes by NSCs
To evaluate the endocytic ability of the self-assembled fullerenes, the soluble fullerenes were introduced to NSCs. The morphology of NSCs was observed by inverted fluorescence microscopy and time lapse video recording. As demonstrated in Fig. S4,† only FNTs were significantly endocytosed by NSCs compared to PFNRs and eFNSs after 48 h of incubation. In addition, the real-time behavior of NSCs cultured with fullerenes (15 μg mL−1) was recorded, as shown in ESI Videos 1–3.† NSCs only took up FNTs and turned into a spindle-like morphology. Such evident morphological changes were not observed in the groups treated with PFNRs and eFNSs (Fig. 3A). Interestingly, when NSCs took up the FNTs, they demonstrated a unique morphological evolution such as a sweeper moving around the culture plate to endocytose more FNTs and keep them inside the cells (ESI Video 2†). However, the NSCs did not take up either PFNRs (ESI Video 1†) or eFNSs (ESI Video 3†). The FNTs remained inside the cells when the cells were divided into two daughter cells. The localization of the self-assembled fullerenes in cells was further examined. After 5 h of treatment with PFNRs, FNTs, or eFNSs (15 μg mL−1), the cells were fixed and stained with actin-488. As demonstrated in Fig. 3B, only the FNTs were located along the cytoskeletal actin fibers. These results verified that only FNTs were endocytosed by NSCs. Meanwhile, PFNRs and eFNSs were not endocytosed by NSCs.
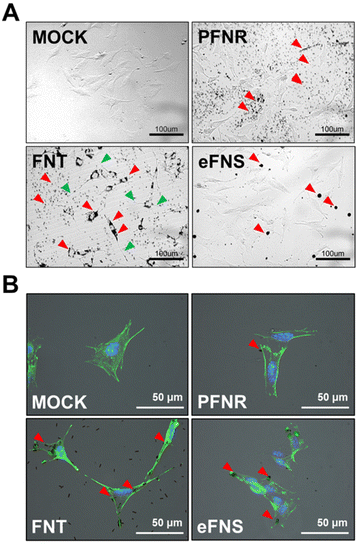 |
| Fig. 3 The localization of the self-assembled fullerenes in NSCs. (A) The time-lapse images of NSCs cultured on a tissue culture plate after adding different types of fullerene assemblies for 24 h. FNTs were endocytosed by NSCs (red arrowheads). The green arrowheads represent long spindle-like NSCs. (B) Immunofluorescence of NSCs after adding different types of fullerene assemblies for 5 h. Green: actin; blue: Hoechst nuclear staining. MOCK: the control group without any fullerenes. | |
FNTs in this study were fabricated from FNRs. Although they have similar shapes and sizes, NSCs only took up FNTs but not FNRs, suggesting that fullerenes with surface amino groups were more easily taken up than the amphiphilic polymer coated FNRs. Stem cells are usually less ready to internalize nanomaterials than mature cells. In the literature, polystyrene nanoparticles with amine groups on the surface were more easily endocytosed by mesenchymal stem cells than those without amine groups.28 Receptor-mediated endocytosis is involved in most endocytosis processes of nanoparticles.29 Meanwhile, the cells may alter their morphology upon interaction with submicron particles, including the formation of lamellipodia and filopodial extension.30 In stem cells, such morphological changes may influence their fate.31 In the current study, NSCs demonstrated an obvious extended morphology during the interaction with FNTs. Such a morphology was neuron-like or oligodendrocyte-like. This phenomenon was not as evident as that of NSCs upon exposure to other fullerene structures such as eFNSs and PFNRs.
2.4. Cell vitality, proliferation, and differentiation of NSCs with assembled fullerenes
The viability of NSCs after treatment with an FNT or eFNS fullerene was evaluated by the VB-48 staining assay, as displayed in Fig. 4A. The glutathione (GSH) levels were represented by the VB-48 intensities for NSCs after the treatment with FNTs or eFNSs. The images of the cytometric analyses revealed that the GSH levels (healthy cells) in the FNT and eFNS exposed groups were nearly the same as that in the control group. These results indicated that introducing an FNT or eFNS fullerene to NSCs did not lead to the generation of excess ROS or reduced the cell vitality neither. In the literature, the shapes and sizes of nanomaterials both affect the outcome of endocytosis, possibly implicating the size selectivity of endocytosis.32 When the nanomaterials are taken up by cells, they may cause immediate or long-term toxicity to cells through the generation of free radicals.33 Fullerenes are well known for their free radical scavenging effects and neuroprotective effects on the central nervous system.34 Our study showed that FNTs did not affect the viability of NSCs. This may be associated with the free radical scavenging ability of C60, which eliminates the possible adverse effect (extra free radical generation) while interacting with cells. This non-cytotoxicity is favorable for self-assembled fullerene structures to serve as drug or gene delivery vehicles.
