DOI:
10.1039/D2NR01710E
(Paper)
Nanoscale, 2022,
14, 8306-8317
Enhanced photocatalytic and antimicrobial performance of a multifunctional Cu-loaded nanocomposite under UV light: theoretical and experimental study†
Received
29th March 2022
, Accepted 17th May 2022
First published on 18th May 2022
Abstract
Due to modern industrialization and population growth, access to clean water has become a global challenge. In this study, a metal–semiconductor heterojunction was constructed between Cu NPs and the Co0.5Ni0.5Fe2O4/SiO2/TiO2 composite matrix for the photodegradation of potassium permanganate, hexavalent chromium Cr(VI) and p-nitroaniline (pNA) under UV light. In addition, the electronic and adsorption properties after Cu loading were evaluated using density functional theory (DFT) calculations. Moreover, the antimicrobial properties of the prepared samples toward pathogenic bacteria and unicellular fungi were investigated. Photocatalytic measurements show the outstanding efficiency of the Cu-loaded nanocomposite compared to that of bare Cu NPs and the composite matrix. Degradation efficiencies of 44% after 80 min, 100% after 60 min, and 65% after 90 min were obtained against potassium permanganate, Cr(VI), and pNA, respectively. Similarly, the antimicrobial evaluation showed high ZOI, lower MIC, higher protein leakage amount, and cell lysis of nearly all microbes treated with the Cu-loaded nanocomposite.
1. Introduction
In pursuit of a modern industrial revolution, pharmaceuticals, agricultural waste, and hazardous chemical compounds are produced. To deal with these environmental pollutants, waste disposal and management have become significant global challenges.1 Some of the hazardous compounds that contaminate water, soil, and the environment are highly toxic and have the potential to cause serious health problems; these pollutants include potassium permanganate, chromium ions, and 4-nitroaniline or p-nitroaniline (pNA). Potassium permanganate is an inorganic dye that is used in many applications, including water treatment.2 However, its existence in potable water may cause many chronic health problems, such as liver damage and skin irritation.3 Chromium exists in water either in trivalent or hexavalent oxidation states, and the hexavalent state has raised concerns due to its high toxicity.4 Moreover, nitroaromatic compounds, such as pNA, are major water-soluble noxious pollutants that have been classified as priority contaminants by the U.S. Environmental Protection Agency.5 In addition to this, millions of people die every year from diseases caused by waterborne pathogens (bacteria and fungi), including diarrhea, typhoid fever, and hepatitis A.6 Recently, these microorganisms have begun to show resistance toward all currently known antibiotics, which poses a real danger to humans and marine life.7
The aforementioned pollutants are detected in wastewater and are detrimental to aquatic organisms and may lead to long-term pollution of the environment. Therefore, eliminating these compounds from industrial wastewater is of particular importance. Many wastewater purification techniques are available, such as coagulation, flocculation, adsorption, ultra-filtration, oxidation, and membrane methods.8 However, these techniques have many limitations as a result of their high cost, complexity, and time-consuming processes. Consequently, particulate photocatalysis has received much attention due to its outstanding photocatalytic characteristics, which include the direct conversion of light energy into chemical energy via a series of redox reactions that occur on the surface of a material known as a photocatalyst. Heterogeneous photocatalysis using semiconductor-based nanocomposites is important because it combines the effects of different nanoparticles.
Titania (TiO2)-based nanocomposites possess desirable advantages, such as cost-effectiveness, extraordinary efficiency, unique stability, and excellent reproducibility. The photocatalytic properties of TiO2-based photocatalysts have been assessed in the degradation of many organic compounds, with the majority of them displaying superior photocatalytic performance toward several pollutants under different conditions.9 However, TiO2-based photocatalysts demonstrate remarkable photocatalytic activity only under UV light due to their wide bandgap energy (3.2 eV for anatase TiO2).10 In addition, the rapid recombination process of electron–hole pairs on the surface of TiO2-based photocatalysts is another serious limitation that hinders their vast applications in water remediation. Thus, to enhance the performance of TiO2-based photocatalysts, many tailoring methods have been carried out. Among them, the construction of a metal–semiconductor heterojunction is a promising route due to the efficient charge separation and increase in the redox reaction time.11 The decoration of TiO2 photocatalysts with carbon nanomaterials or noble metal particles, such as gold (Au), silver (Ag), and platinum (Pt), has been reported to enhance their photocatalytic performance.12 Some of these noble metals (Au–Ag) also act as co-catalysts to improve the absorption of photocatalysts in the visible light range.13 However, the utilization of such metals is limited due to their extraordinary cost, toxicity, and low natural abundance. In the same context, copper (Cu) is more abundant, cost effective, and eco-friendly, exhibits photocatalytic properties, and can be employed as a co-catalyst to prevent rapid electron–hole recombination.14
To further increase the efficiency of TiO2 NPs, the utilization of a SiO2 matrix to enhance the absorption capability of TiO2 and to provide a framework for the dispersion of TiO2 particles has been studied. Excellent adsorption capability has been shown to improve the photoactivity of a TiO2/SiO2 system.15 At the same time, the uniform distribution of TiO2 NPs onto a SiO2 matrix has been shown to lead to an enhancement in the composite specific surface area,16,17 as well as the increased thermal stability of TiO2 NPs.
In heterogeneous photocatalysis, it is difficult to separate the employed photocatalyst from wastewater streams. Consequently, more interest has been directed toward the utility of nanocomposites with magnetic cores for water treatment applications, as these can be readily removed from water using a magnet.18 Among these magnetic materials, spinel nickel–cobalt ferrite (NiCoFe2O4) has been extensively used in diverse applications, such as gas sensing, water desalination, energy storage, radar, and stealth applications,19 due to its excellent chemical and thermal stability, as well as its superparamagnetism.20 Besides this, it exhibits excellent permeability, significant saturation magnetization, and soft coercivity. These properties are essential for exploring its feasibility in several possible industrial and environmental applications.21
Herein, the optimized preparation of a recyclable, magnetic Co0.5Ni0.5Fe2O4/SiO2/TiO2 (CNFST) nanocomposite matrix via a layer-by-layer method is reported. Cu NPs were loaded onto the external surface of the prepared matrix (TiO2 layer) to construct a metal–semiconductor heterojunction of the Co0.5Ni0.5Fe2O4/SiO2/TiO2/Cu 10% (CNFST/Cu 10%) enhanced photocatalyst. The photocatalytic properties of the prepared photocatalyst were examined in the photodegradation of aqueous solutions containing three different types of pollutants (potassium permanganate (an inorganic dye), hexavalent chromium (a heavy metal), and p-nitroaniline (pNA, an organic dye)) under UV-light radiation. In addition, the electronic and adsorption properties of the photocatalyst were analyzed via density functional theory (DFT) analysis. Finally, the antimicrobial activities of the prepared photocatalyst were evaluated against many multi-drug-resistant bacteria and pathogenic fungi and the results were compared to those of standard antibacterial and antifungal agents.
2. Materials and methods
2.1 Materials
The starting reagents copper acetate monohydrate ((C4H8CuO5)·H2O), ascorbic acid (C6H8O6), hydroxypropyl cellulose (molecular weight = 80
000), ammonium hydroxide 28% (NH4OH), sodium hydroxide (NaOH), cobalt chloride (CoCl2), titanium(IV) isopropoxide 97% (Ti(OC3H7)4), nickel chloride (NiCl2), hydrochloric acid (HCl), ferric chloride (FeCl3·6H2O), absolute ethanol 99.9% (C2H5OH), tetraethyl orthosilicate 98% (TEOS) (Si·(OC2H5)4), ethylenediaminetetraacetic acid (C10H16N2O8, EDTA), sulfuric acid (H2SO4), potassium permanganate (KMnO4), potassium dichromate (K2Cr2O7), and pNA (C6H6N2O2) were purchased from Sigma Aldrich-Germany, Wako-Japan and Merck-USA. Chemicals were of extra pure grade and used as received.
