DOI:
10.1039/D2NJ00296E
(Paper)
New J. Chem., 2022,
46, 6899-6920
Structural diversity and magnetic properties of copper(II) quinaldinate compounds with amino alcohols†
Received
19th January 2022
, Accepted 1st March 2022
First published on 1st March 2022
Abstract
The reactions between [Cu(quin)2(H2O)] (quin− = the anionic form of quinoline-2-carboxylic acid) and a series of aliphatic amino alcohols have yielded structurally very diverse copper(II) complexes, labelled a–g. Single-crystal X-ray structure analysis has revealed either intact amino alcohol molecules or amino alcoholate ions serving as ligands. In type a complexes, the amino alcohols are bound in a monodentate manner via NH2. Engagement of both functional groups in coordination was observed for types b and e (a bidentate chelating mode) and type c (a bidentate bridging one) complexes. In view of the strong bidentate chelating coordination of quinaldinate in [Cu(quin)2(H2O)], the formation of homoleptic amino alcohol complexes e was not anticipated. Equally surprising was the transformation of a mononuclear starting material into a one-dimensional (1D) coordination polymer, [Cu(quin)2]n (g). Spontaneous deprotonation of some amino alcohols and coordination of, thus formed, amino alcoholates via both donors also took place. Dinuclear complexes (d) contained two bridging amino alcoholates, whilst bidentate chelating mode was observed for type f. Interestingly, the dinuclear complex exists as two isomers which differ in the position of quinaldinates with respect to the Cu(μ-OR)2Cu core. DFT calculations on isolated syn- and anti-[Cu2(quin)2(3a1pO)2] (3a1pO− = anion of 3-amino-1-propanol) have shown the syn isomer to be more stable. The explanation lies in the intramolecular π⋯π stacking of quinaldinates, possible only in this isomer. Magnetic susceptibility measurements revealed antiferromagnetic interactions between S = 1/2 copper(II) spins in all the studied compounds except in [Cu(quin)2]n (g) for which weak ferromagnetic couplings are detected.
1. Introduction
The last two decades have witnessed remarkable progress in crystal engineering.1–3 As follows from its definition, the design of molecular solids with specific physical and chemical properties through understanding and control of intermolecular interactions,4,5 crystal engineering encompasses many aspects of classical coordination chemistry. The choice of the metal ion is crucial as the metal performs a functional role of the solid material through its redox, magnetic and optical properties. Copper(II) is one of the frequently employed metal ions in crystal engineering because of its versatile coordination behavior, redox and catalytic properties. It has a d9 electron configuration with geometric preferences based partly on LFSE (ligand field stabilization energy): it is normally coordinated by 4, 5 or 6 donors in square-planar, distorted square-pyramidal or, owing to Jahn–Teller distortion, axially distorted octahedral geometries.6 All the above properties combined with lower toxicity than most 4d and 5d transition metals7 make copper(II) a widespread ion in nature. It is present in active sites of many oxidation enzymes.8 The involvement of copper ions in electron and oxygen transport exploits the easy interconversion between the +1 and +2 oxidation states.9
Amino alcohols are a class of multidentate N,O-donor ligands that have also been used in crystal engineering. Both their functional groups, OH and NH2, are able to coordinate to transition metal ions. The OH moiety can give off its proton, producing anions which act as bridging and chelating ligands. Studies of copper(II) coordination chemistry with amino alcohols have shown that the OH group frequently undergoes spontaneous deprotonation.10 Another important characteristic of amino alcohols that makes them suitable for application in crystal engineering is their ability to participate in hydrogen bonding. The above listed properties combined with their commercial availability and low cost have strongly encouraged research on their coordination chemistry with transition metal ions including copper(II).11 Studies with a number of aliphatic amino alcohols have been performed so far, e.g., 2-aminoethanol,12N,N′-dimethylethanolamine,13 3-amino-1-propanol,10,12,14–20 diethanolamine,21–23N-methyldiethanolamine,24–26N-butyldiethanolamine,13 and triethanolamine.27,28 A survey of the Cambridge Structural Database reveals that there are more complexes with amino alcoholate ions than with intact amino alcohols.29 A recent review describes the structural diversity of copper(II) amino alcoholate complexes.30 The most common structural type encountered is Cu(μ-OR)2Cu, a dinuclear core with copper(II) ions bridged by two amino alcoholate (−OR) oxygen atoms. Owing to their rigid behavior, the dinuclear cores served as building units in the construction of metal–organic frameworks (MOFs)31,32 whose dimensionality was controlled through the denticity of the parent amino alcohol.23 It is noteworthy that some copper(II) amino alcoholate complexes have found application in catalysis,33 as exemplified by their catalytic role in the oxidative transformation of alkanes.34–36
We reported recently on the reactions of zinc(II) quinaldinate with two amino alcohols, 3-amino-1-propanol and 2-methylaminoethanol.37 Through the use of quinaldinate (abbreviated throughout the text as quin−), the anionic form of quinaldinic acid (Scheme 1), which usually binds in a N,O-chelating manner and thereby occupies two binding sites in the metal's coordination sphere, the possible reaction outcomes were highly limited. As a follow-up, zinc(II) was replaced with copper(II) which is known for its redox activity. Another reason for choosing copper(II) was that in spite of its radically different chemical behavior, copper(II) forms many homologous compounds to zinc(II). The study was expanded also by using a larger assortment of amino alcohols. Despite the recognized use of many amino alcohols, their combination with quinaldinate in copper(II) coordination chemistry has not been explored and no copper(II) compounds containing both ligands have been documented. The selected amino alcohols include primary amines (3-amino-1-propanol, 2-aminoethanol, 1-amino-2-propanol, 1-amino-2-methyl-2-propanol, 1-amino-2-butanol, 2-amino-1-propanol, 2-amino-2-methyl-1-propanol, 2-amino-1-butanol), secondary amines (2-methylaminoethanol and 2-ethylaminoethanol) and a tertiary amine (2-dimethylaminoethanol). The latter three amino alcohols were chosen in order to explore the influence of an alkyl substituent on nitrogen atoms over their coordination behavior. The structural formulae of the used amino alcohols, their names and the abbreviations are given in Scheme 2. Herein, we report on the products of three-component copper(II)–quinaldinate–amino alcohol systems. Their detailed characterization by single-crystal X-ray diffraction analyses and infrared vibrational spectroscopy is presented. For convenience, the novel compounds are classified into seven structural types, labelled a to g (Scheme 3). Type a denotes trans-[Cu(quin)2L2] (L = amino alcohol) complexes. Although type b and c share the same composition, [Cu(quin)2L], they differ in the amino alcohol binding mode and therefore in dimensionality. In a mononuclear type b complex, the amino alcohol adopts a bidentate chelating coordination mode, whereas in c with an infinite chain structure the amino alcohol serves as a bidentate bridging ligand. A type d dinuclear complex with bridging amino alcoholates exists in two isomeric forms, syn and anti, which differ in the quinaldinate position with respect to the Cu(μ-O)2Cu plane. Type e compounds with the [CuL3]2+ formula are homoleptic amino alcohol complexes, whereas type f is a homoleptic amino alcoholate complex with a metal ion in a square-planar environment. Finally, type g is a homoleptic quinaldinate complex with an infinite chain structure. With the exception of type c compounds, the magnetic properties were studied on at least one compound of each type. A correlation between their magnetic behavior and solid-state structures is presented. In addition, DFT calculations were performed on singlet and triplet states of syn- and anti-[Cu2(quin)2(3a1pO)2]. The calculations on selected 3a1pOH complexes were used to assess their stabilities.
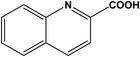 |
| Scheme 1 Structural formula of quinoline-2-carboxylic acid, also known as quinaldinic acid. | |
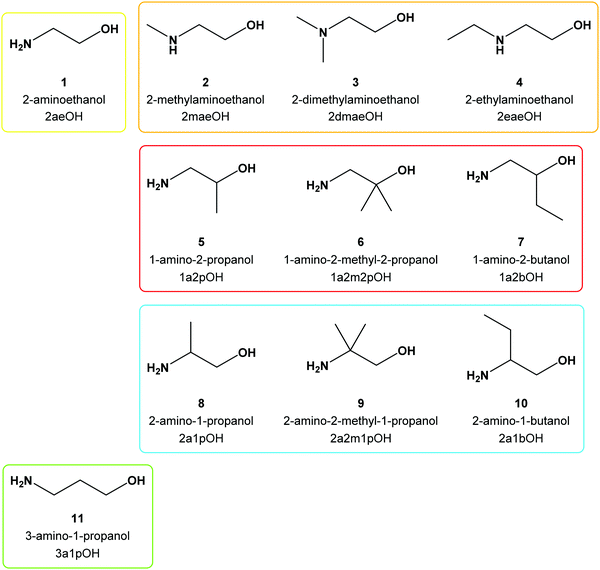 |
| Scheme 2 Amino alcohols used in this study. | |
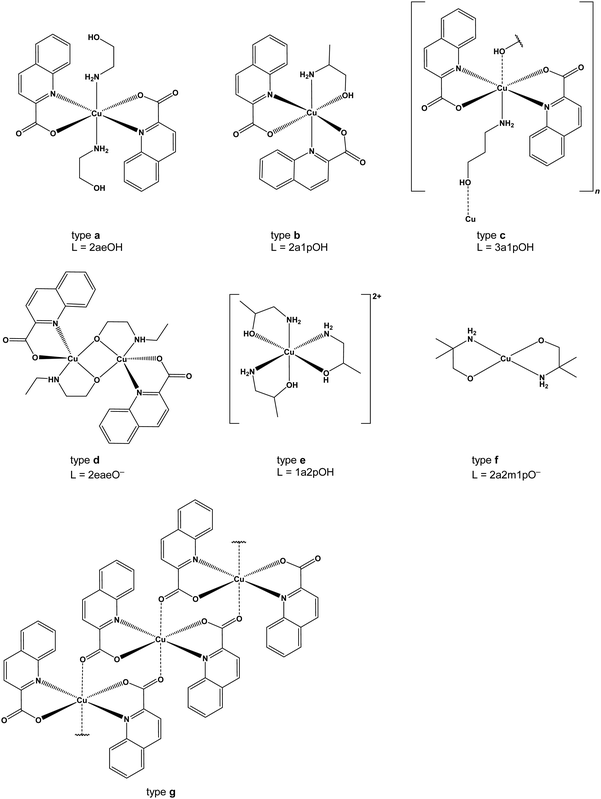 |
| Scheme 3 Structural formulae of novel complexes. L denotes the amino alcohol ligand. | |
2. Experimental
General
Acetonitrile, used as a solvent, was dried with molecular sieves.38 Other reagents were purchased from commercial sources and used as received. The synthetic procedure for the starting material [Cu(quin)2(H2O)] was the same as described previously.39 A Bruker Alpha II FT-IR spectrophotometer with an ATR module was used to record infrared spectra in the 4000–400 cm−1 spectral range. No manipulations were made on the IR spectra. Powder X-ray diffraction (PXRD) patterns for aged anti-11d·4MeOH were obtained using a PANanlytical X’Pert PRO MD diffractometer using monochromatised Cu-Kα radiation (λ = 1.5406 Å). A PerkinElmer 2400 II analyzer was used for elemental CHN analyses. Elemental analyses were not performed for 11a·3a1pOH, 11b, syn-11d, anti-11d·4MeOH, anti-11d·2MeCN, and anti-11d·2MeOH·2(3a1pOH) for three different reasons. 11a·3a1pOH, anti-11d·4MeOH, anti-11d·2MeCN, and anti-11d·2MeOH·2(3a1pOH) are solvates which are not stable outside of the mother liquor, 11b was obtained as a one-time event in a very small amount, whereas syn-11d was obtained in a mixture with other compounds. All novel compounds except syn-9d and syn-10d were characterized by X-ray structure analysis on single-crystals.