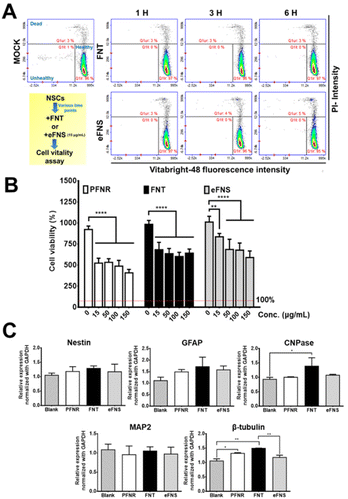 |
| Fig. 4 Survival, proliferation, and differentiation of NSCs in the presence of fullerene structures. (A) The vitality of NSCs was analyzed by the Vitabright-48 staining assay after exposure to various fullerene self-assemblies for 1, 3, and 6 h. (B) Cell viability of NSCs after culture with fullerene self-assemblies for 48 h, measured by a CCK-8 assay. (C) Gene expression of NSCs after culture with PFNRs, FNTs, and eFNSs (15 μg mL−1) for 7 days. Blank group: NSCs without fullerene treatment. Asterisks indicate statistically significant differences, *p < 0.05 and **p < 0.01. | |
The cell viability and possible proliferation were confirmed by a CCK-8 assay, as shown in Fig. 4B. After 48 h, the cell viabilities were ∼680% and ∼980% for FNTs (15 μg mL−1) and the control group, respectively, indicating that the cells remained viable and were able to proliferate. The cell viability was ∼520% for the PFNR group (15 μg mL−1) at 48 h. On the other hand, the cell viability in the eFNS group (15 μg mL−1) was ∼830% at 48 h. These results indicated that the proliferation of NSCs was slightly affected by FNTs. An earlier study reported that the C60 nanofilm reduced the cell proliferation of liver cancer cell lines.35 The random C60 nanowhisker substrates offered the nearby NSCs better viability than the aligned C60 nanowhiskers.13 In the current study, viability analyses of NSCs showed that all fullerene self-assembled structures could reduce the proliferation rate of NSCs.
The gene expression was assessed to verify the cell differentiation ability of NSCs in the presence of PFNRs, FNTs, or eFNSs (15 μg mL−1). The expression of typical markers for neural differentiation, including Nestin (neural stemness marker), GFAP (glial cell marker), CNPase (oligodendrocyte marker), MAP2 (mature neuronal marker), and β-tubulin (early neuronal marker), is shown in Fig. 4C. After 7 days, the expression levels of CNPase and β-tubulin in the FNT group (15 μg mL−1) were significantly increased by ∼1.5-fold as compared to the control group. As shown in Fig. S5 and S6,† the protein levels of CNPase and β-tubulin in the FNT group also slightly increased compared to the control group, as determined by immunofluorescence and flow cytometry. There were no statistically significant changes in the gene expression in the eFNS group, while a small increase of β-tubulin was observed in the PFNR group. These data confirmed that FNTs facilitated the survival (ROS scavenging) and differentiation of NSCs.
The obvious morphological difference mentioned earlier for NSCs exposed to FNTs may account for the greater expression of neural differentiation-related genes in the FNT group compared to the eFNS, PFNR, and control groups. The finding also suggested that the morphological changes of NSCs upon interaction with FNTs may promote the fate of NSCs to differentiate into neurons or oligodendrocytes. In the literature, nanowhiskers, the self-assembled form of C60 with a length of hundreds of microns, had the effect of aligning neural stem cells, thereby inducing neuronal differentiation.13 In comparison, the non-oriented arrangement of FNTs with a length of several microns may not align cells but instead cause cytoskeletal changes to promote the neural differentiation of NSCs. Unlike the nanowhiskers where the length and hydrophilicity of the nanowhiskers limit their applications to a 3D culture, FNTs can be dispersed in hydrogels for further testing the influence in a 3D environment.
Many hydrophilic nanocarbons have been investigated for their effect on cells. Carbon nanotubes (CNTs) possess unique electrical, structural, and mechanical properties, and a diversity of available surface chemistries, and cell-penetrating capabilities, which allow them to serve as implantable matrices or carriers to deliver therapeutic molecules.36 However, the toxicity of CNTs limits the clinical application mainly for two reasons. First, impurities (such as metal catalysts) remaining in the CNT manufacturing process may cause cell inflammation. Second, CNTs have a high length-to-diameter ratio, so they may pierce through cell membranes because of their long length and short diameter.36
2.5. Preparation of chitosan self-healing hydrogels and the composite hydrogels with self-assembled fullerenes
The schematic for preparing 3D chitosan self-healing hydrogels (CS hydrogels) is illustrated in Fig. 5(A and B) and that for preparing composite hydrogels with self-assembled fullerenes is shown in Fig. 5C. The amine group on glycol chitosan reacted with benzaldehydes at both ends of difunctionalized PEG (DF-PEG) to form a dynamic crosslinked network of self-healing hydrogels. As shown in Fig. 5C, after ethylene diamine (EDA) selective etching, the amine group was generated on self-assembled fullerenes, which also had the opportunity to react with the crosslinker in the composite hydrogels. The rheological properties of chitosan self-healing hydrogels and the composite hydrogels with self-assembled fullerenes are displayed in Fig. 5(D and E). The time-dependent changes of dynamic moduli (G′ and G′′) revealed that the composite hydrogels had similar rheological properties to the original hydrogel.