2.2 Methods
2.2.1 Preparation of the composite matrix (Co0.5Ni0.5Fe2O4/SiO2/TiO2), Cu NPs and Cu-loaded nanocomposite (Co0.5Ni0.5Fe2O4/SiO2/TiO2/Cu).
Detailed preparation steps of the composite matrix (CNFST), Cu NPs, and CNFST/Cu nanocomposite are presented in the ESI and are shown in Fig. (S1–3).†
2.2.2 Characterization of the prepared samples.
The crystallinity and formed phases of the photocatalyst samples were analyzed by X-ray diffractometry (XRD) using an Ultima IV X-ray diffractometer (Rigaku, Japan) operated at 40 kV and 30 mA using CuKα radiation (λ = 1.54 Å). The atomic composition and chemical states were investigated using X-ray photoelectron spectroscopy (XPS) on a PHI Quantera SXM scanning X-ray microprobe (ULVAC-Phi, Inc., Japan). In addition, the external morphologies, purities, and mass compositions of the prepared samples were evaluated via scanning electron microscopy (SEM) and energy-dispersive X-ray spectroscopy (EDX) (SU8000 Type II-HITACHI high technologies, Japan). Transmission electron microscopy (TEM) (JEM-2100F (JEOL Ltd, Japan) was used to determine the average particle size and crystallographic plane calculations, while scanning tunneling electron microscopy (STEM) analysis was carried out to reveal the distribution of Cu on the composite matrix. A Tristar II (Micromeritics, Japan) instrument was used to estimate the surface areas and porosities of the photocatalyst samples, facilitated by Brunauer–Emmett–Teller (BET) and Barrett–Joyner–Halenda (BJH) analyses. Fourier-transform infrared (FTIR) analysis using the potassium bromide (KBr) method was conducted to reveal the type of chemical bonding using an FTIR 3600 Jasco infrared spectrometer (Japan). Raman spectroscopy (NRS-3100, Jasco, Japan) was performed to investigate the chemical structures, molecular interactions, and polymorphism of the photocatalyst samples. The stability of the prepared samples was analyzed by carrying out zeta potential calculations using an ELS-Z1NT instrument (Photal Otsuka Electronics, Japan). Finally, diffuse-reflectance analysis and calculations using the Kubelka–Munk equation were employed to determine the absorption and bandgap energy using a V-670 spectrophotometer (Jasco, Japan).
2.2.3 Photocatalytic degradation and antimicrobial activity evaluation.
Detailed experimental steps for the evaluation of both photocatalytic and antimicrobial performance of the prepared samples are shown in the ESI.†
3. Results and discussion
3.1 Characterization of the prepared samples
XRD analysis of the prepared samples shows strong and intense peaks, indicating their crystallinity as shown in Fig. S4.† For Cu NPs, several diffraction peaks were recorded at 2θ = 44.3°, 50.4°, and 74.3°, which correspond to the (111), (200), and (220) lattice planes, and are a good match to the standard peaks of Cu (JCPDS no. 71-4610).22 In addition, this recorded pattern confirms the formation of a face-centered cubic (FCC) crystal structure, which is in good agreement with the selected area electron diffraction (SAED) analysis Fig. 1(c). While the diffraction peaks of the bare composite matrix (CNFST) were recorded at 2θ = 25.3°, 36.1°, 37.4°, 38.5°, 48.5°, 54.6°, 55.4°, 64.8°, 69.8°, 71.58°, and 75.6°, which correspond to the (101), (103), (004), (112), (200), (105), (211), (204), (116), (220), and (215) crystallographic planes, respectively. The recorded diffraction peaks and their corresponding planes can be mainly attributed to anatase TiO2 (JCPDS 21-1272),23,24 the principal external layer of the composite matrix. This conclusion was confirmed from the mass percentage analysis of the EDX Fig. S5(b–d)† and atomic percentage analysis using XPS (Fig. 3). A combination of peaks of the bare composite matrix and Cu NPs can be observed in the pattern of the Cu-loaded composite (CNFST/Cu), indicating their conjugation, as supported by the SEM observation shown in Fig. S5(c),† STEM mapping in Fig. S5(f),† and TEM analysis in Fig. 1(g). It is worth mentioning that a small amount of the loaded Cu NPs over the CNFST surface was oxidized into Cu2O (JCPDS No. 05-0667),25 which is indicated by two small peaks at 36.8° (111) and 42.3° (200) in the XRD pattern. Finally, the calculated crystal sizes from the Scherrer equation were 38.29, 16.04, and 22.78 nm for the Cu NPs, CNFST, and CNFST/Cu samples, respectively.
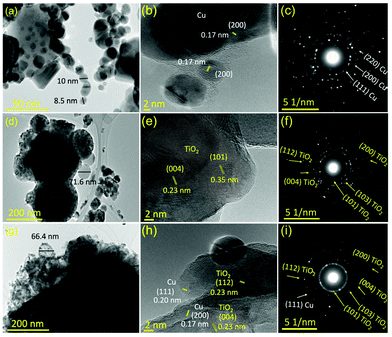 |
| Fig. 1 TEM analyses, HR-TEM observations, and SAED patterns of the (a–c) Cu NPs, (d–f) CNFST matrix, and (g–i) CNFST/Cu 10% nanocomposite. | |
Fig. S5† shows the SEM-observed surface morphology and EDX mass percentages of the bare CNFST and CNFST/Cu nanocomposites. It is obvious that the bare CNFST composite matrix exhibits homogeneous spherical-shaped structures as presented in Fig. S5(a).† While its EDX analysis confirms that Ti has the highest mass ratio, followed by O forming the external TiO2 layer of the CNFST nanocomposite. In addition, SEM observations reveal that Cu NPs are uniformly distributed as clusters on the external layer of the CNFST matrix as shown in Fig. S5(c).† These results are in a good agreement with STEM mapping shown in Fig. S5(f).†
While, Fig. 1(a, d and g) show the TEM images of the prepared Cu NPs, bare CNFST matrix, and CNFST/Cu 10% nanocomposite, respectively. The Cu NPs and matrix particles are almost spherical in shape, with average diameters of around 9 and 70 nm, respectively. The CNFST/Cu nanocomposite exhibits almost the same average diameter as that of the bare CNFST sample.
The high-resolution TEM (HR-TEM) images confirm the conjugation of Cu NPs with the TiO2 layer as shown in Fig. 1(h), because of the presence of characteristic crystallographic planes of both Cu and TiO2 that have d-spacings of 0.17 nm (200) and 0.20 nm (111) for Cu NPs, and 0.238 nm (004) and 0.233 nm (112) for TiO2 NPs. It is worth mentioning that the observed lattice fringes shown in Fig. 1(e) and (h) can be ascribed to anatase TiO2, confirming that TiO2 is the main layer of the composite matrix, matching both XRD and EDX results. Finally, analysis of SAED patterns confirms the formation of Cu NPs with an FCC crystal structure as shown in Fig. 1(c), the dominance of anatase TiO2 in the nanocomposite matrix, as shown in Fig. 1(f), and the conjugation of the Cu NPs with TiO2 to form the CNFST/Cu nanocomposite, as shown in Fig. 1(i).
N2 adsorption–desorption isotherms and BJH pore size distribution analysis are shown in Fig. S6.† According to the IUPAC classification, the bare CNFST matrix exhibits a type III adsorption isotherm, indicating the weak interactions between the adsorbate and adsorbent (N2 gas and CNFST), as shown in Fig. S6(a).† However, upon loading Cu NPs over the CNFST matrix, the adsorption type changes from type III to V, which points out to the formation of mesopores (2–50 nm). In addition, the resultant nanocomposite shows an improved surface area, as shown in Table 1.