trans-[Cu(quin)2(2aeOH)2] (1a)
Procedure A. A Teflon container was filled with [Cu(quin)2(H2O)] (50 mg, 0.12 mmol), acetonitrile (7.5 mL) and 2-aminoethanol (1 mL). The container was closed and inserted into a steel autoclave, which was heated for 24 hours at 105 °C. Afterwards, the autoclave was taken out from the oven and left overnight to cool to room temperature. Green, needle-like crystals of trans-[Cu(quin)2(2aeOH)2] (1a) were filtered off. Yield: 41 mg, 66%. Procedure B. [Cu(quin)2(H2O)] (50 mg, 0.12 mmol), acetonitrile (7.5 mL) and 2-aminoethanol (1 mL) were added to an Erlenmeyer flask. The flask was closed and left to stand under ambient conditions. On the following day, blue crystalline precipitate was filtered off and air-dried. Yield: 53 mg, 85%. Please note that the same product formed at 4 °C. IR (ATR, cm−1): 3284s, 3156w, 3110w, 3087w, 3063w, 2971w, 2958m, 2859w, 2715w, 1604vs, 1587vs, 1562s, 1550s, 1505m, 1461s, 1430m, 1372vs, 1345s, 1301w, 1268w, 1254m, 1218m, 1206w, 1174s, 1152m, 1142w, 1117w, 1079s, 1034vvs, 1021s, 994w, 957m, 896m, 881m, 859s, 854s, 810s, 798s, 777vvs, 748s, 708s, 632s, 607vs, 601vvs, 576s, 548m, 522m, 494s, 482m, 443w, 413m. Elemental analysis calcd. for C24H26CuN4O6 (%): C, 54.39; H, 4.94; N, 10.57. Found (%): C, 54.03; H, 4.73; N, 10.72.
[Cu(quin)2(2maeOH)] (2b)
Procedure A. A Teflon container was filled with [Cu(quin)2(H2O)] (50 mg, 0.12 mmol), acetonitrile (7.5 mL) and 2-methylaminoethanol (0.25 mL). The container was closed and inserted into a steel autoclave, which was heated for 24 hours at 105 °C. Afterwards, the autoclave was taken out from the oven and left overnight to cool to room temperature. Green crystals of [Cu(quin)2(2maeOH)] (2b) were filtered off. Yield: 50 mg, 88%. Procedure B. [Cu(quin)2(H2O)] (50 mg, 0.12 mmol), acetonitrile (7.5 mL) and 2-methylaminoethanol (0.25 mL) were added to an Erlenmeyer flask. The flask was closed and left to stand under ambient conditions. On the following day, blue crystalline solid was filtered off and air-dried. Yield: 51 mg, 90%. Please note that the same product formed at 4 °C. IR (ATR, cm−1): 3378w, 3211m, 3103w, 3054w, 3007w, 2970w, 2904w, 2850w, 1640s, 1594m, 1564m, 1509w, 1484w, 1461m, 1415w, 1362vs, 1343s, 1295m, 1268m, 1216m, 1183m, 1172m, 1150m, 1112w, 1094w, 1058m, 1029s, 979w, 969w, 958w, 899s, 877m, 850m, 800vs, 780vvs, 662m, 640m, 628s, 606s, 563w, 550m, 522m, 497s, 481m, 413m. Elemental analysis calcd for C23H21CuN3O5 (%): C, 57.20; H, 4.38; N, 8.70. Found (%): C, 57.12; H, 4.21; N, 8.76.
[Cu(quin)2]n (g)
A Teflon container was filled with [Cu(quin)2(H2O)] (50 mg, 0.12 mmol), acetonitrile (7.5 mL) and 2-dimethylaminoethanol (0.1 mL). The container was closed and inserted into a steel autoclave, which was heated for 24 hours at 105 °C. Afterwards, the autoclave was taken out from the oven and left overnight to cool to room temperature. Green crystals of [Cu(quin)2]n (g) were filtered off and air-dried. Yield: 39 mg, 81%. Please note that the reaction without the amino alcohol yielded [Cu(quin)2]n (g) with approximately the same yield. On prolonged standing in the solution, compound g converted into a starting material, [Cu(quin)2(H2O)]. IR (ATR, cm−1): 3132w, 3102w, 3080w, 3051w, 3015w, 1637s, 1596m, 1567m, 1556m, 1510m, 1463m, 1436w, 1383s, 1372s, 1357s, 1274m, 1211m, 1202m, 1187m, 1148m, 1112w, 1026m, 1000w, 966m, 904m, 881m, 853s, 805vs, 767vvs, 741s, 735s, 641s, 633s, 611s, 576m, 522m, 493vs, 479m, 436s, 405s. Elemental analysis calcd for C20H12CuN2O4 (%): C, 58.90; H, 2.97; N, 6.87. Found (%): C, 58.82; H, 2.93; N, 6.77.
anti-[Cu2(quin)2(2eaeO)2] (anti-4d)
Procedure A. A Teflon container was filled with [Cu(quin)2(H2O)] (50 mg, 0.12 mmol), acetonitrile (7.5 mL) and 2-ethylaminoethanol (1 mL). The container was closed and inserted into a steel autoclave, which was heated for 24 hours at 105 °C. Afterwards, the autoclave was taken out from the oven and left overnight to cool to room temperature. Green needle-like crystals of anti-[Cu2(quin)2(2eaeO)2] (anti-4d) were filtered off. Yield: 14 mg, 37%. Procedure B. [Cu(quin)2(H2O)] (50 mg, 0.12 mmol), acetonitrile (7.5 mL) and 2-ethylaminoethanol (0.5 mL) were added to an Erlenmeyer flask. The flask was closed and left to stand under ambient conditions. On the following day, green crystalline solid was filtered off and air-dried. Yield: 34 mg, 89%. Please note that the same product formed at 4 °C. IR (ATR, cm−1): 3183m, 3061w, 2975w, 2938m, 2876m, 2833m, 1636vvs, 1595m, 1566m, 1507w, 1464m, 1432w, 1360vs, 1306w, 1262w, 1219m, 1173s, 1153m, 1137w, 1094m, 1062s, 1032s, 993w, 974w, 961w, 929w, 912m, 897m, 876m, 848s, 800s, 781vs, 775vs, 738w, 629s, 600s, 553m, 522w, 490m, 450s, 412s. Elemental analysis calcd for C28H32Cu2N4O6 (%): C, 51.93; H, 4.98; N, 8.65. Found (%): C, 51.98; H, 4.88; N, 8.81.
[Cu(1a2pOH)3](quin)2 (5e)
Procedure A. A Teflon container was filled with [Cu(quin)2(H2O)] (50 mg, 0.12 mmol), acetonitrile (7.5 mL) and 1-amino-2-propanol (0.5 mL). The container was closed and inserted into a steel autoclave, which was heated for 24 hours at 105 °C. Afterwards, the autoclave was taken out from the oven and left overnight to cool to room temperature. Blue needle-like crystals of [Cu(1a2pOH)3](quin)2 (5e) were filtered off. Yield: 60 mg, 81%. Procedure B. [Cu(quin)2(H2O)] (50 mg, 0.12 mmol), acetonitrile (7.5 mL) and 1-amino-2-propanol (0.5 mL) were added to an Erlenmeyer flask. The flask was closed and left to stand under ambient conditions. On the following day, blue crystalline solid was filtered off and air-dried. Yield: 72 mg, 97%. Please note that the same product formed at 4 °C. IR (ATR, cm−1): 3236m, 3122m, 2971w, 2963w, 2927w, 2873w, 1829w, 1615s, 1595m, 1581s, 1553s, 1500m, 1462m, 1424m, 1380s, 1369vs, 1341s, 1300m, 1213w, 1199w, 1171s, 1145w, 1117s, 1108s, 1090s, 1079s, 1058m, 1026s, 993w, 956w, 925w, 912w, 890m, 876w, 856m, 832w, 809s, 777vvs, 739s, 707m, 644m, 627m, 595s, 565w, 548w, 535w, 520m, 500w, 485m, 476m, 408w. Elemental analysis calcd for C29H39CuN5O7 (%): C, 55.01; H, 6.21; N, 11.06. Found (%): C, 54.96; H, 6.11; N, 11.11.
trans-[Cu(quin)2(1a2m2pOH)2] (6a)
Procedure A. A Teflon container was filled with [Cu(quin)2(H2O)] (50 mg, 0.12 mmol), acetonitrile (7.5 mL) and 1-amino-2-methyl-2-propanol (0.25 mL). The container was closed and inserted into a steel autoclave, which was heated for 24 hours at 105 °C. Afterwards, the autoclave was taken out from the oven and left overnight to cool to room temperature. Blue needle-like crystals of trans-[Cu(quin)2(1a2m2pOH)2] (6a) were filtered off. Yield: 62 mg, 90%. Procedure B. [Cu(quin)2(H2O)] (50 mg, 0.12 mmol), acetonitrile (7.5 mL) and 1-amino-2-methyl-2-propanol (0.5 mL) were added to an Erlenmeyer flask. The flask was closed and left to stand under ambient conditions. On the following day, blue crystalline solid was filtered off and air-dried. Yield: 66 mg, 96%. Please note that the same product formed at 4 °C. IR (ATR, cm−1): 3323m, 3244w, 3148w, 3060w, 2974m, 2964w, 2933w, 1609s, 1587s, 1561s, 1548m, 1505m, 1461m, 1430w, 1404m, 1379s, 1369s, 1336s, 1265m, 1250m, 1217m, 1178s, 1142s, 1105s, 1037s, 1018m, 997m, 971m, 958m, 932w, 907m, 896s, 878m, 869m, 859m, 808s, 798s, 774vvs, 745s, 672m, 632vs, 601vs, 589s, 547m, 522m, 495m, 481m, 415w. Elemental analysis calcd for C28H34CuN4O6 (%): C, 57.38; H, 5.85; N, 9.56. Found (%): C, 57.18; H, 5.78; N, 9.54.
trans-[Cu(quin)2(1a2bOH)2] (7a)
A Teflon container was filled with [Cu(quin)2(H2O)] (50 mg, 0.12 mmol), acetonitrile (7.5 mL), triethylamine (0.25 mL) and 1-amino-2-butanol (0.5 mL). The container was closed and inserted into a steel autoclave, which was heated for 24 hours at 105 °C. Afterwards, the autoclave was taken out from the oven and left overnight to cool to room temperature. Green needle-like crystals of trans-[Cu(quin)2(1a2bOH)2] (7a) were filtered off. Yield: 49 mg, 71%. IR (ATR, cm−1): 3431w, 3260m, 3166w, 3078w, 3061w, 2970w, 2957m, 2939w, 2874w, 1594s, 1562s, 1549s, 1506m, 1461s, 1430w, 1370s, 1343s, 1325s, 1281m, 1265m, 1216w, 1170s, 1151m, 1137m, 1115m, 1088m, 1075m, 1062m, 1033m, 986m, 961s, 897m, 879m, 859m, 840w, 802s, 774vvs, 742s, 707m, 632s, 601vs, 551m, 521m, 496s, 480m, 441w, 428w. Elemental analysis calcd for C28H34CuN4O6 (%): C, 57.38; H, 5.85; N, 9.56. Found (%): C, 57.53; H, 5.74; N, 9.70.