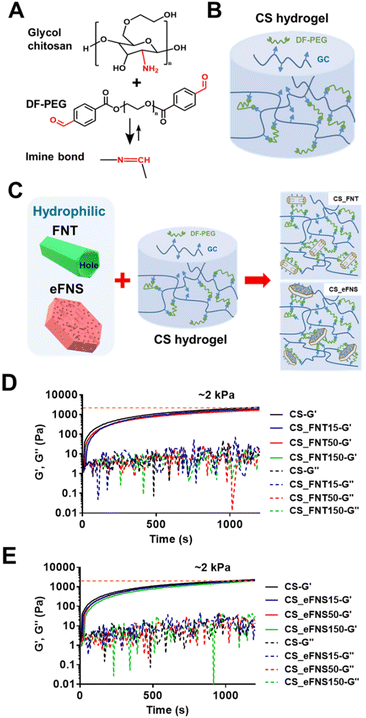 |
| Fig. 5 Preparation of chitosan self-healing hydrogels and composite hydrogels with self-assembled fullerenes. (A and B) Schematic illustration of benzaldehydes at both ends of difunctionalized PEG (DF-PEG) crosslinking with the amine group on glycol chitosan to form self-healing hydrogels (CS hydrogels). (C) The diagram of making composite hydrogels from self-assembled fullerenes of different nanoarchitectured forms. (D and E) Rheological properties of chitosan self-healing hydrogels and composite hydrogels with self-assembled fullerenes, shown as the time-dependent changes of modulus (G′ and G′′) for self-healing hydrogels and the composite hydrogels at 37 °C after mixing. | |
In addition, FNTs, eFNSs, and PFNRs at the same concentration were added to the CS hydrogels to form composite hydrogels. After liquid nitrogen lyophilization, slight differences in the microstructure of the hydrogels were observed by SEM, as shown in Fig. S7.† The hole shapes were different in the composite hydrogels. The CS_FNT composite hydrogel had columnar holes and the aspect ratio was around two. The CS_FNS composite hydrogel had layered holes. The CS_FNS composite hydrogel had an irregular shape. The CS_PFNR composite hydrogel had spheroidal holes of uniform size.
2.6. Injectable and self-healing properties of chitosan fullerene composite self-healing hydrogels
The injectability test showed that the concentration of 15 μg mL−1 FNTs in the composite hydrogel did not affect the injectability of the hydrogel through a 30G syringe needle (Fig. 6A). As shown in Fig. 6B, the gross observation of the self-healing property of the CS_FNT hydrogel at room temperature was examined on disc-shaped CS_FNT hydrogels with a central hole. The central cavity in the gel healed after 24 h. There was no significant difference between the healing speed of CS and CS_FNT hydrogel groups.
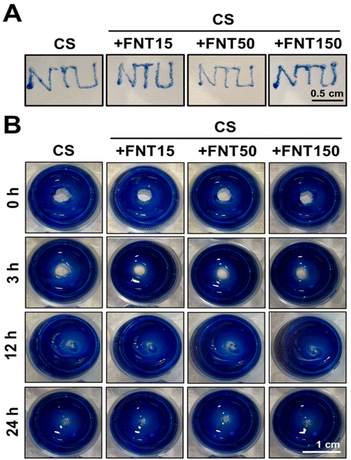 |
| Fig. 6 Injectability and self-healing properties of chitosan self-healing composite hydrogels. (A) Injection of CS self-healing hydrogels and the composite hydrogels to pass through a 30-gauge needle. (B) Images of the self-healing ability of the CS hydrogels with a central hole after 0, 3, 12, and 24 h. CS: chitosan self-healing hydrogel. For composite hydrogels, +FNT15 represents the composite hydrogel containing 15 μg mL−1 FNTs, +FNT50 represents that containing 50 μg mL−1 FNTs, and so forth. | |
The self-healing hydrogel, based on glycol chitosan and the Schiff crosslinker DF-PEG, has the advantages of injectability and excellent self-healing.22 These properties were important for the survival and differentiation of neural progenitors encapsulated.17 In the current study, the rheological properties and self-healing behavior of the composite hydrogel were not adversely affected by the addition of hydrophilic fullerenes, and the injectability was neither sacrificed. Since hydrophilic fullerenes have crosslinkable amino groups on the surface,14 the amino groups can also interact with the crosslinker DF-PEG of the hydrogel network, as supported by Fig. S8.† This may account for the maintenance of a soft modulus and self-healing properties of the composite hydrogel. Meanwhile, as the content of fullerenes increases, the injectability of the composite hydrogel may be reduced. When the concentration was 150 μg mL−1, the composite hydrogel was hindered from passing through a 30G syringe needle.