Table 1 Calculated BET surface areas, pore areas, and pore volumes of the prepared samples
No. |
Sample |
BET surface area (m2 g−1) |
Pore volume (cm3 g−1) |
Pore area (m2 g−1) |
1 |
CNFST |
4.78 ± 0.05 |
0.0008 |
1.63 |
2 |
CNFST/Cu 10% |
11.34 ± 0.08 |
0.0007 |
1.54 |
While Fig. S6(b and c)† shows the BJH pore size distributions of the samples. The prepared samples exhibit both multimodal and wide porosity distribution. On the one hand, the bare CNFST matrix showed 2 weak peaks in the mesoporous region (2–50 nm) and a large increasing portion in the macroporous region (>50 nm). On the other hand, CNFST/Cu nanocomposite possessed a larger ratio of mesopores and less decreasing volume of macropores. As a result of the formation of more mesopores after Cu loading, the observed surface area was improved.
Table 2 shows the zeta potential values, net charge, and corresponding stability of the prepared samples. it can be seen that Cu NPs exhibit a net positive charge and high tendency to agglomerate in aqueous solutions, while the negatively-charged CNFST matrix shows moderate stability that can be attributed to its higher zeta potential value.26 Finally, the CNFST/Cu nanocomposite exhibited a net positive charge, and its zeta potential and stability index were found to be in the middle between both samples.7
Table 2 Zeta potential and the corresponding colloidal stability of the prepared samples
No. |
Sample |
Zeta potential (mV) |
Stability |
1 |
Cu |
+4.22 |
Strong agglomeration |
2 |
CNFST |
−38.62 |
Moderate stability |
3 |
CNFST/Cu 10% |
+22.08 |
Incipient instability |
Chemical bonding and functional groups of the prepared samples were revealed by the FTIR spectra, shown in Fig. S7.† The absence of Cu–O stretching vibrations suggests that the volume fraction of Cu oxide is very small, and Cu NPs were loaded onto the TiO2 surface via weak electrostatic van der Waals forces.
While XPS analysis was carried out to investigate the chemical states and to estimate the atomic ratios of the main elements constituting the CNFST/Cu nanocomposite, as shown in Fig. 2(a–d). Fig. 2(a) shows the survey XPS spectrum of the nanocomposite, from which multiple peaks corresponding to all constituting elements were recorded. Fig. 2(b–d) shows the deconvoluted peaks of the main elements, Cu, Ti, and O. For Cu 2p three peaks were detected, one at 951.6 eV and two peaks at 932.3 and 933.9 eV, which are characteristic of metallic copper Cu(0) or Cu(I) and Cu(II), respectively.27 This confirms the presence of oxidized Cu on the surface of the nanocomposite, matching the XRD analysis results. For Ti 2p, two peaks at binding energies of 464.5 and 458.2 eV can be observed that confirm that the Ti4+ state is dominant in the TiO2 layer.28 Finally, O 1s is represented by two peaks, one at 531.3 eV, which is due to the presence of hydroxyl groups on the surface of the composite or adsorbed water molecules, and the second at 529.6 eV corresponding to metal oxides of Ti and Cu. In addition, the observed shoulder (>531.3 eV) toward higher binding energies corresponds to a large number of oxygen vacancies that are present on the surface of the material, which is favorable for increased photocatalytic efficiency due to their role as electron reservoirs.29,30
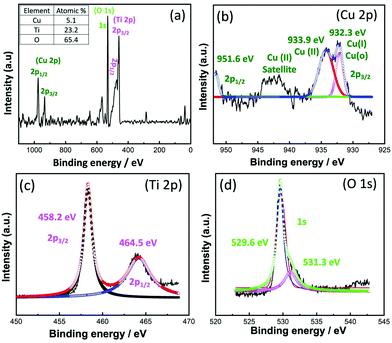 |
| Fig. 2 XPS (a) survey analysis and atomic ratios and (b) deconvoluted Cu 2p, (c) Ti 2p, and (d) O 1s spectra. | |
Finally, diffuse-reflectance spectra were recorded, and bandgap energy values were calculated using the Kubelka–Munk equation as shown in Fig. 3. Both nanocomposites exhibit absorption in the UV region at around 350 nm (Fig. 3(a)), while bandgap calculations show that upon Cu loading the bandgap energy slightly increased from 3.1 to 3.15 eV, as presented in Fig. 3(b). This result may be attributed to the fact that Cu NPs absorb visible light, and that the absorption wavelength range overlaps with that of TiO2, affecting the extrapolation used to calculate the bandgap energy value.
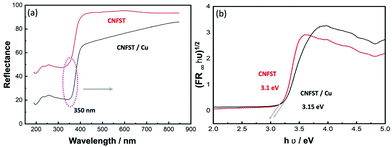 |
| Fig. 3 (a) Diffuse-reflectance spectra of the CNFST and CNFST/Cu 10% nanocomposites and (b) bandgap energy calculations acquired using the Kubelka–Munk equation. | |
3.2 Photocatalytic activity of the prepared samples
3.2.1 Potassium permanganate degradation (inorganic dye).
Fig. 4(a–c) shows a comparison of the photocatalytic degradation of potassium permanganate inorganic dye by the prepared samples along with dye photolysis and dye removal due to adsorption in the dark. From the graph, it can be clearly observed that in the absence of the prepared samples, the employed UV light degrades only around 11% of the dye after 4 h, as shown in Fig. 4(a), while adsorption in the dark leads to almost 17% removal of the dye at the same time, as shown in Fig. 4(b). However, photocatalytic removal using the prepared samples is more efficient, as shown in Fig. 4(c). The prepared CNFST/Cu sample shows the highest dye removal efficiency, with around 44% dye removal after only 80 min. This improved photocatalytic activity can be ascribed to the synergistic effect between the Cu NPs and the prepared CNFST nanocomposite.
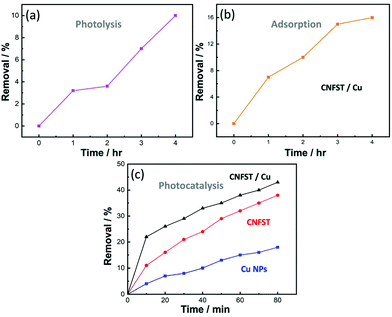 |
| Fig. 4 Potassium permanganate degradation by (a) photolysis (no photocatalysts), (b) adsorption in the dark (no light), and (c) photocatalytic degradation by the prepared samples under UV irradiation (10 mg photocatalyst, 50 ml vol, 20 ppm dye conc., and pH = 7). | |
3.2.2 Chromium(VI) reduction.
The photocatalytic reduction of chromium ions by the prepared samples was investigated at different pH values in the range of 2–5 using 1 mM EDTA as a hole scavenger, with the results shown in Fig. 5(a–d). Similarly, the CNFST/Cu sample exhibits a much more enhanced Cr(VI) reduction efficiency compared to the bare CNFST nanocomposite and the Cu NPs. Fig. 5(a) reveals that almost 57% of Cr(VI) ions were reduced to Cr(III) ions after 120 min at pH 5 compared to only 19% and 25% by the Cu and bare CNFST nanocomposite, respectively. While at pH 2, the Cr(VI) reduction performance is much higher than that at pH = 5 as revealed by Fig. 5(b). Even at pH = 2, the CNFST/Cu sample exhibits a superior Cr(VI) reduction ability compared to the bare CNFST sample, with almost 100% of Cr(VI) being reduced after 60 min, as revealed in Fig. 5(c). Finally, Fig. 5(d) shows the effect of using EDTA on the reduction performance of Cr(VI), it is clear that on using 1 mM EDTA, the CNFST/Cu sample shows a more enhanced reduction ability due to efficient charge separation.