[Cu(1a2bOH)3](quin)2 (7e)
[Cu(quin)2(H2O)] (50 mg, 0.12 mmol), acetonitrile (7.5 mL) and 1-amino-2-butanol (0.5 mL) were added to an Erlenmeyer flask. The flask was closed and left to stand under ambient conditions. On the following day, blue crystalline solid, [Cu(1a2bOH)3](quin)2 (7e), was filtered off and air-dried. Yield: 75 mg, 95%. Please note that the same product formed at 4 °C. IR (ATR, cm−1): 3263m, 3212m, 3139m, 2964m, 2933m, 2875m, 1836w, 1613s, 1594m, 1578m, 1550s, 1500m, 1462s, 1425m, 1383s, 1367vs, 1340s, 1312m, 1249w, 1215w, 1202w, 1170s, 1146w, 1110m, 1097m, 1053m, 1038s, 1019m, 981s, 955m, 919w, 890w, 881w, 856m, 809s, 779vvs, 743s, 700m, 645m, 627m, 594s, 556m, 548m, 521m, 498m, 478m, 459w, 430w. Elemental analysis calcd for C32H45CuN5O7 (%): C, 56.92; H, 6.72; N, 10.37. Found (%): C, 56.53; H, 6.66; N, 10.48.
Reactions with 2a1pOH (8)
The reactions with 2-amino-1-propanol are not reproducible. Furthermore, mixtures of products can form. The procedures listed below yielded a certain product at least once.
[Cu(quin)2(2a1pOH)] (8b)
A Teflon container was filled with [Cu(quin)2(H2O)] (50 mg, 0.12 mmol), acetonitrile (7.5 mL) and 2-amino-1-propanol (0.15 mL). The container was closed and inserted into a steel autoclave, which was heated for 24 hours at 105 °C. Afterwards, the autoclave was taken out from the oven and left overnight to cool to room temperature. Crystalline solid, [Cu(quin)2(2a1pOH)] (8b), was filtered off and air-dried. Yield: 46 mg, 81%. IR (ATR, cm−1): 3376w, 3343w, 3226w, 3140w, 3102w, 3056w, 3009w, 2957w, 2932w, 2877w, 1641s, 1592m, 1565m, 1512w, 1459s, 1429w, 1361vs, 1342s, 1297m, 1275w, 1264w, 1251w, 1217m, 1183m, 1173s, 1149m, 1114m, 1053s, 1039s, 969w, 958w, 919w, 899s, 883w, 850m, 842m, 800vs, 781vvs, 678m, 640m, 629s, 606s, 558m, 521m, 498s, 486m, 479m, 461w, 431m, 414m. Elemental analysis calcd for C23H21CuN3O5 (%): C, 57.20; H, 4.38; N, 8.70. Found (%): C, 57.15; H, 4.23; N, 8.47.
[Cu(quin)2(2a1pOH)]n (8c)
[Cu(quin)2(H2O)] (50 mg, 0.12 mmol), acetonitrile (6.5 mL), methanol (1 mL) and 2-amino-1-propanol (0.25 mL) were added to an Erlenmeyer flask. The mixture was stirred thoroughly until all the solid was consumed. The resulting blue solution was left to stand under ambient conditions. After a period of time, crystals of [Cu(quin)2(2a1pOH)]n (8c) and syn-[Cu2(quin)2(2a1pO)2] (syn-8d) were obtained. IR (ATR, cm−1): 3302w, 3214w, 3133w, 3099w, 3052w, 2987w, 2973w, 2953w, 2907w, 2823w, 1641s, 1624s, 1592s, 1564m, 1505w, 1457m, 1427w, 1359s, 1341s, 1258m, 1252m, 1216m, 1169m, 1153m, 1141m, 1119m, 1076s, 1060s, 1021w, 964m, 955w, 917w, 897m, 880m, 852m, 801vs, 778vvs, 640s, 629s, 608s, 599s, 570m, 523m, 494m, 428s, 406m. Elemental analysis calcd for C23H21CuN3O5 (%): C, 57.20; H, 4.38; N, 8.70. Found (%): C, 57.02; H, 4.19; N, 8.66.
syn-[Cu2(quin)2(2a1pO)2] (syn-8d)
[Cu(quin)2(H2O)] (50 mg, 0.12 mmol), acetonitrile (6 mL), methanol (1.5 mL), triethylamine (0.25 mL) and 2-amino-1-propanol (0.25 mL) were added to an Erlenmeyer flask. The mixture was stirred thoroughly until all the solid was consumed. The resulting blue solution was left to stand under ambient conditions. After a period of time, a small amount of crystals of syn-[Cu2(quin)2(2a1pO)2] (syn-8d) was obtained. IR (ATR, cm−1): 3323w, 3251s, 3145m, 3066w, 3050w, 2955m, 2917m, 2875m, 2841w, 2804w, 2711w, 1635vvs, 1596m, 1567s, 1556m, 1508m, 1462m, 1431w, 1352vs, 1304m, 1276m, 1262w, 1216w, 1204w, 1175s, 1148m, 1116m, 1070s, 1047s, 1020m, 966m, 955w, 938w, 922w, 896m, 876m, 853s, 801vs, 771vvs, 742s, 716s, 661w, 634s, 626m, 603s, 574m, 556m, 535m, 521m, 495s, 457s, 428s. Elemental analysis calcd for C26H28Cu2N4O6 (%): C, 50.40; H, 4.56; N, 9.04. Found (%): C, 50.28; H, 4.56; N, 8.94.
[Cu(2a1pOH)3](quin)2 (8e)
Procedure A. [Cu(quin)2(H2O)] (50 mg, 0.12 mmol), acetonitrile (7.5 mL) and 2-amino-1-propanol (0.25 mL) were added to an Erlenmeyer flask. The flask was closed and left to stand under ambient conditions. On the following day, blue crystalline solid, [Cu(2a1pOH)3](quin)2 (8e), was filtered off and air-dried. Yield: 58 mg, 78%. Procedure B. A Teflon container was filled with [Cu(quin)2(H2O)] (50 mg, 0.12 mmol), acetonitrile (7.5 mL) and 2-amino-1-propanol (0.5 mL). The container was closed and inserted into a steel autoclave, which was heated for 24 hours at 105 °C. Afterwards, the autoclave was taken out from the oven and left overnight to cool to room temperature. The resulting green solution was stored at 4 °C. Crystals of 8e were filtered off after a few days. Yield: 46 mg, 62%. IR (ATR, cm−1): 3213m, 3123m, 2962w, 2926w, 2881w, 1835w, 1606s, 1557s, 1500m, 1460m, 1424m, 1365vs, 1337s, 1296m, 1245w, 1212m, 1199w, 1167m, 1143w, 1109s, 1070m, 1043s, 989w, 955m, 919w, 890m, 878m, 855m, 838w, 806s, 778vvs, 740s, 680m, 656m, 627s, 593s, 552m, 521m, 476m, 455m, 406w. Elemental analysis calcd for C29H39CuN5O7 (%): C, 55.01; H, 6.21; N, 11.06. Found (%): C, 54.78; H, 6.12; N, 10.73.
syn-[Cu2(quin)2(2a2m1pO)2] (syn-9d)
[Cu(quin)2(H2O)] (50 mg, 0.12 mmol), acetonitrile (6 mL), methanol (1.5 mL) and 2-amino-2-methyl-1-propanol (0.5 mL) were added to an Erlenmeyer flask. The mixture was stirred thoroughly until all the solid was consumed. The resulting blue solution was left to stand under ambient conditions. After a few days, a small amount of crystalline syn-[Cu2(quin)2(2a2m1pO)2] (syn-9d) was obtained. IR (ATR, cm−1): 3255m, 3145m, 3060w, 2971w, 2951w, 2905w, 2871w, 2835w, 1638vvs, 1597m, 1567m, 1506w, 1462m, 1361vs, 1302w, 1255w, 1231m, 1188m, 1172m, 1148m, 1129m, 1068m, 1047s, 1019m, 983m, 958w, 924w, 897m, 875w, 853s, 804s, 772vs, 738m, 707m, 627s, 602m, 545m, 522m, 499m, 444s, 413s. Elemental analysis calcd for C28H32Cu2N4O6 (%): C, 51.93; H, 4.98; N, 8.65. Found (%): C, 51.89; H, 5.06; N, 8.88.
[Cu(2a2m1pO)2](2a2m1pOHH)2(quin)2 (9f)
Procedure A. [Cu(quin)2(H2O)] (50 mg, 0.12 mmol), acetonitrile (7.5 mL) and 2-amino-2-methyl-1-propanol (0.5 mL) were added to an Erlenmeyer flask. The flask was closed and left to stand under ambient conditions. On the following day, a mixture of blue crystalline solid, syn-9d, and colorless needle-like crystals of 2a2m1pOHH+guin− (2a2m1pOHH+ = a cationic form of 2-amino-2-methyl-1-propanol with a NH3+ moiety) was obtained. 2a2m1pOHH+quin− crystallized in two polymorphic modifications. After a few days, only purple crystals of [Cu(2a2m1pO)2](2a2m1pOHH)2(quin)2 (9f) were left in the flask. The purple solid was filtered off. Yield: 83 mg, 92%. Procedure B. A Teflon container was filled with [Cu(quin)2(H2O)] (50 mg, 0.12 mmol), acetonitrile (6 mL), methanol (1.5 mL) and 2-amino-2-methyl-1-propanol (0.5 mL). The container was closed and inserted into a steel autoclave, which was heated for 24 hours at 105 °C. Afterwards, the autoclave was taken out from the oven and left overnight to cool to room temperature. The resulting green solution was concentrated under reduced pressure on a rotary evaporator. A glass vial with diethyl ether was carefully inserted into the Erlenmeyer flask with the concentrate. After a few days, purple crystals of 9f grew from the solution. Yield: 73 mg, 81%. IR (ATR, cm−1): 3315m, 3213w, 3076m, 2982m, 2957m, 2869m, 2845m, 2792m, 2743m, 2610m, 2550m, 2512m, 2110w, 1644m, 1602s, 1582s, 1553s, 1501m, 1471m, 1464m, 1423w, 1384s, 1372vs, 1326w, 1297w, 1275m, 1260w, 1222w, 1211w, 1190m, 1169m, 1147m, 1100w, 1055vvs, 1010s, 984m, 975m, 954w, 928w, 913w, 903w, 890m, 870w, 852m, 802s, 786vvs, 775vvs, 738m, 690m, 637w, 626s, 608s, 593m, 564w, 521m, 474s, 458s, 442s, 422m. Elemental analysis calcd for C36H56CuN6O8 (%): C, 56.56; H, 7.38; N, 10.99. Found (%): C, 56.50; H, 7.43; N, 10.98.
syn-[Cu2(quin)2(2a1bO)2] (syn-10d)
[Cu(quin)2(H2O)] (50 mg, 0.12 mmol), acetonitrile (7.5 mL) and 2-amino-1-butanol (0.25 mL) were added to an Erlenmeyer flask. The flask was closed and left to stand under ambient conditions. On the following day, a small amount of crystalline solid, syn-[Cu2(quin)2(2a1bO)2] (syn-10d), was filtered off and air-dried. Note. On prolonged standing in solution, the salt, 2a1bOHH+quin− (2a1bOHH+ = a cationic form of 2-amino-1-butanol with a NH3+ moiety), precipitated. 2a1bOHH+quin− crystallized in two polymorphic modifications. IR (ATR, cm−1): 3255s, 3143m, 3051w, 2965m, 2920m, 2896m, 2880m, 2837m, 2806w, 2701w, 1636vvs, 1594m, 1567s, 1506m, 1460s, 1351vs, 1261w, 1214w, 1172s, 1147m, 1128m, 1105m, 1075s, 1019m, 987m, 964m, 954w, 930w, 896m, 874m, 867w, 851s, 801s, 770vs, 739s, 719s, 634s, 602s, 555m, 538m, 521m, 493s, 467m, 434s. Elemental analysis calcd for C28H32Cu2N4O6 (%): C, 51.93; H, 4.98; N, 8.65. Found (%): C, 51.31; H, 4.58; N, 8.70.