2.7. Survival, proliferation, and differentiation of NSCs in the composite hydrogel
The morphology of NSCs in CS_FNT hydrogels is shown in Fig. 7A. To assess if the composite hydrogels supported long-term cell survival, the cell growth was measured in a 3D culture for 3 days. The data are presented in Fig. 7B. The cells in the pristine CS hydrogel served as the control (100%). After 3 days, the cell viability increased to 268%, 298%, and 315% in CS, CS_FNT15 and CS_FNT150 hydrogels, respectively, suggesting that the cell viability was enhanced in the composite hydrogels.
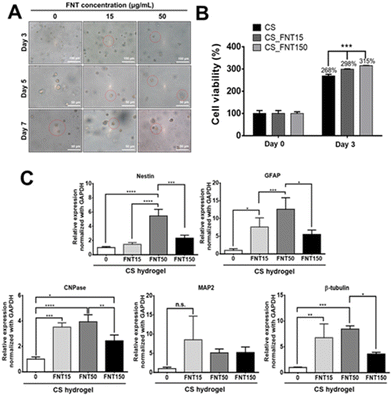 |
| Fig. 7 Morphology, proliferation, and gene expression of NSCs encapsulated in different composite hydrogels. (A) Observation of the cell surface in different composite hydrogels at 3, 5, and 7 days. (B) Proliferation of NSCs in different composite hydrogels. CS represents the pristine chitosan self-healing hydrogel. CS_FNT15 represents the composite hydrogel containing 15 μg mL−1 FNTs, CS_FNT50 represents that containing 50 μg mL−1 FNTs, and so forth. (C) The gene expression of NSCs encapsulated in different composite hydrogels for 3 days. Asterisks indicate statistically significant differences, *p < 0.05, **p < 0.01, ***p < 0.001, and ****p < 0.0001. n.s. represents not significant. | |
The effect on the neuronal differentiation behavior is shown in Fig. 7C. The expression levels of specific neural markers for NSCs in different composite hydrogels after 3 days of incubation were analysed. The gene expression levels of GFAP, CNPase, and β-tubulin were increased for NSCs in the composite hydrogel with a low concentration of FNTs (15 μg mL−1). Meanwhile, the gene expression levels of Nestin, GFAP, CNPase, and β-tubulin genes were significantly upregulated for NSCs in the CS_FNT50 group, but significantly decreased in the CS_FNT150 group. These data suggested that 50 μg mL−1 FNTs in the CSF hydrogel was adequate for the 3D culture of neural stem cells. Besides, these findings were consistent with the report regarding the fact that CNPase mediates strong tubulin polymerization dimers to form microtubule and polymeric tubulin structures.37 CNPase is an essential component of the cells’ cytoskeletal machinery that directs process outgrowth in oligodendrocytes.37 In our research, NSCs expressed increased CNPase and β-tubulin gene expression levels after treatment with a low concentration of FNTs for 7 days (Fig. 4C). In addition, the FNT composite hydrogel exhibited significantly higher expression levels of CNPase and β-tubulin genes, suggesting more differentiation towards oligodendrocytes and neurons.
3. Experimental section
3.1. Preparation and characterization of hydrophobic or hydrophilic self-assembled fullerenes
For the preparation of self-assembled fullerene nanostructures, the liquid–liquid interfacial precipitation (LLIP) method was employed. Hydrophobic fullerene nanorods (FNRs) and nanosheets (FNSs) were obtained through different crystallization methods at the interface by mixing different dissolved concentrations of C60 (99.5% pure, MTR Ltd, USA) in different aromatic solvents with non-solvent alcohols.14 After that, using EDA as a selective etchant, two hydrophilic fullerene self-assembled nanostructures were obtained, which were etched fullerene nanotubes (FNTs) and etched fullerene nanosheets (eFNSs). Briefly, C60 powder was completely dissolved in m-xylene. For C60 crystallization to form FNRs, the C60 solution was placed in a clean glass bottle, with the addition of the non-solvent isopropyl alcohol (IPA). To improve the surface hydrophilicity of FNRs, EDA was added to the FNR mixture and sonicated for 10 min at room temperature to achieve selective etching. The solution was then centrifuged and re-added to the same concentration and volume of the etchant and sonicated. After washing three times, hydrophilic FNTs were obtained. FNSs were prepared by simply changing the aromatic solvent of C60 from m-xylene to p-xylene. The preparation method of eFNSs followed a similar procedure to that of FNTs by EDA selective etching. The experimental details of the previous paper were followed. Pluronic FNRs (PFNRs) were obtained by adding FNRs into 0.5 wt% Pluronic (P123, polyethylene glycol-polypropylene glycol-polyethylene glycol (PEG-PPG-PEG), Mn ∼ 5800, Sigma-Aldrich, USA) aqueous solution with resuspension overnight. After centrifuging and removing the supernatant, the precipitate was washed with ultrapure water. After repeating the above step twice, the precipitate was vacuum dried for 24 h at 80 °C.