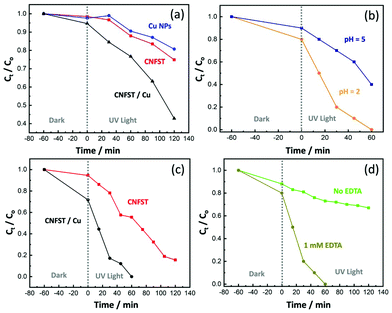 |
| Fig. 5 (a) Cr(VI) reduction by the prepared samples (10 mg photocatalyst, 20 ppm Cr(VI) solution, pH 5, and 1 mM EDTA), (b) the effect of pH on Cr(VI) reduction by the CNFST/Cu sample, (c) Cr(VI) reduction at pH 2 (other parameters are fixed), and (d) the effect of EDTA on Cr(VI) reduction by the CNFST/Cu sample. | |
3.2.3 pNA degradation (organic dye).
It can be clearly observed from Fig. 6(a) that the degradation of pNA due to photolysis after 4 h was only 9%, while the removal due to adsorption in the dark was around 18% after the same amount of time, as shown in Fig. 6(b). While Fig. 6(c) shows the progressive decline in the absorption peaks (at λmax = 380 and 230 nm) due to photodegradation by the CNFST/Cu photocatalyst under UV irradiation.31Fig. 6(d) shows that the CNFST/Cu photocatalyst possesses the highest photocatalytic activity (65% removal) toward pNA compared to bare Cu dots (22% removal) and the bare CNFST nanocomposite (26% removal), after 90 min under UV irradiation. This improved photocatalytic efficiency can be attributed to the effect of the constructed metal–semiconductor heterojunction of the nanocomposite, which facilitates charge separation and the absorption of incident light. In addition, it features a relatively higher surface area (11.34 m2 g−1) and a network of mesopores that facilitate the photocatalytic reaction, as confirmed by the BET surface area and porosity analyses. In addition, the presence of surface oxygen vacancies enhanced light absorption leading to the production of more photogenerated electrons for improved photodegradation performance.32,33
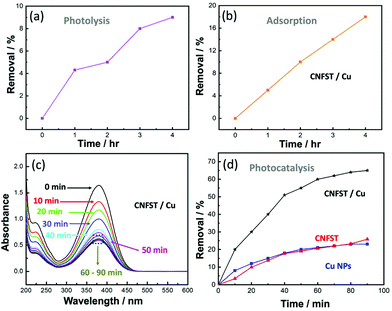 |
| Fig. 6 Removal of pNA by (a) photolysis, (b) adsorption, (c) absorbance reduction with time due to the CNFST/Cu nanocomposite, and (d) a comparison of the photocatalytic degradation of the prepared samples (10 mg photocatalyst, 50 ml vol, 10 ppm dye conc., and pH = 7). | |
3.2.3.1 Effect of the photocatalyst dose and initial pNA concentration.
The effects of the photocatalyst dose and the initial pNA concentration on the photocatalytic performance were analyzed, as shown in Fig. S8(a and b).† Upon increasing the photocatalyst dose from 5 to 20 mg in an aqueous solution of pNA, the percentage of pNA removal increases from 43% to 95% after 90 min of irradiation under UV light, as shown in Fig. S8(a).† As the amount of the CNFST/Cu photocatalyst increases, there is an increase in the number of active sites available for pNA removal. In other words, by increasing the number of active sites on the surface of the employed photocatalyst, the number of absorbed photons increases, thus leading to more redox reactions and enhanced efficiency. Consequently, more charge carriers and more free radicals are likely to be generated. Secondly, it was observed that as the initial concentration of pNA increases from 5 to 15 ppm, the percentage removal of pNA decreases from 84% to 46.67%, as shown in Fig. S8(b).† The increase in the concentration of pNA leads to the formation of aggregates on the surface of the photocatalyst. Consequently, a few photons can reach and stimulate redox reactions over the surface of the photocatalyst, thus leading to a reduction in the degradation efficiency.34 In addition, after a specific time the vacant sites of the CNFST/Cu photocatalyst become saturated with the adsorbed pNA molecules. Moreover, repulsive forces occur between pNA and the CNFST/Cu photocatalyst, which lead to the negligible degradation of pNA. This implies that after an equilibrium state is reached, further irradiation does not lead to more degradation.35,36
3.2.3.2 Reaction kinetics and the apparent rate constant.
The reaction kinetics of the degradation of pNA under UV light using the prepared CNFST/Cu photocatalyst are a good fit to a pseudo first-order model, as shown in the results presented in Fig. S9(a).† In addition, it can be observed that the value of the reaction rate constant, k, decreases in line with an increase in the initial concentration of pNA, as shown in Fig. S9(b),† which is in a good agreement with the degradation performance results. As the concentration of pNA increases, fewer H2O molecules are adsorbed onto the surface of the CNFST/Cu photocatalyst and the number of created hydroxyl radicals is reduced.37
3.2.3.3 Effect of the initial pH and the point of zero charge (PZC).
The impact that solution pH has on the elimination rate of pNA in the presence of the CNFST/Cu photocatalyst is shown in Fig. S10(a).† In acidic solution (pH 5), the pNA concentration is reduced by 18% compared to its original concentration after 90 min under UV light, while the degradation of pNA reaches around 66% in neutral solution (pH 7). However, after the same contact time, around 71% of pNA is degraded in an alkaline solution (pH 9). This result can be ascribed to the electrostatic interactions occurring between pNA molecules and CNFST/Cu photocatalyst in an alkaline medium, which lead to an enhancement in the number of redox reactions that take place.38 The pH of the PZC was recorded as 7.5 as shown in Fig. S10(b),† which implies that the surface charge of the CNFST/Cu composite is positive in the acidic medium, whereas, it possesses a negative charge in the alkaline media. Furthermore, at neutral pH, the surface charge is zero and the electrostatic forces that occur between the CNFST/Cu composite surface and pNA molecules can be disregarded.39 The proposed photocatalytic reaction mechanism of the prepared CNFST/Cu photocatalyst is shown in Fig. S11.†
3.3 Molecular modeling
To explain the interaction between the modified TiO2 (external layer) and the tested pNA, DFT calculations were employed to determine the electronic and adsorption properties of TiO2 before and after modification with Cu NPs.40
3.3.1 Computational model and structure.
DFT calculations were performed using the Gaussian 16 software at the M062X/6-31(d,p) level of theory.41 To account for the possibility of the occurrence of van der Waals interactions between the adsorbent (TiO2) and the adsorbate (pNA), Grimme's dispersion correction (gd3) was added to the M062X functional. As shown in Fig. S12(a),† the TiO2 layer is represented by a finite nanoflake. Thus, to study the effect that the addition of Cu NPs and Cu oxides has on the adsorption properties of the nanoflake, Cu, CuO, and Cu2O were attached, as shown in Fig. S12(b–d).† These constructed structures were then allowed to interact with pNA initially placed 3.5 Å above them, as shown in Fig. S12(g).† It can be observed from Fig. S12(f–h)† that the addition of Cu and Cu oxides improves the adsorption of pNA (with respect to the unmodified TiO2 in Fig. S12(e)†), this is because of the formation of several chemical bonds between the O and C atoms of pNA and the Cu atoms of the chemically-modified TiO2 nanoflake.
To confirm these findings, the adsorption energy (Ea) was calculated before and after the addition of Cu and its oxides, as summarized in Table 3, where Ea was calculated using the formula: Ea = (Ead + EpNA − Er)/n, where Ead is the ground state energy of the adsorbent (TiO2, TiO2–CuO, etc.), EpNA is the ground state energy of pNA, Er is the ground state energy of the product after adsorption, and n is the total number of atoms. From the values of Ea shown in Table 3, the attachment of Cu oxides increased the adsorption energy by double the original value before the attachment, from ∼0.05 eV for TiO2/pNA to 0.1 eV for TiO2–CuO/pNA or TiO2–CuO–Cu2O/pNA.