[Cu(2a1bOH)3](quin)2 (10e)
[Cu(quin)2(H2O)] (50 mg, 0.12 mmol), acetonitrile (6 mL), methanol (1.5 mL) and 2-amino-1-butanol (0.5 mL) were added to an Erlenmeyer flask. The mixture was stirred thoroughly until all the solid was consumed. The resulting blue solution was left to stand under ambient conditions. On the following day, the solution was concentrated under reduced pressure on a rotary evaporator. A glass vial with diethyl ether was carefully inserted into the Erlenmeyer flask with the concentrate. Typically, a mixture of blue crystals of [Cu(2a1bOH)3](quin)2 (10e), syn-10d and/or 2a1bOHH+quin− was obtained. IR (ATR, cm−1): 3218m, 3125m, 2963m, 2934m, 2877w, 1840w, 1594s, 1557s, 1500m, 1460s, 1423m, 1366vs, 1337s, 1247w, 1211w, 1199w, 1167s, 1144m, 1108m, 1095m, 1044vs, 997m, 953m, 922w, 889m, 880m, 854s, 806s, 778vvs, 741s, 626s, 591s, 550w, 521m, 486m, 477m, 437w. Elemental analysis calcd for C32H45CuN5O7 (%): C, 56.92; H, 6.72; N, 10.37. Found (%): C, 56.74; H, 6.74; N, 10.21.
trans-[Cu(quin)2(3a1pOH)2] (11a)
[Cu(quin)2(H2O)] (50 mg, 0.12 mmol), acetonitrile (7.5 mL) and 3-amino-1-propanol (150 mg) were added to an Erlenmeyer flask. The flask was closed and left to stand under ambient conditions. On the following day, blue needle-like crystals of trans-[Cu(quin)2(3a1pOH)2] (11a) were filtered off. Yield: 58 mg, 89%. IR (ATR, cm−1): 3384m, 3329m, 3224m, 3052w, 2959w, 2943w, 2914w, 2869w, 2858w, 2815w, 1623s, 1595m, 1559m, 1548m, 1504w, 1480w, 1459m, 1432m, 1408w, 1385s, 1371vs, 1342s, 1263m, 1213m, 1174m, 1161m, 1146m, 1051s, 1030m, 1021m, 995s, 983m, 961m, 899s, 875w, 855m, 810s, 802s, 773vvs, 738s, 629vs, 618s, 600s, 560m, 520s, 495s, 476m, 458m. Elemental analysis calcd for C26H30CuN4O6 (%): C, 55.96; H, 5.42; N, 10.04. Found (%): C, 55.68; H, 5.23; N, 10.11.
trans-[Cu(quin)2(3a1pOH)2]·3a1pOH (11a·3a1pOH)
[Cu(quin)2(H2O)] (25 mg, 0.06 mmol) and acetonitrile (7.5 mL) were added to an Erlenmeyer flask. After the contents were cooled to 4 °C, 3-amino-1-propanol (150 mg) was added. The reaction mixture was left to stand in a closed vessel at 4 °C for approximately 1 hour. Afterwards, blue crystalline solid, trans-[Cu(quin)2(3a1pOH)2]·3a1pOH (11a·3a1pOH), was filtered off. Yield: 29 mg. Please note that owing to the solvent molecules of 3a1pOH, the crystals of 11a·3a1pOH are not stable when taken out from the mother liquor. In the solution, 11a·3a1pOH converts into 11a within a day. IR (ATR, cm−1): 3359w, 3255m, 3175m, 3050w, 2950w, 2867w, 1634s, 1614s, 1588s, 1562s, 1504m, 1486w, 1462m, 1427w, 1391s, 1377s, 1359s, 1344s, 1299w, 1268w, 1215w, 1168m, 1153m, 1147m, 1109w, 1060s, 1044s, 1017m, 993m, 955w, 931m, 894s, 855m, 849m, 801vs, 775vvs, 745m, 672m, 630s, 599s, 550m, 521m, 500m, 482w.
[Cu(quin)2(3a1pOH)] (11b)
A Teflon container was filled with [Cu(quin)2(H2O)] (50 mg, 0.12 mmol), acetonitrile (7.5 mL) and 3-amino-1-propanol (150 mg). The container was closed and inserted into a steel autoclave, which was heated for 24 hours at 105 °C. Afterwards, the autoclave was taken out from the oven and left overnight to cool to room temperature. The resulting green solution was transferred to an Erlenmeyer flask which was left open for 1 day. Afterwards, the flask was stoppered. Within 24 hours, crystals of [Cu(quin)2(3a1pOH)] (11b) grew from the oil. Please note that 11b was obtained as a one-time event, otherwise 11a, 11a·3a1pOH or anti-11d·2MeCN formed. IR (ATR, cm−1): 3345m, 3233m, 3154m, 3104w, 3056w, 3011w, 2985w, 2943w, 2917w, 2879w, 1641s, 1594s, 1564s, 1506m, 1458s, 1435w, 1416w, 1362vs, 1342s, 1295m, 1259m, 1242m, 1217m, 1183m, 1173s, 1151m, 1112s, 1093m, 1046m, 1024w, 975s, 958m, 946s, 899s, 881m, 851s, 833w, 799s, 782vvs, 661s, 640s, 633s, 627s, 606s, 555m, 521s, 497s, 478m.
[Cu(quin)2(3a1pOH)]n (11c)
anti-[Cu2(quin)2(3a1pO)2]·4MeOH (50 mg), acetonitrile (6 mL), methanol (1.5 mL), quinaldinic acid (28 mg) and 3-amino-1-propanol (24 mg) were added to an Erlenmeyer flask. The contents were stirred at room temperature for 1 day. All the solid was consumed meanwhile. The resulting blue solution was stored at 4 °C for a few days. Crystals of [Cu(quin)2(3a1pOH)]n (11c) were filtered off and air-dried. Yield: 22 mg. IR (ATR, cm−1): 3335w, 3238w, 3154w, 3095w, 3071w, 2953w, 2928w, 2886w, 1644s, 1628s, 1598s, 1564m, 1506w, 1475w, 1460s, 1446w, 1360vs, 1343s, 1295w, 1257m, 1237w, 1217w, 1197w, 1180m, 1172m, 1154m, 1139m, 1081m, 1066s, 1034m, 994w, 963w, 956w, 901m, 881m, 858m, 816m, 803vs, 775vvs, 750m, 640m, 630m, 608s, 600m, 569w, 548w, 530w, 521m, 499m, 431m, 405m. Elemental analysis calcd for C23H21CuN3O5(%): C, 57.20; H, 4.38; N, 8.70. Found (%): C, 57.18; H, 4.30; N, 8.16.
syn-[Cu2(quin)2(3a1pO)2] (syn-11d)
A Teflon container was filled with [Cu(quin)2(H2O)] (50 mg, 0.12 mmol), acetonitrile (7.5 mL) and 3-amino-1-propanol (150 mg). The container was closed and inserted into a steel autoclave, which was heated for 24 hours at 105 °C. Afterwards, the autoclave was taken out from the oven and left overnight to cool to room temperature. The resulting green solution was concentrated under reduced pressure on a rotary evaporator. A glass vial with diethyl ether was carefully inserted into the Erlenmeyer flask with the concentrate. After a period of time, crystals of syn-[Cu2(quin)2(3a1pO)2] (syn-11d) and 11a deposited from the concentrate. Please note that syn-11d was obtained only once.
syn-[Cu2(quin)2(3a1pO)2]·2H2O (syn-11d·2H2O)
A Teflon container was filled with [Cu(quin)2(H2O)] (50 mg, 0.12 mmol), methanol (7.5 mL), triethylamine (0.5 mL) and 3-amino-1-propanol (150 mg). The container was closed and inserted into a steel autoclave, which was heated for 24 hours at 105 °C. Afterwards, the autoclave was taken out from the oven and left overnight to cool to room temperature. The resulting green solution was concentrated under reduced pressure on a rotary evaporator. A glass vial with diethyl ether was carefully inserted into the Erlenmeyer flask with the concentrate. After a period of time, crystals of syn-[Cu2(quin)2(3a1pO)2]·2H2O (syn-11d·2H2O), syn-11d and 11a deposited from the concentrate. Please note that a more reliable way of preparing syn-11d·2H2O proved to be by powdering a sample of anti-11d·4MeOH. The powdered material was left to stand in the air for at least 1 day. IR (ATR, cm−1): 3469m, 3271m, 3160m, 3058w, 2936w, 2906w, 2885w, 2806m, 2698w, 1633vs, 1615vs, 1594s, 1563s, 1506m, 1460s, 1429w, 1372vs, 1343s, 1328m, 1306w, 1277w, 1260w, 1215w, 1180s, 1172s, 1148m, 1106w, 1085s, 1055s, 1017w, 985w, 956w, 932s, 896m, 883w, 877m, 852m, 802s, 778vvs, 741m, 718m, 658m, 642m, 633m, 596s, 520s, 494s, 450s. Elemental analysis calcd for C26H32Cu2N4O8 (%): C, 47.63; H, 4.92; N, 8.55. Found (%): C, 47.54; H, 4.72; N, 8.16.
anti-[Cu2(quin)2(3a1pO)2]·2MeCN (anti-11d·2MeCN)
Procedure A. [Cu(quin)2(H2O)] (50 mg, 0.12 mmol), acetonitrile (6 mL) and methanol (1.5 mL) were added to an Erlenmeyer flask. After, the contents were cooled to 4 °C, 3-amino-1-propanol (150 mg) was added. The reaction mixture was left to stand in a closed vessel at 4 °C. Blue-green crystals of anti-[Cu2(quin)2(3a1pO)2]·2MeCN (anti-11d·2MeCN) were obtained after a few days. Procedure B. A Teflon container was filled with [Cu(quin)2(H2O)] (50 mg, 0.12 mmol), acetonitrile (7.5 mL), triethylamine (0.5 mL) and 3-amino-1-propanol (150 mg). The container was closed and inserted into a steel autoclave, which was heated for 24 hours at 105 °C. Afterwards, the autoclave was taken out from the oven and left overnight to cool to room temperature. The resulting green solution was concentrated under reduced pressure on a rotary evaporator. The concentrate was transferred to an Erlenmeyer flask and left to stand under ambient conditions. Crystals of anti-11d·2MeCN were obtained after few days. Please note that crystals of anti-11d·2MeCN are not stable when taken out from the mother liquor. IR (ATR, cm−1): 3310m, 3219w, 3141w, 3056w, 3023w, 2983w, 2931w, 2876m, 2823w, 2708w, 2249w, 1630vs, 1619s, 1593m, 1560s, 1507m, 1461m, 1430m, 1397w, 1359vs, 1343s, 1301m, 1275m, 1260m, 1213w, 1178m, 1149s, 1112w, 1082s, 1059s, 1041m, 1021w, 969w, 956w, 930s, 885s, 858w, 821w, 811m, 779vvs, 745s, 684m, 641m, 633m, 596s, 521m, 501m, 486m, 434m, 407m.
anti-[Cu2(quin)2(3a1pO)2]·4MeOH (anti-11d·4MeOH)
[Cu(quin)2(H2O)] (200 mg, 0.47 mmol), methanol (7.5 mL) and 3-amino-1-propanol (300 mg) were added to an Erlenmeyer flask. The flask was closed and left to stand under ambient conditions. After a few minutes, all the solid was consumed and the solution acquired a green color. Large, dark blue crystals of anti-[Cu2(quin)2(3a1pO)2]·4MeOH (anti-11d·4MeOH) grew overnight. Yield: 141 mg. Please note that the crystals of anti-11d·4MeOH are not stable when removed from the mother liquor. In the air, they are converted into syn-11d·2H2O. IR (ATR, cm−1): 3332w, 3264m, 3237m, 3151m, 3068w, 2921m, 2874m, 2851w, 2721w, 1632s, 1619s, 1594m, 1562m, 1507m, 1459m, 1430m, 1404w, 1359s, 1342s, 1302w, 1287w, 1261m, 1212w, 1177m, 1167s, 1149m, 1113w, 1086s, 1063s, 1022vs, 971w, 954w, 928s, 891m, 885s, 857w, 820w, 810m, 779vvs, 742s, 703m, 641s, 632s, 595s, 553m, 522s, 503s, 489s, 438s, 408m.