The morphologies of FNRs, FNTs, FNSs, eFNSs and PFNRs were characterized using a Hitachi Model S-4800 field emission scanning electron microscope (FE-SEM). The equipment was operated at an accelerating voltage of 10 kV. Prior to the measurement, fullerene samples were dropped onto a passivated silicon substrate and dried. The SEM samples were then coated with a layer of about 2 nm platinum metal using a Hitachi S-2030 ion coater.
Surface functional groups were identified by attenuated total reflection-Fourier transform infrared (ATR-FTIR, Nexus 670, Japan) spectroscopy. The Raman spectra of these self-assembled fullerenes were recorded using a Raman spectrometer (NRS-3100, JASCO, Japan). The equipment was operated at 0.01 mW power with a 514.5 nm laser. The zeta potential and hydrodynamic diameters of self-assembled fullerenes were measured using a particle-size analyzer (ELSZ-2000ZS, Otsuka Electronics Co., Ltd, Japan).
3.2. Culture of neural stem cells (NSCs)
NSCs were obtained from adult mouse brain and transfected with the promoter F1B-green fluorescence protein (F1B-GFP) for purification of the NSCs.38,39 The NSCs were cultured in 1
:
1 HG-DMEM and Ham's F-12 (DMEM/F12, Gibco, USA) supplemented with 10% fetal bovine serum (Gibco, USA), 400 μg mL−1 G418 (Gibco, Thailand), and 1% penicillin-streptomycin (Gibco, USA) at 37 °C under a humidified atmosphere containing 5% CO2. The culture media were refreshed every 2 days.
3.3. Cellular uptake studies
To study the endocytic ability of NSCs, a time-lapse recording system was used to track the NSCs incubated with fullerenes. NSCs (3000 cells per well) were incubated with PFNRs, FNTs, and eFNSs (15 μg mL−1) for 24 h in a 37 °C incubator.
The localization of fullerenes in the cytosol was determined by staining using a cellular cytoskeletal actin-488 antibody. NSCs (3000 cells per well) were seeded on a cover slide overnight. Then, the cells were incubated with PFNRs, FNTs, and eFNSs (15 μg mL−1) at 37 °C. After 5 h, the cells were fixed in 4% paraformaldehyde for 20 min. Next, the cells were washed with PBS and permeabilized with 0.1% Triton X-100 for 15 min. Then, the samples were blocked with 0.5% BSA for 1 h at room temperature, followed by incubation overnight at 4 °C with actin-488 (Proteintech, USA) antibodies. Afterwards, the cells were counterstained with Hoechst 33258 (the fluorescent nuclear stain, Sigma-Aldrich) and visualized using a fluorescence microscope (Leica, DM IRB).
3.4. Cell vitality and proliferation of NSCs in the presence of assembled fullerenes
The reactive oxygen species (ROS) activity of NSCs exposed to the self-assembled fullerenes was determined by a cell vitality assay using an advanced image cytometer (NucleoCounter NC-3000, ChemoMetec). The cells (1 × 105 cells per well) were grown in 24 well plates overnight and treated with 15 μg mL−1 fullerenes for 1, 3, and 6 h. Then, the cells were washed three times with phosphate-buffered saline (PBS). 250 μL of trypsin was added to dissociate the cells, which were collected by centrifugation. The cell pellet was re-suspended with 50 μL of culture medium. Next, 19 μL of cell suspension was stained with 1 μL of Solution 5 (ChemoMetec) according to the manufacturer's protocol. Solution 5 contained three types of fluorescent stains. Propidium iodide stained the dead cells, acridine orange served as a fluorescent counterstain, and VitaBright-48 (VB-48) represented the level of thiols such as reduced glutathione in the cells. Finally, 10 μL of stained cells were loaded with an 8-chamber NC-Slide A8™ and analyzed using the image cytometer system.