Table 3 The calculated HOMO/LUMO energy gap (Eg) and adsorption energy (Ea) for the selected structures
Structure |
E
g (eV) |
E
a (eV) |
TiO2 |
3.73 |
— |
pNA |
6.78 |
— |
TiO2–CuO |
3.93 |
— |
TiO2–CuO–Cu |
3.27 |
— |
TiO2–CuO–Cu2O |
3.08 |
— |
TiO2/pNA |
3.12 |
0.0496 |
TiO2–CuO/pNA |
3.68 |
0.0961 |
TiO2–CuO–Cu2O/pNA |
2.76 |
0.1070 |
TiO2–CuO–Cu/pNA |
3.19 |
0.0798 |
It is worth mentioning that the increase in the adsorption energy in the case of the TiO2–CuO–Cu/pNA structure is unique, which can be ascribed to the closeness of the Cu atoms in this system, leading to strong interactions between them, as presented in Fig. S12(g).†
3.3.2 Electronic density of states.
The electronic density of states of Cu-modified TiO2 before and after the adsorption of pNA is shown in Fig. 7. In this representation, the molecular orbitals are represented by Gaussian functions with a broadening of α = 0.032 eV. The highest occupied molecular orbital (HOMO) distributions are also presented to show the effects that chemical modification and the adsorption of pNA have on this electronic orbital. The energy gap of TiO2, as shown in Table 3 and Fig. 7(a), is 3.73 eV, which is slightly higher than the experimental value of 3.1 eV. This increase in the energy gap is the result of the quantum size effect, where the smaller the TiO2 nanoflake, the higher the energy gap. Attaching one CuO molecule to the structure increased the energy gap to 3.93 eV, with these increases expected to be due to the passivation of the interactive electrons of the Ti atoms at the edge. This effect, seen in Fig. 7(c), results from a decrease in the intensity of the distribution cubes on the Ti atoms around the CuO. However, further addition of Cu atoms or Cu2O molecules decreases the energy gaps to 3.27 and 3.08 eV, respectively.
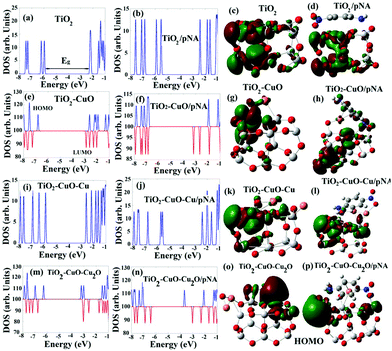 |
| Fig. 7 Electronic density of states and the corresponding HOMO of the studied TiO2 structures before and after the adsorption of pNA. The blue and red peaks in the DOS spectra represent spin up and spin down molecular orbitals, respectively. Dark red and blue cubes in the HOMO distribution represent the positive and negative signs of the molecular orbital wave function. | |
Increasing the number of attached Cu and Cu oxides increases the interactions between them and TiO2, leading to the creation of low-energy electronic states within the energy gap, and thus reducing it.42,43 For example, the HOMO of TiO2–CuO–CuO2 is located almost around the attached CuO. In other words, it originates from the weaker bonds between CuO and TiO2. Therefore, Cu and Cu oxides can be used to enhance the photocatalytic properties of TiO2 by decreasing its energy gap toward the visible light region. The energy gap further decreases after the adsorption of pNA due to the same reason, where the highest decreases to 2.76 eV for TiO2–CuO–CuO2/pNA corresponds to the highest interaction and thus the highest absorption. Fig. 7 shows the HOMO distributions before and after the adsorption of pNA, which provides additional confirmation for the improved adsorption capability of the system upon the addition of Cu and Cu oxides. In the first case, as shown in Fig. 7(d), the HOMO is distributed only on the TiO2, which implies moderate physical adsorption, while in the other cases, the HOMO is distributed on both TiO2 and pNA due to the formation of chemical bonds between TiO2 and pNA.
3.3.3 Molecular electrostatic potential (MESP).
Fig. S13† shows MESP contour maps, which indicate the computation of the surrounding charges, nucleus, and electron concentration at a particular position, using the colors red, orange, yellow, green, and blue, where red represents the lowest MESP level and blue represents the highest MESP level. The red color indicates positions with the highest electrostatic repulsion and more reactivity, whereas the blue color show positions with the lowest electrostatic repulsion. For the pNA and TiO2 model, the red color is localized around the oxygen atoms of NO2 and around the oxygen atoms of the TiO2 corners. When pNA and TiO2 interact, the color distribution of TiO2 varies, resulting in the red color spreading around the TiO2 oxygen atoms, reflecting the increase in the TiO2 reactivity. Furthermore, in the case of TiO2/CuO, the presence of CuO increases the reactivity of TiO2, resulting in the concentration of the red color around the TiO2 structure. The interaction of Cu with TiO2–CuO leads to a significant change in the color distribution of TiO2–CuO, with an increase in the red color around the oxygen atoms of TiO2 reflecting the increase in reactivity. Meanwhile, in the case of TiO2–CuO–CuO2, the oxygen atoms of TiO2 as well as those of CuO are red in color, meaning that it is more active. The color distribution of the TiO2–CuO–Cu/pNA structure indicates an increase in the reactivity around the TiO2, as observed in the expansion of the red color around it. Finally, in terms of the interactions of pNA with TiO2–CuO and TiO2–CuO–CuO2, the red color density increases across the surface of the structure, indicating that the presence of pNA enhances the reactivity of the structure.
3.4 Antimicrobial activity of the synthesized nanocomposites
3.4.1 Well diffusion methods.
As presented in Fig. 8 and Table 4, all samples hinder the microbial growth of the tested pathogenic bacteria and unicellular fungi. However, after the loading of Cu NPs into the nanocomposite, the antimicrobial activity of the bare nanocomposite was improved. The prepared CNFST/Cu sample shows significant growth inhibition against all tested microbes (except for P. vulgaris) with respect to the positive control (amoxicillin and nystatin), Cu NPs, and the bare CNFST nanocomposite. It is worth mentioning that the highest antimicrobial activity of the prepared CNFST/Cu sample was recorded against the S. aureus bacterial strain, which led to a ZOI of around 35.6 ± 0.58 mm compared to only 20.5 ± 0.76 mm for the bare CNFST sample, 13.2 ± 0.58 mm for Cu NPs, and around 14.5 ± 0.53 mm for the positive control. The MIC results (Table 4) show the promising application of the prepared nanocomposites as antimicrobial agents. The lowest recorded MIC concentration exhibited by the bare CNFST nanocomposite and the positive control is 0.48 μg mL−1 against E. coli. While the CNFST/Cu nanocomposite showed a MIC concentration of around 0.03 μg mL−1 against S. aureus compared with 62.5 μg mL−1 for Cu NPs. In addition, it possessed dual functionality, acting as bacteriostatic at low concentrations, and a bactericidal at high concentrations, as reported in ref. 44. This enhanced activity can be attributed to the synergistic effect of the Cu NPs and the prepared CNFST nanocomposite.