Monitoring the conversion of anti-11d·4MeOH into syn-11d·2H2O with IR spectroscopy
Few, freshly prepared crystals of anti-11d·4MeOH were placed in an ATR sample holder. At the start of the experiment, the spectra were collected every 5 minutes. The last spectrum was recorded after 24 hours. The spectra are shown in the ESI† (Fig. S20). The identity of the product was confirmed by PXRD (Fig. S1, ESI†).
Reaction of anti-[Cu2(quin)2(3a1pO)2]·4MeOH with 3a1pOH
anti-11d·4MeOH (50 mg) was stirred in acetonitrile (7.5 mL) with quinaldinic acid (28 mg) and 3a1pOH (33 mg) at room temperature for 2 days. Crystalline 11a was filtered off. Yield: 60 mg.
anti-[Cu2(quin)2(3a1pO)2]·2MeOH·2(3a1pOH) (anti-11d·2MeOH·2(3a1pOH))
A Teflon container was filled with [Cu(quin)2(H2O)] (50 mg, 0.12 mmol), methanol (7.5 mL) and 3-amino-1-propanol (150 mg). The container was closed and inserted into a steel autoclave, which was heated for 24 hours at 105 °C. Afterwards, the autoclave was taken out from the oven and left overnight to cool to room temperature. The resulting green solution was concentrated under reduced pressure on a rotary evaporator. A glass vial with diethyl ether was carefully inserted into the Erlenmeyer flask with the concentrate. After a period of time, crystals of anti-11d·2MeOH·2(3a1pOH) grew from the concentrate. Please note that the crystals of anti-11d·2MeOH·2(3a1pOH) are not stable when taken out from the mother liquor. They were obtained only once. IR (ATR, cm−1): 3386w, 3341m, 3330m, 3271m, 3227m, 3152w, 3057w, 2927m, 2902w, 2866m, 2823w, 1628vs, 1593s, 1560s, 1505w, 1459m, 1431m, 1365vs, 1343s, 1304w, 1280w, 1262m, 1212w, 1167m, 1147m, 1113w, 1085m, 1051s, 995s, 983m, 958m, 933m, 899m, 893m, 885m, 855m, 810s, 803s, 793m, 774vvs, 738s, 705m, 640m, 630s, 619s, 597s, 560m, 520m, 495s, 458m, 436m.
X-ray structure analysis
Single crystal X-ray diffraction data were recorded using an Agilent SuperNova diffractometer with molybdenum (Mo-Kα, λ = 0.71073 Å) or copper (Cu-Kα, λ = 1.54184 Å) micro-focus sealed X-ray source at 150 K. The diffractometer was equipped with mirror optics and an Atlas detector. Silicone grease was used to place each crystal on a fiber glass tip, which was then mounted on the goniometer head. Data were processed using CrysAlis PRO.40 Structures were solved with Olex2 software41 using intrinsic phasing in ShelXT42 and refined with the least squares methods in ShelXL.43 Anisotropic displacement parameters were determined for all non-hydrogen atoms. Where possible, NH2, NH, NH3+ and OH hydrogen atoms of amino alcohols were located from a difference Fourier map and refined with isotropic displacement parameters. OH hydrogen atoms of water and methanol in anti-11d·4MeOH, syn-11d·2H2O and anti-11d·2MeOH·2(3a1pOH) were located from a difference Fourier map. In some structures, the hydrogen atoms of amino alcohol heteroatoms were added in calculated positions due to ligand disorder (7a, 8c, 11b and 11c), large number of atoms in the asymmetric unit (8e and 10e) or a poor-quality data set (syn-8d). The remaining hydrogen atoms were placed in geometrically calculated positions in all structures and refined using riding models. Disorder was observed for amino alcohol ligands in 2b, 5e, 7a, 7e, 8c, 8e, 10e, 11b and 11c. For 2b, the disorder over a two-fold rotation axis pertains also to copper(II) ion. For all, the disorder was resolved using the appropriate PART instruction. The programs Platon,44 Ortep45 and Mercury46 were used for crystal structure analysis and the preparation of figures. The crystallographic data are collected in Tables S1–S3 (ESI†). All crystal structures were deposited at the CCDC and were assigned deposition numbers 2076993 (1a), 2076994 (2b), 2076995 (g), 2076996 (anti-4d), 2076997 (5e), 2076998 (6a), 2076999 (7a), 2077000 (7e), 2077001 (8b), 2077002 (8c), 2077003 (syn-8d), 2077004 (8e), 2077005 (9f), 2077006 (10e), 2077007 (11a), 2077008 (11a·3a1pOH), 2077009 (11b), 2077010 (11c), 2077011 (syn-11d), 2077012 (syn-11d·2H2O), 2077013 (anti-11d·2MeCN), 2077014 (anti-11d·4MeOH), and 2077015 (anti-11d·2MeOH·2(3a1pOH)).
Computational details
Geometries of copper(II) complexes were optimized without constraints from X-ray diffraction data, using the Gaussian 09 software.47 Coordinates of complex molecules of 11a, 11b, syn-11d·2H2O and anti-11d·2MeCN were used. The initial geometry of 11a–L was derived from a repeating unit of 1D-polymer 11c. Vibrational analysis confirmed the nature of these structures as minima in the potential energy surface and was also used to obtain Zero Point and Gibbs free energies. The basis set 6-31+g(d,p)48,49 including diffusion and polarization was used for all atoms, except for Cu, which was described by means of the SDD basis and the associated electron core potential (ECP).50 The B3PW9151–53 functional with the D3 version of Grimme's dispersion with Becke–Johnson damping54 was used. Bulk solvent effects (acetonitrile) were included during optimization with the SMD continuum model.55 Relative energies of the triplet and antiferromagnetically coupled singlet states were assessed for the syn and anti isomers of [Cu2(quin)2(3a1pO)2] and the stabilities of the corresponding DFT wavefunctions were tested.56,57 Typically, RHF to UHF instabilities were found for wave functions obtained from spin-restricted (singlet) wavefunctions. When a more stable, spin-unrestricted, wavefunction was located, it was used as the initial guess for subsequent geometry optimization. Analysis of the electron density within the Atoms In Molecules (AIM) theory of R. F. W. Bader58 was performed with the Multiwfn program,59,60 which was also used for Non-Covalent Interaction (NCI) Analysis.61 The Cylview visualization software62 was used to prepare some of the figures.
Magnetic measurements
All stable compounds, that could be obtained in pure form and in sufficient quantities, were subjected to magnetic susceptibility measurements using a MPMS-XL Quantum Design SQUID magnetometer which works between 1.8 and 400 K for dc applied fields ranging from −7 to 7 T. Measurements were performed on analytically pure polycrystalline samples (25.91, 21.40, 22.34, 14.12, 19.13, 23.15, 20.09, 19.97, 20.34, 23.75, 18.15 and 18.30 mg for 1a, 2b, anti-4d, 5e, 6a, 7a, 7e, 8e, 9f, 11a, syn-11d·2H2O, and g, respectively) introduced in polyethylene bags (3 × 0.5 × 0.02 cm, weighing typically between 7 and 19 mg). Prior to the experiments, a M vs. H measurement was performed at 100 K to confirm the absence of ferromagnetic impurities. Consistent dc susceptibility and in-phase ac susceptibility have been obtained between 1.85 and 15 K. The data were corrected for the sample holder and the diamagnetic contribution of the sample.
3. Results and discussion
3.1. Synthetic considerations
In this study, a total of eleven different amino alcohols was reacted with the [Cu(quin)2(H2O)] precursor. The studied systems yielded six types of compounds, labelled a to f, which contained both the quinaldinate and the amino alcohol ligand. A homoleptic quinaldinate complex [Cu(quin)2]n, labelled g, was also obtained. All the novel products are listed in Scheme 4. The product abbreviation consists of a number which represents the amino alcohol and a letter which represents the compound type.
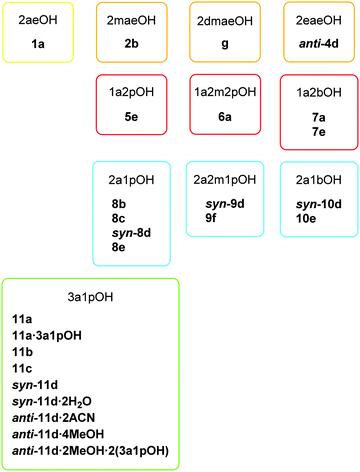 |
| Scheme 4 List of novel copper(II) compounds with their abbreviation consisting of a number which represents the used amino alcohol and a letter which represents the compound type. | |
Typically, a large excess of amino alcohol was used in the reaction. Such a ligand-to-metal ion ratio favors the formation of a complex with the maximum number of ligands. In our case, trans-[Cu(quin)2L2], a type a complex with two amino alcohol ligands L per metal ion, is obtained. Interestingly, only systems with 2-aminoethanol (1), 1-amino-2-methyl-2-propanol (6), 1-amino-2-butanol (7) and 3-amino-1-propanol (11) yielded such complexes. Our study shows that the amino alcohol ligands preferentially bind to copper(II) ions through their NH2 groups. The coordination behavior of the N-alkyl substituted amino alcohols, 2-methylaminoethanol (2), 2-dimethylaminoethanol (3) and 2-ethylaminoethanol (4), was thus expected to be drastically different. As mentioned below, the alkyl substituent on nitrogen neither prevents its coordination to copper(II) nor favors one coordination mode. For 2-dimethylaminoethanol (3) with two methyl substituents (the ligand with the greatest steric hindrance), no amino alcohol complex was isolated during the course of our work. Instead, a homoleptic quinaldinate complex, [Cu(quin)2]n (g) with an infinite chain structure, was obtained. A later rational synthesis of g ruled out 2-dimethylaminoethanol as a necessary reagent. It should be noted that many copper(II) complexes with 2-dimethylaminoethanol or its deprotonated form are known.29 In these complexes, 2dmaeOH adopts a bidentate chelating coordination. The ligand with one methyl group, 2-methylaminoethanol (2), coordinates to copper(II) in a bidentate chelating manner. The type b complex, [Cu(quin)2(2maeOH)] (2b), is the only species that could be isolated from this ligand system regardless of the reaction conditions. The 2-ethylaminoethanol (4) system also features a single product, a dinuclear anti-[Cu2(quin)2(2eaeO)2] (anti-4d) with a deprotonated amino alcohol which is coordinated through its alkoxide oxygen and amino moiety. No auxiliary strong base was necessary to assist in the deprotonation of amino alcohols. Again, anti-4d formed both under mild reaction conditions, 4 °C or room temperature, and at 105 °C in an autoclave.