The proliferation rates of NSCs treated with PFNR, FNT, and eFNS self-assembled fullerenes were further assessed by Cell Counting Kit-8 (CCK-8, Sigma). Briefly, NSCs (5000 cells per well) were plated in the wells of a 96-well culture plate for 24 h, and the medium was incubated to a culture medium with 5 μL of the CCK-8 solution and 95 μL of the culture medium according to the manufacturer's instructions. The first absorbance (ODinitial) was measured at 450 nm using a microplate reader (SpectraMax iD3, Molecular Devices, USA). Next, the culture medium was replaced with several concentrations of PFNRs, FNTs, and eFNSs in a culture medium for 48 h. After that, the cells were washed with PBS twice to eliminate fullerenes and incubated with CCK-8 solution. The second absorbance optical density (ODfinal) was obtained. The cell viability was determined as (ODfinal/ODinitial) × 100%, which was the percentage relative to the initial value.
Next, the proliferation rates of NSCs in composite hydrogels containing fullerenes of different concentrations (15, 50, and 150 μg mL−1) were also analyzed by a CCK-8 assay. Briefly, composite hydrogels (0.3 mL) were mixed with NSCs (density 2 × 106 cells per mL) at 37 °C. In each well of the 24-well plate, 0.3 mL of hydrogels and 2 mL of medium were added. After 3 days of incubation, the cell proliferation in the composite hydrogels was evaluated by a CCK-8 assay and analyzed using a microplate reader as mentioned above.
3.5. Gene expression of neural-related markers of NSCs
Total RNA was extracted from samples using the TRIzol® reagent (Invitrogen, USA) and chloroform after the NSC-laden composite hydrogels were incubated for 3 days. The RNA strand was transcribed reversely into cDNA and amplified using a RevertAid H minus First Strand cDNA Synthesis kit (Thermo Fisher, USA). Real-time reverse transcription-polymerase chain reaction (qRT-PCR) was performed by utilizing a KAPA SYBR Green qPCR Kit (Biosystem), consisting of denaturation at 95 °C for 10 min, annealing and extension for 30 s at 60 °C. PCR was operated using a StepOne Plus Real-Time PCR instrument (Applied Biosystems, USA). The expression levels were normalized to the housekeeping gene glyceraldehyde 3-phosphate dehydrogenase (GAPDH) and then represented by the relative ratio of gene expression. The primer sequences for each gene used in this study are shown in Table S3.†
3.6. Preparation of chitosan self-healing hydrogels and the composite hydrogels with self-assembled fullerenes
Chitosan self-healing hydrogels (CS hydrogels) with a dynamic Schiff-base were formed by mixing 1.5 wt% glycol chitosan (Wako, 410 kDa, deacetylation degree 78.2%) and 1 wt% crosslinker solution of telechelic difunctional polyethylene glycol (DF-PEG) at room temperature. DF-PEG was synthesized through the Steglich esterification reaction of PEG (Sigma-Aldrich, 4000 kDa) and 4-formylbenzoic acid (Sigma-Aldrich) to form telechelic aldehyde groups.40 The ratio of PEG telechelic substitution to the aldehyde group was evaluated by NMR. In the preparation of composite hydrogels, different self-assembled fullerene nanomaterials were added to the CS hydrogels (2.5 wt% solid content) to contain 15, 50, or 150 μg mL−1 self-assembled fullerenes in the final hydrogels.
3.7. Rheological properties and morphologies of self-healing hydrogels and composite hydrogels
The viscoelastic properties of composite hydrogels were investigated using a rheometer (RS-5, TA Instruments) with a cone-plate geometry immediately after loading the composite hydrogel samples (0.8 mL) on the platform. The samples were maintained at 37 °C for measurement. The storage shear modulus (G′) and loss shear modulus (G′′) were investigated against time (0–1800 s) at a constant frequency of 1 Hz and 1% oscillatory strain (time sweep). The morphology of the composite hydrogels was examined by SEM after the hydrogels were freeze-dried.
3.8. Statistical analysis
The experimental data were represented by the mean and standard deviation. The reproducibility of each experiment was independently confirmed three times. The statistical difference between the experimental groups was determined by one-way ANOVA. A statistically significant difference was represented by the values of *p < 0.05, **p < 0.01, ***p < 0.001, and ****p < 0.0001, and “n.s.” represents not significant.