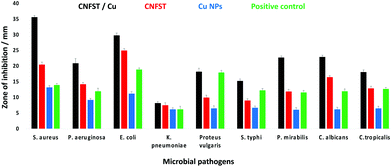 |
| Fig. 8 ZOI antimicrobial test of the prepared samples against different pathogenic bacteria and unicellular fungi. | |
Table 4 Antimicrobial ZOI and MIC values of the prepared samples against different bacteria and Candida strains
Test organism |
CNFST/Cu |
CNFST |
Amoxicillin/Nystatin |
Cu NPs |
ZOI (mm) |
MIC μg ml−1 |
ZOI (mm) |
MIC μg ml−1 |
ZOI (mm) |
MIC μg ml−1 |
ZOI (mm) |
MIC μg ml−1 |
ZOI: zone of inhibition, MIC: minimum inhibitory concentration. Values are means ± SD (n = 3). Data within the groups were analyzed using one-way analysis of variance (ANOVA) followed by a, b, c, d, e, f, g, h Duncan's multiple range test (DMRT). |
Staphylococcus aureus
|
35.6 ± 0.58a |
0.03 |
20.5 ± 0.76b |
7.81 |
14.0 ± 0.46c |
250 |
13.2 ± 0.58a |
62.5 |
Pseudomonas aeruginosa
|
20.9 ± 1.53f |
7.81 |
14.2 ± 0.58d |
62.5 |
12.0 ± 1.00d |
250 |
9.2 ± 0.50c |
250 |
Escherichia coli
|
29.8 ± 0.76bc |
0.12 |
25.0 ± 0.53a |
0.48 |
18.9 ± 0.50a |
0.48 |
11.2 ± 0.72b |
125 |
Klebsiella pneumoniae
|
8.2 ± 0.50h |
250 |
7.5 ± 0.87fg |
250 |
6.2 ± 1.00f |
500 |
6.20 ± 0.53f |
500 |
Proteus vulgaris
|
18.2 ± 1.15g |
31.25 |
10.0 ± 0.69d |
31.25 |
18.0 ± 0.68b |
31.25 |
6.5 ± 0.87de |
500 |
Salmonella typhi
|
15.3 ± 0.64e |
125 |
9.0 ± 0.58f |
125 |
12.3 ± 0.61d |
250 |
6.7 ± 0.58d |
500 |
Proteus mirabilis
|
22.7 ± 0.52ef |
7.81 |
11.9 ± 0.72e |
31.25 |
11.6 ± 0.66c |
62.5 |
6.1 ± 0.68g |
500 |
Candida albicans
|
22.9 ± 0.58de |
0.48 |
16.5 ± 0.50c |
7.81 |
12.0 ± 0.70d |
250 |
6.2 ± 0.69f |
500 |
Candida tropicalis
|
18.1 ± 0.66e |
1.95 |
12.9 ± 0.68d |
31.25 |
12.7 ± 0.52d |
250 |
6.5 ± 0.70d |
500 |
3.5 Growth curve assay
The repression impact of the prepared nanocomposites on S. aureus is presented in Fig. 9(a). The extension rate of S. aureus in the untreated control group is fast, with the most potent optical density (at λ = 600 nm) (OD600) value of around 2.78 nm. By contrast, the OD600 values of the nanocomposites are lower, showing a repression impact. The performance of both nanocomposites can be attributed to two main factors. Firstly, the presence of Co–Ni ferrite NPs, which exhibit good antibacterial potential against different bacterial strains (S. aureus, P. aeruginosa, and E. coli) and unicellular fungi.45 Secondly, the positively-charged liberated metal ions (Co2+,Cu2+, Fe2+, Ni2+, Si2+, and Ti2+) from the prepared nanocomposite facilitate the electrostatic interactions with the negatively-charged membrane of S. aureus.46 The generated ROS on the surface of the prepared samples results in protein oxidation, DNA injury, and lipid peroxidation, which destroy bacterial cells.47
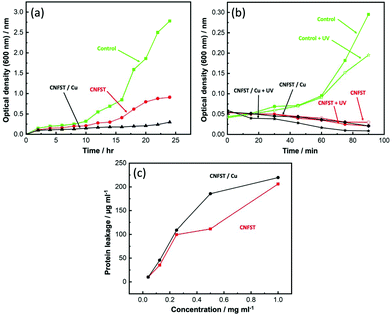 |
| Fig. 9 (a) The effect of the prepared nanocomposites on the growth curve of S. aureus, (b) UV activation effect of the prepared nanocomposites against S. aureus, and (c) impact of the prepared nanocomposites on protein leakage from S. aureus cell membranes. | |
The CNFST/Cu sample shows the best performance due to the presence of Cu NPs exhibiting unique antibacterial activity, as described in previous studies.48,49 It is worth mentioning that the higher hypersensitivity of the Gram-positive bacteria toward the nanocomposites may be explained as a consequence of their less turgid cell membrane.50
3.6 Effect of UV activation: disinfection potential of the prepared samples
The effect of UV activation of the prepared samples on S. aureus is shown in Fig. 9(b). With respect to the untreated control group, the growth of S. aureus was significantly reduced after the treatment with both nanocomposites, with the CNFST/Cu sample showing excellent disinfection potential upon UV exposure. In water and air, and upon photon absorption, active hydroxyl (˙OH) and ROS (O2−, and H2O2) species are generated in the presence of O2 and H2O.51 The ROS produced, particularly H2O2, can penetrate the cell membranes and produce active oxidative hydroxyls via a reaction within the bacterial cells that ultimately leads to bacterial disinfection.52,53
3.7 Determination of protein leakage from the bacterial cell membranes
The amount of protein leakage in the suspension of S. aureus cells treated by the prepared samples was calculated using the Bradford method.54 From Fig. 9(c), it can be observed that the quantity of cellular protein leakage from S. aureus is proportional to the concentrations of the employed nanocomposites. Protein leakage of 205.95 and 219.58 μg mL−1 was detected after treatment with 1 mg mL−1 of CNFST and CNFST/Cu nanocomposites, respectively, confirming the strong antibacterial characteristics of the synthesized nanocomposites, and indicating the creation of holes in the cell membrane of S. aureus that led to the leakage of protein from the S. aureus cytoplasm. Related studies55 describe comparable outcomes with ferrites, which revealed concentration-dependent destabilization in the cell membrane of bacterial cells and the leakage of their intracellular substance into the extracellular form (bacterial cell suspension). It can thus be assumed that the disruption of membrane permeability is a vital process in the repression of bacterial mass.
3.8 Reaction mechanism estimation by SEM analysis
SEM analysis was carried out to determine the possible antimicrobial mechanism against S. aureus, with the results presented in Fig. 10. SEM analysis of the control (untreated) bacterial cells shows prolonged cells with regular surfaces, as displayed in Fig. 10(a). However, after treatment with the CNFST nanocomposite, unusual morphological irregularities of the S. aureus cells were identified, including semilysis of their outer surfaces and some deformations, as can be observed in Fig. 10(b). Upon treatment with the CNFST/Cu nanocomposite, complete lysis of the bacterial cells (cell malformation) was achieved, decreasing the total number of viable cells, as shown in Fig. 10(c), creating holes on the surface of the bacterial cells, as can be observed in Fig. 10(d), which is in good agreement with the membrane leakage assay.