A key information obtained from these eleven systems is that the nature of the product depends more upon the amino alcohol used than the applied reaction conditions. For example, 2-aminoethanol (1), 1-amino-2-propanol (5) and 1-amino-2-methyl-2-propanol (6), yielded in combination with the [Cu(quin)2(H2O)] starting material a single product, both under mild and under forcing reaction conditions. The amino alcohols 1 and 6 afforded type a complexes, [Cu(quin)2(2aeOH)2] (1a) and [Cu(quin)2(1a2m2pOH)2] (6a), whereas for 5, an ionic [Cu(1a2pOH)3](quin)2 (5e) was obtained. The formation of a homoleptic amino alcohol complex from [Cu(quin)2(H2O)] under mild conditions is surprising. The {Cu(quin)2} fragment was not expected to fall apart. Instead, in the presence of 1-amino-2-propanol (5), the copper(II) coordination sphere was almost instantaneously depleted of bidentate chelating quinaldinates. Each of the systems with 1-amino-2-butanol (7), 2-amino-1-propanol (8), 2-amino-2-methyl-1-propanol (9), 2-amino-1-butanol (10) and 3-amino-1-propanol (11) has led to more than one product. Reactions with 2-amino-2-methyl-1-propanol (9) afforded a dinuclear type d complex with a syn disposition of aromatic ligands, syn-[Cu2(quin)2(2a2m1pO)2] (syn-9d), and [Cu(2a2m1pO)2](2a2m1pOHH)2(quin)2 (9f), a co-crystal which contains a homoleptic amino alcoholate complex. The 2-amino-2-methyl-1-propanol system is the only one that produced the type f complex. Dinuclear complex syn-9d co-crystallized with the amino alcohol salt with quinaldinate, 2a2m1pOHH+quin− (2a2m1pOHH+ = a cationic form of amino alcohol with a NH3+ moiety). If the latter mixture was left undisturbed for a couple of days, both solids were consumed and a new phase, purple crystals of [Cu(2a2m1pO)2](2a2m1pOHH)2(quin)2 (9f) appeared. This sequence of events suggests the origin of 2a2m1pOHH+ and 2a2m1pO− ions. The following reaction implies a transfer of the OH proton from one amino alcohol molecule to the NH2 group in another:
2a2m1pOH + 2a2m1pOH ⇌ 2a2m1pOHH+ + 2a2m1pO− |
The coordination of thus formed 2a2m1pO− ions to copper(II) could be the driving force for this reaction. Once the amount of the amino alcoholate ions is large enough, a successive reaction, conversion of syn-9d into 9f, can take place:
syn-[Cu2(quin)2(2a2m1pO)2] + 4(2a2m1pOHH+) + 2(2a2m1pO−) + 2quin− → 2[Cu(2a2m1pO)2](2a2m1pOHH)2(quin)2 |
The 2-amino-1-butanol (10) system is another example with the confirmed formation of the 2a1bOHH+quin− salt. Similar to the 2-amino-2-methyl-1-propanol (9) ligand system, the salt and the dinuclear complex with amino alcoholate ligands, syn-10d, crystallized simultaneously.
The ligand systems of 2-amino-1-propanol (8) with four products and 3-amino-1-propanol (11) with nine products displayed the greatest structural diversity among the isolated products. The reactions with 8 turned out to be very erratic. Despite numerous and methodical attempts, no reproducible synthetic conditions necessary for the formation of pure [Cu(quin)2(2a1pOH)] (8b), [Cu(quin)2(2a1pOH)]n (8c), syn-[Cu2(quin)2(2a1pO)2] (syn-8d) or [Cu(2a1pOH)3](quin)2 (8e) could be established. The poor reproducibility of these reactions remains our most worrisome concern. Conversely, the 3-amino-1-propanol ligand system displays more control over the reaction outcome. The type a complex, [Cu(quin)2(3a1pOH)2] (11a), appears as the thermodynamic product. Several 3a1pOH and 3a1pO− compounds were shown to transform into 11a. The type a complex crystallizes also as a solvate, [Cu(quin)2(3a1pOH)2]·3a1pOH (11a·3a1pOH). The crystals of 11a·3a1pOH are not stable, neither in air nor in solution. In both cases, they transform into 11a. Interestingly, the 3a1pOH system yielded two mono amino alcohol compounds with the [Cu(quin)2(3a1pOH)] composition, a mononuclear 11b and a 1D-polymer 11c. Mononuclear 11b features a bidentate chelating amino alcohol with the hydroxyl-to-copper(II) bond distance being on the verge of the acceptable bonding interactions. 11b was obtained only once, otherwise a complex with two amino alcohol ligands per copper(II) ion formed. The reaction of 11b with the excess ligand confirms its metastable nature. In 1D-polymer 11c, the amino alcohol ligand is engaged in coordination both functional groups, yet to two metal ions. The composition of the reaction mixture that afforded 11c merits comment. anti-[Cu2(quin)2(3a1pO)2]·4MeOH (anti-11d·4MeOH) with a dinuclear core reacted with quinaldinic acid to form 11c in the mixture of 3-amino-1-propanol, acetonitrile and methanol. The rigidity of the dinuclear core in anti-11d·4MeOH was ruled out also by its conversion into 11a and its reaction with the air moisture to syn-[Cu2(quin)2(3a1pO)2]·2H2O (syn-11d·2H2O). The latter reaction is not surprising, as syn-11d·2H2O usually crystallized from reaction mixtures that contained water which was introduced unintentionally, for example, with triethylamine. The latter was added with the aim to assist in the deprotonation of amino alcohol. What makes the conversion of anti-11d·4MeOH into syn-11d·2H2O interesting is the isomerization that also takes place. The conversion was monitored by IR vibrational spectroscopy, whereas the identity of the final product was confirmed by PXRD (ESI,† Fig. S1). The 3a1pOH reaction system afforded three more dinuclear compounds, anti-[Cu2(quin)2(3a1pO)2]·2MeCN (anti-11d·2MeCN), syn-[Cu2(quin)2(3a1pO)2] (syn-11d) and anti-[Cu2(quin)2(3a1pO)2]·2MeOH·2(3a1pOH) (anti-11d·2MeOH·2(3a1pOH)). Their crystallization depends critically upon the content of solvents. The difficulties to control it, explain why, for example, the methanol/3-amino-1-propanol solvate, anti-11d·2MeOH·2(3a1pOH), was obtained only once. The closing remark pertains to the syn-/anti-isomerism observed for a dinuclear 3a1pO− complex. Since both isomers can crystallize from the same reaction mixture, the reaction conditions do not favor one isomer over another.
3.2. Description of crystal structures
The descriptions are ordered according to the structural types shown in Scheme 3. Most relevant geometric parameters are given in Tables 1–4. trans-[Cu(quin)2(2aeOH)2] (1a), trans-[Cu(quin)2(1a2m2pOH)2] (6a), trans-[Cu(quin)2(1a2bOH)2] (7a), trans-[Cu(quin)2(3a1pOH)2] (11a) and trans-[Cu(quin)2(3a1pOH)2]·3a1pOH (11a·3a1pOH) belong to type a complexes which have in common that copper(II) is coordinated by two bidentate chelating quinaldinates and two monodentate amino alcohol ligands bound via N-donor atom of the amino moiety. The ORTEP drawing of 11a is shown in Fig. 1. The arrangement of ligands is trans. All type a complexes are centrosymmetric with the N4O2 donor set defining vertices of a distorted octahedron. Due to Jahn–Teller effect which is a common phenomenon for d9 ions,63 the octahedron is elongated. This bonding pattern is usually described as a “4 + 2” coordination.6 The two longer bonds are observed to the quinaldinate nitrogen atoms. The aromatic ligands deviate from strict planarity. Their non-planarity can be described by a dihedral angle between the carboxylate and the bicyclic system. The deviation is most pronounced for 11a with an angle of 10.3(2)°.
Table 1 Relevant geometric parameters [Å, °] for complexes with monodentate amino alcohol ligands
Compound |
Amino alcohol |
Cu–NH2 |
Cu–N(quin−) |
Cu–O(quin−) |
Non-planarity of quin− a |
Given by the dihedral angle between the carboxylate and the bicyclic system.
|
1a
|
2aeOH |
2.0014(15) |
2.3498(14) |
2.0813(12) |
4.6(3) |
6a
|
1a2m2pOH |
2.0193(14) |
2.3916(14) |
2.0445(12) |
5.3(3) |
7a
|
1a2bOH |
2.0033(15) |
2.4130(16) |
2.0355(12) |
2.5(3) |
11a
|
3a1pOH |
2.0184(14) |
2.4185(14) |
2.0034(11) |
10.3(2) |
11a·3a1pOH
|
3a1pOH |
2.0177(16), 2.0263(15) |
2.3627(15), 2.3869(15) |
2.0149(12), 2.0168(12) |
4.22(12), 6.3(3) |
Table 2 Relevant bond lengths [Å] for complexes with bidentate chelating amino alcohol ligands
Compound |
Amino alcohol |
Cu–amino group |
Cu–OH |
Cu–N(quin−) |
Cu–O(quin−) |
2b
|
2maeOH |
2.052(2) |
2.517(2) |
2.0353(13) |
1.9379(15) |
8b
|
2a1pOH |
2.0209(18) |
2.5059(16) |
2.3775(16), 2.0762(15) |
1.9381(13), 1.9524(14) |
11b
|
3a1pOH |
1.953(4) |
2.510(4) |
2.2408(13) |
1.9468(11) |
5e
|
1a2pOH |
1.998(2), 1.999(2), 2.057(2) |
2.0130(19), 2.3862(19), 2.4029(19) |
— |
— |
7e
|
1a2bOH |
1.9945(19), 1.9982(19), 2.035(2) |
2.0191(19), 2.3824(18), 2.4558(17) |
— |
— |
8e
|
2a1pOH |
1.900(8), 1.905(9), 1.994(3), 2.005(3), 2.036(12), 2.038(4), 2.082(11), 2.120(9), 2.133(7) |
1.995(12), 2.034(3), 2.069(11), 2.235(8), 2.297(8), 2.361(4), 2.385(6), 2.399(7), 2.418(4) |
— |
— |
10e
|
2a1bOH |
1.991(3), 1.997(3), 1.998(3), 2.000(3), 2.004(4), 2.004(3), 2.009(3), 2.010(3), 2.011(3), 2.019(3), 2.019(3), 2.020(3) |
1.995(3), 1.997(3), 2.005(3), 2.015(3), 2.331(3), 2.355(3), 2.381(3), 2.389(3), 2.462(3), 2.526(3), 2.550(3), 2.609(3) |
— |
— |
Table 3 Relevant bond lengths [Å] for complexes with bidentate bridging amino alcohol ligands
Compound |
Amino alcohol |
Cu–NH2 |
Cu–OH |
Cu–N(quin−) |
Cu–O(quin−) |
8c
|
2a1pOH |
2.056(5) |
2.288(4) |
2.2466(19) |
1.9535(14) |
11c
|
3a1pOH |
2.079(6) |
2.286(5) |
2.2447(17) |
1.9460(12) |
Table 4 Relevant geometric parameters [Å] for complexes with amino alcoholate ligands
Compound |
Amino alcohol |
Cu–amino group |
Cu–O− |
Cu–N(quin−) |
Cu–O(quin−) |
τ
65
|
syn-8d
|
2a1pOH |
2.001(3) |
1.941(3), 1.974(3) |
2.225(3) |
1.967(3) |
0.31 |
syn-11d
|
3a1pOH |
1.984(2), 2.004(2) |
1.9174(15)–1.9626(15) |
2.3088(17), 2.308(2) |
1.9728(15), 1.9695(16) |
0.13, 0.20 |
syn-11d·2H2O
|
3a1pOH |
1.981(2), 1.980(2) |
1.9275(16)–1.9432(17) |
2.310(2), 2.3170(19) |
2.0041(17), 2.0012(17) |
0.02, 0.13 |
anti-4d
|
2eaeOH |
2.039(3) |
1.929(2), 1.945(2) |
2.281(2) |
1.962(2) |
0.33 |
anti-11d·2MeCN
|
3a1pOH |
1.9946(14) |
1.9270(12), 1.9459(12) |
2.0472(14) |
2.2271(13) |
0.05 |
anti-11d·4MeOH
|
3a1pOH |
2.0007(13) |
1.9211(10), 1.9581(10) |
2.0189(12) |
2.2248(11) |
0.12 |
anti-11d·2MeOH·2(3a1pOH)
|
3a1pOH |
1.9921(13) |
1.9353(10), 1.9518(10) |
2.0422(12) |
2.2311(11) |
0.09 |
9f
|
2a2m1pOH |
1.9935(13) |
1.9162(10) |
— |
— |
— |
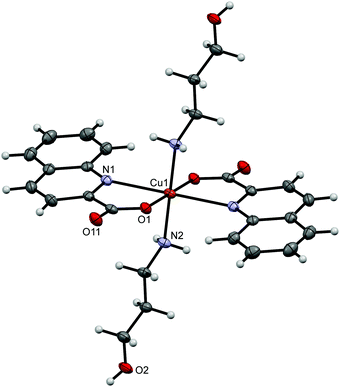 |
| Fig. 1 ORTEP drawing of trans-[Cu(quin)2(3a1pOH)2] (11a). Displacement ellipsoids are drawn at the 50% probability level. Hydrogen atoms are shown as spheres of arbitrary radii. | |
[Cu(quin)2(2maeOH)] (2b), [Cu(quin)2(2a1pOH)] (8b) and [Cu(quin)2(3a1pOH)] (11b) fall under type b compounds. In all, copper(II) ion is coordinated by two bidentate chelating quinaldinates and a bidentate chelating amino alcohol bound via both functional groups. Thus, the metal ion acquires the N3O3 coordination environment. The ORTEP drawing of [Cu(quin)2(2a1pOH)] (8b) is shown in Fig. 2. Complex molecules of 2b and 11b possess a crystallographically imposed C2 symmetry. Hence, their amino alcohol ligands exhibit disorder. In spite of the long bond between the metal ion and the OH moiety, 2.517(2) Å (2b), 2.5059(16) Å (8b), and 2.510(4) Å (11b), the most appropriate description of the amino alcohol binding mode remains a bidentate chelating one.