4. Conclusion
The unique hydrophilic modification process provides a good opportunity to study the biomedical properties of such fullerene nanostructures in cell culture. The endocytic activity with low toxicity of neural stem cells was observed in the 2D culture. The unique π–π interaction and one-dimensional structure of FNTs appeared to effectively guide the extension and elongation of neural stem cells, and a neuron-like morphology was observed in the 2D culture. Combining self-healing hydrogels and self-assembled C60 fullerenes may have potential benefits for neural tissue engineering. Hydrogels provide the structural support and the soft 3D microenvironment essential for the development of neural cells and tissues. Through the combined effect of mechanically soft hydrogels and geometrically elongated fullerene nanostructures, significant differentiation of NSCs was observed, which was associated with their morphological changes. Hydrophilic self-assembled fullerenes of sheet shapes also exerted a differentiation effect on neural stem cells, suggesting that fullerene chemistry in addition to the geometric effect also played a role in changing cell behavior. We believed that soft hydrogels and fullerenes provided distinct and complementary environmental cues. Therefore, the combined use of controllable self-assembled fullerenes and self-healing hydrogels may offer a more effective approach to controlling stem cell behavior in a 3D biomimetic microenvironment.
Author contributions
Chui-Wei Wong and Kun-Che Tsai: conceptualization, methodology, investigation, visualization, and writing – original draft. Lok Kumar Shrestha and Katsuhiko Ariga: conceptualization, investigation, and supervision. Shan-hui Hsu: conceptualization, investigation, writing – partial original draft, final review & editing, and supervision.
Conflicts of interest
There are no conflicts to declare.
Acknowledgements
This work was supported by the Dragon Gate Program, the Ministry of Science and Technology, Taiwan, R.O.C. (MOST 109-2926-I-002-511 and MOST 110-2926-I-002-505). This study was partially supported by JSPS KAKENHI grant number JP20H00392, JP20H00316, JP21H04685, JP21F50068, and JP20K05590. Neural stem cells were kindly supplied by Dr Ing-Ming Chiu, National Health Research Institutes. The DLS and zeta potential measurements were kindly assisted by Dr Daniel T. Payne, International Center for Young Scientists, National Institute for Materials Science (NIMS).
References
- S. Subramoney, Adv. Mater., 1998, 10, 1157–1171 CrossRef CAS.
- P. J. Krusic, E. Wasserman, P. N. Keizer, J. R. Morton and K. F. Preston, Science, 1991, 254, 1183–1185 CrossRef CAS PubMed.
- S. Bosi, T. Da Ros, G. Spalluto and M. Prato, Eur. J. Med. Chem., 2003, 38, 913–923 CrossRef CAS PubMed.
- N. Gharbi, M. Pressac, M. Hadchouel, H. Szwarc, S. R. Wilson and F. Moussa, Nano Lett., 2005, 5, 2578–2585 CrossRef CAS PubMed.
- H. Isobe, W. Nakanishi, N. Tomita, S. Jinno, H. Okayama and E. Nakamura, Chem.– Asian J., 2006, 1, 167–175 CrossRef CAS.
- S. Goodarzi, T. Da Ros, J. Conde, F. Sefat and M. Mozafari, Mater. Today, 2017, 20, 460–480 CrossRef CAS.
- A. Astefanei, O. Nunez and M. T. Galceran, Anal. Chim. Acta, 2015, 882, 1–21 CrossRef CAS PubMed.
- L. L. Dugan, D. M. Turetsky, C. Du, D. Lobner, M. Wheeler, C. R. Almli, C. K. Shen, T. Y. Luh, D. W. Choi and T. S. Lin, Proc. Natl. Acad. Sci. U. S. A., 1997, 94, 9434–9439 CrossRef CAS PubMed.
- M. Sathish, K. Miyazawa, J. P. Hill and K. Ariga, J. Am. Chem. Soc., 2009, 131, 6372–6373 CrossRef CAS PubMed.
- L. K. Shrestha, Q. Ji, T. Mori, K. Miyazawa, Y. Yamauchi, J. P. Hill and K. Ariga, Chem.– Asian J., 2013, 8, 1662–1679 CrossRef CAS PubMed.
- C. Park, E. Yoon, M. Kawano, T. Joo and H. C. Choi, Angew. Chem., Int. Ed., 2010, 49, 9670–9675 CrossRef CAS PubMed.
- P.-W. Luo, H.-W. Han, C.-S. Yang, L. K. Shrestha, K. Ariga and S.-H. Hsu, Adv. Biosyst., 2019, 3, 201800254 Search PubMed.
- F. Y. Hsieh, L. K. Shrestha, K. Ariga and S. H. Hsu, Chem. Commun., 2017, 53, 11024–11027 RSC.
- C.-T. Hsieh, S.-H. Hsu, S. Maji, M. K. Chahal, J. Song, J. P. Hill, K. Ariga and L. K. Shrestha, Mater. Horiz., 2020, 7, 787–795 RSC.
- P. Zhuang, A. X. Sun, J. An, C. K. Chua and S. Y. Chew, Biomaterials, 2018, 154, 113–133 CrossRef CAS PubMed.
- V. A. Kornev, E. A. Grebenik, A. B. Solovieva, R. I. Dmitriev and P. S. Timashev, Comput. Struct. Biotechnol. J., 2018, 16, 488–502 CrossRef CAS PubMed.