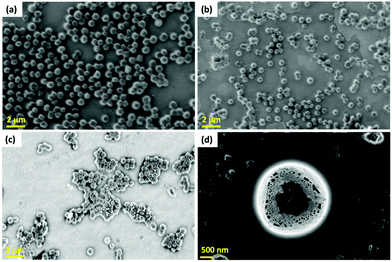 |
| Fig. 10 Reaction mechanism estimation using SEM analysis, (a) control (untreated) S. aureus cells, (b) treated cells with the CNFST nanocomposite, (c) treated cells with the CNFST/Cu nanocomposite, and (d) a magnified bacterial cell treated with the CNFST/Cu nanocomposite confirming the creation of holes on the surface of treated S. aureus bacterial cells. | |
The possible antimicrobial mechanism is presented in Fig. S14.† There are many possible factors that contribute to this mechanism, such as ROS (superoxide anions, O2−),56 the liberated ions from the metal and metal oxides inside the pathogenic microbes, and the alkaline conditions. In addition, ferrites and Cu NPs exhibit good antimicrobial properties, meaning that these materials can alter the microbial morphology, diminish the microbial membrane permeability, and generate oxidative stress due to H2O2 production.56,57
The CNFST/Cu nanocomposite initiates its activity by adhesion at the exterior surface of the microbial cells, leading to membrane damage, the formation of pits, and the switching off of the ion transport activity.58 Then, the liberated metal ions destroy all intracellular macromolecules, such as DNA, plasmids, and essential bacterial organs. In addition, cellular toxicity takes place because of the oxidative pressure facilitated by the production of ROS. Finally, in an acidic medium, cellular toxicity and genotoxicity occur due to the actions of the core ferrite and the surface Cu NPs.58
4. Conclusion
An optimized layer-by-layer approach was used to prepare a Cu-loaded Co0.5Ni0.5Fe2O4/SiO2/TiO2 nanocomposite, which was then utilized for the photodegradation of three types of pollutants (potassium permanganate, chromium ions, and pNA) under UV light. DFT analysis was carried out to investigate the adsorption and electronic properties of the prepared CNFST/Cu sample. Moreover, the antimicrobial performance of the prepared nanocomposites against many types of pathogenic bacteria and unicellular fungi was studied using various assays, such as well diffusion, UV-light activation assessment, and protein leakage. The results in this study show that FCC Cu NPs are formed and uniformly distributed on the surface of the prepared nanocomposite matrix as revealed from XRD, SEM, and STEM mapping results. Upon Cu loading, the crystal size, diameter of the particles, and the bandgap of the CNFST matrix slightly increase. However, the BET surface area is largely improved due to more mesopores being formed compared to macropores, as shown in the BJH pore size distribution curves. The FTIR analysis shows no Cu–O stretching, suggesting that the Cu NP loading occurs via van der Waals forces. The prepared nanocomposite exhibits strong absorption in the UV region at around 350 nm. Finally, XPS analysis shows the atomic ratios of the main elements that constitute the composite and confirms the existence of oxygen vacancies over the Cu-loaded composite. The photocatalytic properties of the prepared nanocomposite were tested and compared to those of bare Cu and CNFST samples, with the prepared Cu-loaded nanocomposite showing outstanding photocatalytic properties toward all tested pollutants. This efficiency can be attributed to the improved surface area, porosity, and the constructed heterojunction separating photogenerated charge carriers and elongating the reaction time. In addition, the effect of the pH on the photocatalytic properties of the prepared nanocomposite varied from one type of pollutant to another. Similarly, the Cu-loaded nanocomposite shows significant antimicrobial properties toward almost all pathogenic microbes. This performance can be ascribed to the synergetic effect between the composite matrix and the loaded Cu NPs.
Author contributions
M. Abd Elkodous: Conceptualization, data curation formal analysis, and writing – original draft. Ahmed M. El-Khawaga: Data curation and formal analysis. Gharieb S. El-Sayyad: Data curation, formal analysis, and writing – original draft. M. I. A. Abdel Maksoud: Formal analysis and writing – original draft. Nurhaswani Alias: Data curation. Hazem Abdelsalam: Formal analysis and writing – original draft. Medhat A. Ibrahim: Supervision. Mohamed A. Elsayed: Supervision. Go Kawamura: Data curation and supervision. Zainovia Lockman: Supervision. Wai Kian Tan: Writing – review – editing and supervision. Atsunori Matsuda: Writing – review – editing and supervision.
Conflicts of interest
There are no conflicts to declare.
Acknowledgements
The authors A. Matsuda and G. Kawamura would like to acknowledge the Japan Society for Promotion of Science (JSPS) KAKENHI Grant No. 18H03841 and No. 21K18823.
References
- T. R. Zolnikov, F. Furio, V. Cruvinel and J. Richards, Waste Manage., 2021, 126, 291–308 CrossRef PubMed.
- J. R. Laszakovits and A. A. MacKay, Water Res., 2019, 155, 86–95 CrossRef CAS PubMed.
- V. K. Agrawal, A. Bansal, R. Kumar, B. L. Kumawat and P. Mahajan, Indian J. Crit. Care Med., 2014, 18, 819 CrossRef PubMed.
- T. Pavesi and J. C. Moreira, J. Appl. Toxicol., 2020, 40, 1183–1197 CrossRef CAS PubMed.
- M. Miskam, N. K. Abu Bakar and S. Mohamad, Talanta, 2014, 120, 450–455 CrossRef CAS PubMed.
- G. S. El-Sayyad, M. Abd Elkodous, A. M. El-Khawaga, M. A. Elsayed, A. I. El-Batal and M. Gobara, RSC Adv., 2020, 10, 5241–5259 RSC.
- M. Abd Elkodous, G. S. El-Sayyad, M. I. A. Abdel Maksoud, I. Y. Abdelrahman, F. M. Mosallam, M. Gobara and A. I. El-Batal, Biol. Trace Elem. Res., 2020, 196, 297–317 CrossRef CAS PubMed.
- K. Atacan, N. Güy, S. Çakar and M. Özacar, J. Photochem. Photobiol., A, 2019, 382, 111935 CrossRef CAS.
- X. He, A. Wang, P. Wu, S. Tang, Y. Zhang, L. Li and P. Ding, Sci. Total Environ., 2020, 140694 CrossRef CAS PubMed.
- F.-X. Xiao, S.-F. Hung, H. B. Tao, J. Miao, H. B. Yang and B. Liu, Nanoscale, 2014, 6, 14950–14961 RSC.
- H. Wang, L. Zhang, Z. Chen, J. Hu, S. Li, Z. Wang, J. Liu and X. Wang, Chem. Soc. Rev., 2014, 43, 5234–5244 RSC.
- M. Scarisoreanu, A. G. Ilie, E. Goncearenco, A. M. Banici, I. P. Morjan, E. Dutu, E. Tanasa, I. Fort, M. Stan and C. N. Mihailescu, Appl. Surf. Sci., 2020, 509, 145217 CrossRef CAS.
- M. Afsari, A. A. Youzbashi, H. Nuranian and S. M. Zahraee, Mater. Res. Bull., 2017, 94, 15–21 CrossRef CAS.
- N. R. Reddy, U. Bharagav, M. M. Kumari, K. K. Cheralathan, M. V. Shankar, K. R. Reddy, T. A. Saleh and T. M. Aminabhavi, J. Environ. Manage., 2020, 254, 109747 CrossRef CAS PubMed.
- H. S. Kibombo, R. Peng, S. Rasalingam and R. T. Koodali, Catal. Sci. Technol., 2012, 2, 1737–1766 RSC.
- J. Chen, F. Qiu, W. Xu, S. Cao and H. Zhu, Appl. Catal., A, 2015, 495, 131–140 CrossRef CAS.
- M. Bellardita, M. Addamo, A. Di Paola, G. Marcì, L. Palmisano, L. Cassar and M. Borsa, J. Hazard. Mater., 2010, 174, 707–713 CrossRef CAS PubMed.
- M. I. A. Abdel Maksoud, G. S. El-Sayyad, A. M. El-Khawaga, M. Abd Elkodous, A. Abokhadra, M. A. Elsayed, M. Gobara, L. I. Soliman, H. H. El-Bahnasawy and A. H. Ashour, J. Hazard. Mater., 2020, 399, 123000 CrossRef CAS PubMed.
- M. I. A. Abdel Maksoud, R. A. Fahim, A. E. Shalan, M. Abd Elkodous, S. O. Olojede, A. I. Osman, C. Farrell, A. A. H. Al-Muhtaseb, A. S. Awed, A. H. Ashour and D. W. Rooney, Environ. Chem. Lett., 2021, 19, 375–439 CrossRef CAS.
- W. Zhang, A. Sun, X. Pan, Y. Han, X. Zhao, L. Yu, Z. Zuo and N. Suo, J. Mater. Sci.: Mater. Electron., 2019, 30, 18729–18743 CrossRef CAS.
- A. Lassoued and J. F. Li, Solid State Sci., 2020, 104, 106199 CrossRef CAS.
- T. Sinha and M. Ahmaruzzaman, Photochem. Photobiol. Sci., 2016, 15, 1272–1281 CrossRef CAS PubMed.
- S. S. Al-Taweel and H. R. Saud, J. Chem. Pharm. Res., 2016, 8, 620–626 CAS.
- W. Yusmaman and J. Gunlazuardi, AIP Conf. Proc., 2020, 2243, 020033 CrossRef CAS.
- J. Long, J. Dong, X. Wang, Z. Ding, Z. Zhang, L. Wu, Z. Li and X. Fu, J. Colloid Interface Sci., 2009, 333, 791–799 CrossRef CAS PubMed.