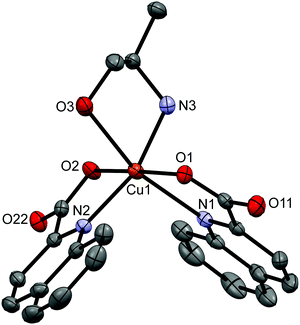 |
| Fig. 2 ORTEP drawing of [Cu(quin)2(2a1pOH)] (8b). Displacement ellipsoids are drawn at the 50% probability level. Hydrogen atoms are omitted for clarity. | |
[Cu(quin)2(2a1pOH)]n (8c) and [Cu(quin)2(3a1pOH)]n (11c) belong to type c complexes. The coordination environment of copper(II) consists of two bidentate chelating quinaldinates and two amino alcohol ligands, one being coordinated via NH2 and the other via OH moiety. The distribution of the N3O3 donors describes a distorted octahedron. The binding mode of the amino alcohol ligand is bidentate bridging, i.e., the amino moiety is bound to one metal ion and the hydroxyl moiety to a neighboring one. As a result, an infinite chain structure is formed. The ORTEP drawings of a repeating unit and a short section of a chain in [Cu(quin)2(2a1pOH)]n (8c) are shown in Fig. 3.
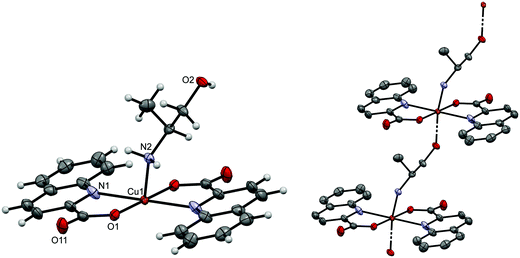 |
| Fig. 3 ORTEP drawings of a repeating unit (left) and a short section of a chain (right) in [Cu(quin)2(2a1pOH)]n (8c). The Cu(II) coordination sphere is completed by the O1, N1 and N2/O2 (1 − x, 1 − y, 1 − z) symmetry equivalents. Displacement ellipsoids are drawn at the 50% probability level. Hydrogen atoms are shown as spheres of arbitrary radii (left) or omitted for clarity (right). | |
Type d represents dinuclear complexes in which two copper(II) ions are bridged by two oxygen atoms, each belonging to a deprotonated amino alcohol. The resulting intermetallic distance is in the 2.9177(9)–3.0541(4) Å range. Both copper(II) ions share the same coordination environment which consists of a bidentate chelating quinaldinate and two amino alcoholate ions. As usually observed, the amino alcoholate engages in coordinating both donor groups:29 the amino group is bound to one metal ion, while the alkoxide oxygen is bound to two of them. In Harris notation, this binding mode is labelled 2.21.64 The metal ion is five-coordinated by N2O3 donors. With τ parameters in the 0.02 to 0.33 range, the geometry can be best described as a square pyramid.65 The dinuclear complexes exist in two isomeric forms which differ in the relative arrangement of quinaldinates with respect to the Cu2(μ-O)2 plane. In syn isomers, the quinaldinates are on the same side of this plane. Such an arrangement allows intramolecular π⋯π stacking (Table S8, ESI†). Complex molecules of syn-[Cu2(quin)2(3a1pO)2] (syn-11d) and syn-[Cu2(quin)2(3a1pO)2]·2H2O (syn-11d·2H2O) have no symmetry, while syn-[Cu2(quin)2(2a1pO)2] (syn-8d) belongs to the C2 point group symmetry. Interestingly, syn-8d is the only dinuclear complex that features a non-planar Cu2(μ-O)2 unit with the fold angle between its Cu(μ-O)2 planes being 20.64(8)°. Fig. 4 shows the ORTEP drawing of syn-11d. The relative distribution of the N2O3 donors in syn isomers is the same: the quinaldinate oxygen occupies an equatorial site and the quinaldinate nitrogen the apical one. On the other hand, anti isomers have their quinaldinates located on the opposite sides of the Cu2(μ-O)2 plane, i.e., one quinaldinate is above and the other one below this plane. With such an arrangement of the aromatic ligands, intramolecular π⋯π interactions are not possible. The complex molecules of anti-[Cu2(quin)2(2eaeO)2] (anti-4d), anti-[Cu2(quin)2(3a1pO)2]·2MeCN (anti-11d·2MeCN), anti-[Cu2(quin)2(3a1pO)2]·4MeOH (anti-11d·4MeOH) and anti-[Cu2(quin)2(3a1pO)2]·2MeOH·2(3a1pOH) (anti-11d·2MeOH·2(3a1pOH)) are centrosymmetric with a planar Cu2(μ-O)2 unit. Fig. 5 shows the ORTEP drawings of complex molecules in anti-4d and anti-11d·2MeCN. It is to be noted that the relative distribution of the N2O3 donors in anti-4d differs from the one in other anti compounds. In anti-4d, the quinaldinate oxygen occupies an equatorial site and the quinaldinate nitrogen the apical one, whereas all other compounds feature a reversed arrangement.
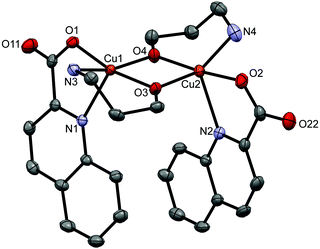 |
| Fig. 4 ORTEP drawing of syn-[Cu2(quin)2(3a1pO)2] (syn-11d). Displacement ellipsoids are drawn at the 50% probability level. Hydrogen atoms are omitted for clarity. | |
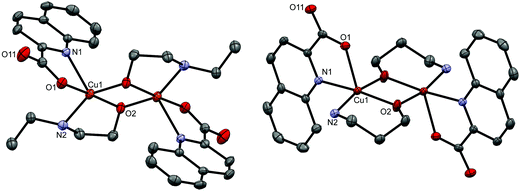 |
| Fig. 5 ORTEP drawing of anti-[Cu2(quin)2(2eaeO)2] (anti-4d) (left) and of anti-[Cu2(quin)2(3a1pO)2] in anti-11d·2MeCN (right). Displacement ellipsoids are drawn at the 50% probability level. Hydrogen atoms are omitted for clarity. | |
The ionic compounds, [Cu(1a2pOH)3](quin)2 (5e), [Cu(1a2bOH)3](quin)2 (7e), [Cu(2a1pOH)3](quin)2 (8e) and [Cu(2a1bOH)3](quin)2 (10e), feature a positively charged copper(II) complex with three amino alcohol ligands coordinated in a bidentate chelating manner and two quinaldinate ions as counter anions. The ORTEP drawing of the [Cu(1a2bOH)3]2+ ion in 7e is shown in Fig. 6. The N3O3 donor set occupies vertices of a distorted octahedron in a mer distribution. The bonding pattern matches the “4 + 2” description with the two longer bonds formed between copper(II) and the hydroxyl oxygen.
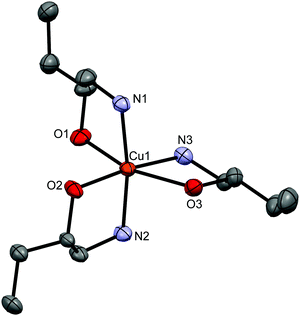 |
| Fig. 6 ORTEP drawing of [Cu(1a2bOH)3]2+ in 7e. Displacement ellipsoids are drawn at the 50% probability level. Hydrogen atoms are omitted for clarity. | |
A co-crystal [Cu(2a2m1pO)2](2a2m1pOHH)2(quin)2 (9f) consists of a neutral complex [Cu(2a2m1pO)2] with two amino alcoholate ligands coordinated in a bidentate chelating manner (Fig. 7), NH2-protonated amino alcohol species and quinaldinate anions in the 1
:
2
:
2 ratio. The four-coordinate [Cu(2a2m1pO)2] is centrosymmetric with the N2O2 donors in a square-planar arrangement. A set of the closest neighbors of copper(II) ion consists apart from the N2O2 donors also of two hydroxyl groups of the 2a2m1pOHH+ cations at a distance of 3.5197(12) Å.
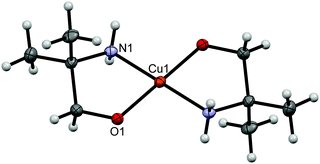 |
| Fig. 7 ORTEP drawing of [Cu(2a2m1pO)2] in 9f. Displacement ellipsoids are drawn at the 50% probability level. Hydrogen atoms are shown as spheres of arbitrary radii. | |
[Cu(quin)2]n (g) is a one-dimensional coordination polymer which may be regarded as an infinite repetition of mononuclear [Cu(quin)2] units. These mononuclear units are linked with the agency of long Cu⋯O bonds, 2.5772(12) Å, with oxygen atom belonging to the quinaldinate of the adjacent unit. Fig. 8 shows the ORTEP drawing of a short section of a chain. In this chain, each copper(II) ion is coordinated by four quinaldinates: two are bound in a N,O-chelating manner and two are bound via one carboxylate oxygen atom. The N2O4 donors surround the metal ion in the “4 + 2” bonding pattern. The quinaldinate ligand has employed in coordination all three donor atoms to two metal ions and thereby serves as a bridging ligand. In Harris notation, this binding mode is labelled 2.111.64 The quinaldinates substantially deviate from planarity as the angle between the carboxylate moiety and the bicyclic system amounts to 19.22(14)°. Interestingly, a π⋯π stacking interaction occurs within the chains between adjacent quinaldinates. Two C–H⋯O interactions with a length of 2.9530(18) Å may be observed between the quinaldinates of the same [Cu(quin)2] unit.