- X. W. Li, E. Katsanevakis, X. Y. Liu, N. Zhang and X. J. Wen, Prog. Polym. Sci., 2012, 37, 1105–1129 CrossRef CAS.
- R. C. Cheung, T. B. Ng, J. H. Wong and W. Y. Chan, Mar. Drugs, 2015, 13, 5156–5186 CrossRef CAS PubMed.
- Y. S. Li, X. Wang, Y. Wei and L. Tao, Chin. Chem. Lett., 2017, 28, 2053–2057 CrossRef CAS.
- S. Lin, N. Sangaj, T. Razafiarison, C. Zhang and S. Varghese, Pharm. Res., 2011, 28, 1422–1430 CrossRef CAS PubMed.
- F. Y. Hsieh, H. H. Lin and S. H. Hsu, Biomaterials, 2015, 71, 48–57 CrossRef CAS PubMed.
- T. C. Tseng, L. Tao, F. Y. Hsieh, Y. Wei, I. M. Chiu and S. H. Hsu, Adv. Mater., 2015, 27, 3518–3524 CrossRef CAS PubMed.
- F. Y. Hsieh, H. W. Han, X. R. Chen, C. S. Yang, Y. Wei and S. H. Hsu, Biomaterials, 2018, 174, 31–40 CrossRef CAS PubMed.
- F. Gattazzo, A. Urciuolo and P. Bonaldo, Biochim. Biophys. Acta, 2014, 1840, 2506–2519 CrossRef CAS PubMed.
- H. Kuzmany, R. Pfeiffer, M. Hulman and C. Kramberger, Philos. Trans. R. Soc., A, 2004, 362, 2375–2406 CrossRef CAS PubMed.
- R. G. Shrestha, L. K. Shrestha, A. H. Khan, G. S. Kumar, S. Acharya and K. Ariga, ACS Appl. Mater. Interfaces, 2014, 6, 15597–15603 CrossRef CAS PubMed.
- J. D. A. Rodrigues and R. S. V. Nascimento, J. Appl. Polym. Sci., 2010, 116, 3047–3055 CrossRef.
- X. E. Jiang, J. Dausend, M. Hafner, A. Musyanovych, C. Rocker, K. Landfester, V. Mailander and G. U. Nienhaus, Biomacromolecules, 2010, 11, 748–753 CrossRef CAS.
- D. Manzanares and V. Cena, Pharmaceutics, 2020, 12, 371 CrossRef CAS PubMed.
- K. Anselme, P. Davidson, A. M. Popa, M. Giazzon, M. Liley and L. Ploux, Acta Biomater., 2010, 6, 3824–3846 CrossRef CAS PubMed.
- M. J. Dalby, N. Gadegaard and R. O. Oreffo, Nat. Mater., 2014, 13, 558–569 CrossRef CAS PubMed.
- R. Vacha, F. J. Martinez-Veracoechea and D. Frenkel, Nano Lett., 2011, 11, 5391–5395 CrossRef CAS PubMed.
- S. W. Shin, I. H. Song and S. H. Um, Nanomaterials, 2015, 5, 1351–1365 CrossRef CAS PubMed.
- K. Ariga, X. Jia, J. Song, C. T. Hsieh and S. H. Hsu, ChemNanoMat, 2019, 5, 692–702 CrossRef CAS.
- M. Sosnowska, M. Kutwin, S. Jaworski, B. Strojny, M. Wierzbicki, J. Szczepaniak, M. Łojkowski, W. Święszkowski, J. Bałaban and A. Chwalibog, Int. J. Nanomed., 2019, 14, 6197 CrossRef CAS PubMed.
- P. M. Costa, M. Bourgognon, J. T. Wang and K. T. Al-Jamal, J. Controlled Release, 2016, 241, 200–219 CrossRef CAS PubMed.
- J. Lee, M. Gravel, R. Zhang, P. Thibault and P. E. Braun, J. Cell Biol., 2005, 170, 661–673 CrossRef CAS PubMed.
- Y. C. Hsu, D. C. Lee, S. L. Chen, W. C. Liao, J. W. Lin, W. T. Chiu and I. M. Chiu, Dev. Dyn., 2009, 238, 302–314 CrossRef CAS PubMed.
- I. M. Chiu, K. Touhalisky, Y. Liu, A. Yates and A. Frostholm, Oncogene, 2000, 19, 6229–6239 CrossRef CAS PubMed.
- M. Liu, X. Zeng, C. Ma, H. Yi, Z. Ali, X. Mou, S. Li, Y. Deng and N. He, Bone Res., 2017, 5, 1–20 Search PubMed.
|
This journal is © The Royal Society of Chemistry 2022 |