- M. Arulprakasajothi, K. Elangovan, U. Chandrasekhar and S. Suresh, Therm. Sci., 2018, 22, 477–485 CrossRef.
- S. Chawla, N. Sankarraman and J. Payer, J. Electron Spectrosc. Relat. Phenom., 1992, 61, 1–18 CrossRef CAS.
- S. A. Abdullah, M. Z. Sahdan, N. Nayan, Z. Embong, C. R. C. Hak and F. Adriyanto, Mater. Lett., 2020, 263, 127143 CrossRef CAS.
- Y. Peng, P. Kan, Q. Zhang and Y. Zhou, Catalysts, 2019, 9, 558 CrossRef CAS.
- Y. Tu, S. Chen, X. Li, J. Gorbaciova, W. P. Gillin, S. Krause and J. Briscoe, J. Mater. Chem. C, 2018, 6, 1815–1821 RSC.
- Y. Zhang, J. Chen, L. Hua, S. Li, X. Zhang, W. Sheng and S. Cao, J. Hazard. Mater., 2017, 340, 309–318 CrossRef CAS PubMed.
- J. Luo, P. Lin, P. Zheng, X. Zhou, X. Ning, L. Zhan, Z. Wu, X. Liu and X. Zhou, Chemosphere, 2022, 298, 134297 CrossRef CAS PubMed.
- Z. Shen, Y. Zhou, Y. Guo, J. Zhao, J. Song, Y. Xie, Y. Ling and W. Zhang, Chin. Chem. Lett., 2021, 32, 2524–2528 CrossRef CAS.
- H. Nawaz, M. Umar, I. Nawaz, Q. Zia, M. Tabassum, H. Razzaq, H. Gong, X. Zhao and X. Liu, Chem. Eng. J., 2021, 419, 129542 CrossRef CAS.
- S. Banerjee and M. C. Chattopadhyaya, Arabian J. Chem., 2017, 10, S1629–S1638 CrossRef CAS.
- Y. Miyah, A. Lahrichi, M. Idrissi, S. Boujraf, H. Taouda and F. Zerrouq, J. Assoc. Arab Univ. Basic Appl. Sci., 2017, 23, 20–28 Search PubMed.
- H. Zhang, D. Liu, S. Ren and H. Zhang, Res. Chem. Intermed., 2017, 43, 1529–1542 CrossRef CAS.
- B.-M. Jun, S. S. Elanchezhiyan, Y. Yoon, D. Wang, S. Kim, S. Muthu Prabhu and C. M. Park, Chem. Eng. J., 2020, 393, 124733 CrossRef CAS.
- A. M. El-Khawaga, A. A. Farrag, M. A. Elsayed, G. S. El-Sayyad and A. I. El-Batal, J. Cluster Sci., 2021, 32, 1107–1119 CrossRef CAS.
- P. Hohenberg and W. Kohn, Phys. Rev., 1964, 136, B864 CrossRef.
-
M. J. Frisch, G. W. Trucks, H. B. Schlegel, G. E. Scuseria, M. A. Robb, J. R. Cheeseman, G. Scalmani, V. Barone, G. A. Petersson, H. Nakatsuji, X. Li, M. Caricato, A. V. Marenich, J. Bloino, B. G. Janesko, R. Gomperts, B. Mennucci, H. P. Hratchian, J. V. Ortiz, A. F. Izmaylov, J. L. Sonnenberg, D. Williams-Young, F. Ding, F. Lipparini, F. Egidi, J. Goings, B. Peng, A. Petrone, T. Henderson, D. Ranasinghe, V. G. Zakrzewski, J. Gao, N. Rega, G. Zheng, W. Liang, M. Hada, M. Ehara, K. Toyota, R. Fukuda, J. Hasegawa, M. Ishida, T. Nakajima, Y. Honda, O. Kitao, H. Nakai, T. Vreven, K. Throssell, J. A. Montgomery Jr., J. E. Peralta, F. Ogliaro, M. J. Bearpark, J. J. Heyd, E. N. Brothers, K. N. Kudin, V. N. Staroverov, T. A. Keith, R. Kobayashi, J. Normand, K. Raghavachari, A. P. Rendell, J. C. Burant, S. S. Iyengar, J. Tomasi, M. Cossi, J. M. Millam, M. Klene, C. Adamo, R. Cammi, J. W. Ochterski, R. L. Martin, K. Morokuma, O. Farkas, J. B. Foresman and D. J. Fox, Gaussian 16, Revision C.01, Gaussian, Inc., Wallingford CT, 2016 Search PubMed.
- M. H. N. Assadi and D. A. Hanaor, Appl. Surf. Sci., 2016, 387, 682–689 CrossRef CAS.
- M. Dorraj, B. T. Goh, N. A. Sairi, P. M. Woi and W. J. Basirun, Appl. Surf. Sci., 2018, 439, 999–1009 CrossRef CAS.
- K. P. Devi, S. A. Nisha, R. Sakthivel and S. K. Pandian, J. Ethnopharmacol., 2010, 130, 107–115 CrossRef CAS PubMed.
- A. Ashour, A. I. El-Batal, M. A. Maksoud, G. S. El-Sayyad, S. Labib, E. Abdeltwab and M. El-Okr, Particuology, 2018, 40, 141–151 CrossRef CAS.
- G. Sharmila, M. Thirumarimurugan and C. Muthukumaran, Microchem. J., 2019, 145, 578–587 CrossRef CAS.
- M. M. Naik, H. B. Naik, G. Nagaraju, M. Vinuth, H. R. Naika and K. Vinu, Microchem. J., 2019, 146, 1227–1235 CrossRef.
- W. Qing, K. Chen, Y. Yang, Y. Wang and X. Liu, Microchem. J., 2020, 152, 104262 CrossRef CAS.
- H. Rani, S. P. Singh, T. P. Yadav, M. S. Khan, M. I. Ansari and A. K. Singh, Mater. Chem. Phys., 2020, 239, 122052 CrossRef CAS.
- A. Samavati, M. Mustafa, A. Ismail, M. Othman and M. Rahman, Mater. Express, 2016, 6, 473–482 CrossRef CAS.
- L. F. Gaunt, C. B. Beggs and G. E. Georghiou, IEEE Trans. Plasma Sci., 2006, 34, 1257–1269 CAS.
- R. M. Fathy and A. Y. Mahfouz, J. Nanostruct. Chem., 2021, 1–21 Search PubMed.
- A. Joe, S.-H. Park, D.-J. Kim, Y.-J. Lee, K.-H. Jhee, Y. Sohn and E.-S. Jang, J. Solid State Chem., 2018, 267, 124–133 CrossRef CAS.
- N. Bradford, Anal. Biochem., 1976, 72, e254 CrossRef.
- S. Rajesh, V. Dharanishanthi and A. V. Kanna, J. Exp. Nanosci., 2015, 10, 1143–1152 CrossRef CAS.
- A. I. El-Batal, F. M. Mosallam and G. S. El-Sayyad, J. Cluster Sci., 2018, 29, 1003–1015 CrossRef CAS.
- M. Abd Elkodous, G. S. El-Sayyad, I. Y. Abdelrahman, H. S. El-Bastawisy, A. E. Mohamed, F. M. Mosallam, H. A. Nasser, M. Gobara, A. Baraka, M. A. Elsayed and A. I. El-Batal, Colloids Surf., B, 2019, 180, 411–428 CrossRef CAS PubMed.
- M. A. Maksoud, G. S. El-Sayyad, A. M. El-Khawaga, M. Abd Elkodous, A. Abokhadra, M. A. Elsayed, M. Gobara, L. Soliman, H. El-Bahnasawy and A. Ashour, J. Hazard. Mater., 2020, 399, 123000 CrossRef PubMed.
|
This journal is © The Royal Society of Chemistry 2022 |