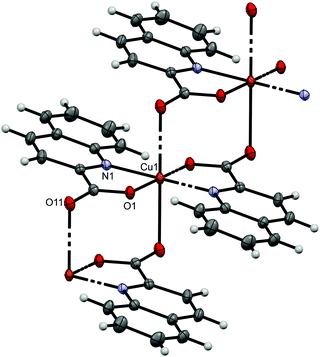 |
| Fig. 8 ORTEP drawing of a short section of a chain in [Cu(quin)2]n (g). Displacement ellipsoids are drawn at the 50% probability level. Hydrogen atoms are shown as spheres of arbitrary radii. The Cu(II) coordination sphere is completed by the O11 (−x, 1 − y, 1 − z and 1 + x, y, z), N1 and O1 (1 − x, 1 − y, 1 − z) symmetry equivalents. The Cu–N bond lengths are 2.0907(12) Å, and the Cu–O bond lengths are 1.9089(10) and 2.5772(12) Å. | |
The novel copper(II) complexes possess two types of amino alcohol ligands: neutral molecules and amino alcoholate anions that form upon the deprotonation of OH group. Amino alcohols invariably coordinate through their amino group either as monodentate ligands or as bidentate chelating or bidentate bridging ligands. In the latter two cases, the OH group also participates in coordination. In general, the copper(II)-to-amine bonds span a narrow interval, 1.900(8)–2.133(7) Å. Conversely, large variations may be observed among the Cu–OH bonds: they can be as short as 1.995(3) Å (10e) or as long as 2.609(3) Å (10e). Among the known copper(II) amino alcohol complexes, a bidentate chelating coordination prevails, whereas a monodentate one through the amino group occurs only rarely.29 To the best of our knowledge, there is no example of a bidentate bridging coordination that was encountered in type c complexes. As a rule, the amino alcoholate ligands employ in coordination both donor groups. Compound 9f revealed a bidentate chelating fashion, whereas dinuclear compounds a tridentate bridging one. A survey of the known copper(II) amino alcoholate complexes shows that the coordination with the alkoxide oxygen acting as a bridge between two or three metal ions is the prevailing one.29 The bonding pattern of the amino alcoholate ligands in our compounds is uniform: the copper(II)-to-amine bonds are in the 1.980(2)–2.039(3) Å range and the copper(II)-to-alkoxide bonds are 1.9162(10)–1.974(3) Å.
3.3. Intermolecular interactions
As anticipated, the connectivity in the solid state is governed by hydrogen bonds and π⋯π stacking interactions. The supramolecular structures are described only briefly and more details are provided in the ESI.† Type a compounds 1a, 6a and 7a share a similar connectivity in the solid state. In 1a, complex molecules are hydrogen bonded via OH⋯COO− and NH2⋯COO− interactions into infinite supramolecular chains. The shortest Cu⋯Cu contacts in 1a are 5.9728(2) Å. These contacts occur within the same chain. The chains pack in a parallel fashion with no significant π⋯π stacking interactions between quinaldinate ligands.66 The intermetallic distances in 6a and 7a are very similar. In 11a, the OH⋯COO− hydrogen bonds link molecules into chains with the shortest Cu⋯Cu contacts within the chains exceeding 11 Å. Due to the presence of 3-amino-1-propanol molecules in 11a·3a1pOH, its solid-state structure differs. An intricate pattern of hydrogen bonding involving both the complex and solvent molecules of crystallization produces a three-dimensional supramolecular network. Interestingly, as in other type a compounds, there are no π⋯π stacking interactions between quinaldinate ligands. The chelates 2b, 8b and 11b share the same connectivity pattern: the OH⋯COO− and NH2⋯COO− synthons link molecules into chains with π⋯π stacking occurring between adjacent chains. The structures of 1D-polymers 8c and 11c are identical: the chains are hydrogen bonded into layers with π⋯π stacking occurring between adjacent layers.
The syn disposition of aromatic ligands in syn-[Cu2(quin)2(3a1pO)2] (syn-11d) allows intramolecular π⋯π stacking. Only weak hydrogen bonds may be observed between dinuclear complex molecules. These bonds link molecules into supramolecular chains. The presence of water molecules of crystallization in syn-11d·2H2O entails changes in the connectivity. Hydrogen bonds between water and complex molecules produce infinite layers. A comparison with the complex molecule of syn-11d reveals that in syn-11d·2H2O the quinaldinate ligands within the same complex molecule are slightly pulled apart. Furthermore, intermolecular π⋯π stacking may be observed. In syn-[Cu2(quin)2(2a1pO)2] (syn-8d), hydrogen bonds between NH2 and COO− entities link complex molecules into double chains. Both types of π⋯π stackings may be observed, intra- and intermolecular ones, the latter occurring within the same chain. Interactions for anti-[Cu2(quin)2(3a1pO)2] compounds are different since intramolecular π⋯π stacking is not possible. In the acetonitrile solvate, anti-11d·2MeCN, the NH2⋯COO− synthons link complex molecules into infinite layers. In addition, the complex molecules are held together with intermolecular π⋯π stacking within the same layer. Acetonitrile solvent molecules are packed in-between layers. The presence of the solvent, capable of forming hydrogen bonds, changes the connectivity pattern. Both anti-11d·4MeOH and anti-11d·2MeOH·2(3a1pOH) contain such solvents. In the methanol solvate, anti-[Cu2(quin)2(3a1pO)2] molecules and methanol molecules are linked into a three-dimensional supramolecular network in which the layers of complex molecules alternate with the layers of solvent molecules. In anti-11d·2MeOH·2(3a1pOH) with two solvents of crystallization, the dinuclear complex molecules are hydrogen bonded into layers. Within the layer, intermolecular π⋯π stacking interactions may be observed. The surface of layers is decorated with methanol and 3-amino-1-propanol molecules. Again, the resulting three-dimensional connectivity pattern may be viewed as an alternation of layers of complex molecules and layers of solvent molecules. The absence of a solvent in anti-[Cu2(quin)2(2eaeO)2] (anti-4d) makes the compound stable and results in a different connectivity pattern: the complex molecules are linked into chains via the NH2⋯COO− hydrogen bonds. The chains pack in a parallel fashion with π⋯π stacking interactions between adjacent chains.
DFT calculations show the isolated syn isomer of [Cu2(quin)2(3a1pO)2] to be the most stable by 7.2 kcal mol−1 (ΔZPE). This can be almost entirely pinpointed to the intramolecular π⋯π stacking as confirmed by the analysis of non-covalent interactions (NCI, Fig. 9). This analysis shows NCIs as isosurfaces in which the electron density (ρ), its reduced gradient and the second largest eigenvalue of Hessian matrix of the electron density (λ2) are close to zero.61 On the other hand, the calculations in the absence of empirical dispersion yield both isomers isoenergetic.
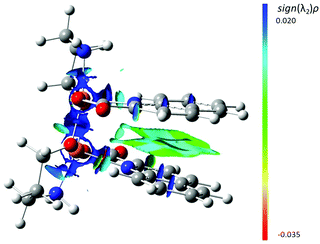 |
| Fig. 9 The NCI plot showing intramolecular π⋯π interactions in syn-[Cu2(quin)2(3a1pO)2] with blue and red regions representing repulsive and attractive weak interactions, respectively. Intermediate, weak non-covalent interactions are shown in green. | |
Type e compounds share the same connectivity in the solid state. For example, in 5e, the [Cu(1a2pOH)3]2+ complex cations and the quinaldinate counterions are hydrogen bonded into supramolecular chains with π⋯π stacking interactions between adjacent chains. The solid-state structure of 9f consists of the [Cu(2a2m1pO)2] complex molecules, 2a2m1pOHH+ cations and quin− anions that are hydrogen bonded into layers with π⋯π stacking interactions between the layers. In the structure of g, infinite chains pack in a parallel fashion: each chain is surrounded by six others. π⋯π stacking interactions occur within the same chain. No classical hydrogen bonds may be observed.
3.4. Relative stability of the 3a1pOH monomers
As the 3-amino-1-propanol ligand produces the richest system in terms of the isolated species, two mononuclear complexes, trans-[Cu(quin)2(3a1pOH)2] (11a) and [Cu(quin)2(3a1pOH)] (11b), were optimized by DFT methods in bulk acetonitrile in order to assess their relative stabilities. The choice of 11a was governed by its reproducible formation under very diverse conditions. On the other hand, the difficulties experienced during the isolation of the two mono amino alcohol compounds, 11b and 11c, implied their nature to be very labile. The calculations indeed show 11a to be the most stable mononuclear species in this system. Furthermore, they indicate that the formal extrusion of one 3ap1OH ligand from trans-[Cu(quin)2(3a1pOH)2] (11a) to yield [Cu(quin)2(3a1pOH)] (11b) with the remaining amino alcohol bound in a bidentate chelating manner is slightly exergonic (by ca. 1 kcal mol−1; see Table S15 for details, ESI†). The process can be considered to occur through a five-coordinate species, labelled 11a–L. The latter was derived from a repeating unit of the 1D-polymer 11c.
trans-[Cu(quin)2(3a1pOH)2] (11a) → [Cu(quin)2(3a1pOH)] (11a–L) + 3a1pOH |
The calculations show 11a–L and 3ap1OH to be almost isoenergetic with 11a. The results on the relative stabilities of 11a, 11a–L and 11b merit further comment since the calculated free energy differences point towards exclusive formation of 11a–L in the solution. The free energy variations were calculated at 1 atm for all species and intermolecular interactions in solution were not considered. However, the entropy term more than compensates for the energy required for the dissociation of one 3a1pOH ligand from 11a. Nevertheless, the energy variations calculated for the interconversion of the above species can be regarded as small enough and as such consistent with their facile interconversion in solution.
As for the greater calculated stability of 11a–Lvs.11b, it must be noted that both species are almost isoenergetic when zero-point energies are compared. However, there is an entropy penalty related to the formation of the chelate 11b, which is counterbalanced by the energy released in the formation of a Cu–OH bond. This bond is significantly longer (by ca. 0.5 Å) than the other two Cu–O bonds, and the same is true for the Cu–N bond trans to it. The elongation of both bonds is attributable to the Jahn–Teller distortion. Accordingly, the Atom in Molecules (AIM) analysis58 of the calculated electron density reveals electron densities at the bond critical points of these elongated bonds that are ca. 0.03 a.u., compared to the electron densities at the bond critical points for the remaining coordination bonds, which are in the range of 0.06–0.08 a.u. The calculated structures of 11b and 11a–L feature marked deviations from their respective ideal octahedral or square pyramid coordination environments (and from the initial X-ray geometries) caused by intramolecular π⋯π stacking of quinaldinates (Fig. 10). In the crystal lattice of 11b, intermolecular π⋯π stacking may be observed instead.
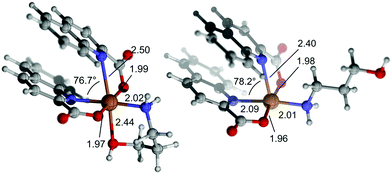 |
| Fig. 10 DFT-optimized geometries of 11b (left) and 11a–L (right). | |
3.5. Magnetic properties
The magnetic properties of a series of copper(II) complexes were studied by dc susceptibility measurements between 300 and 1.85 K. The mononuclear compounds, which were measured are [Cu(quin)2(2aeOH)2] (1a), [Cu(quin)2(1a2m2pOH)2] (6a), [Cu(quin)2(1a2bOH)2] (7a) [Cu(quin)2(3a1pOH)2] (11a), [Cu(quin)2(2maeOH)] (2b), [Cu(1a2pOH)3](quin)2 (5e), [Cu(1a2bOH)3](quin)2 (7e), [Cu(2a1pOH)3](quin)2 (8e) and [Cu(2a2m1pO)2](2a2m1pOHH)2(quin)2 (9f). Their room temperature χT product values fall within the 0.38 to 0.43 cm3 K mol−1 interval and are consistent with the presence of one S = 1/2 Cu(II) center with g values ranging from 2.03(5) to 2.15(5). Furthermore, the series of a, b, e and f compounds displays a very similar temperature dependence of χT products (Fig. 11, top part). On lowering the temperature, the χT value remains constant at first, whereas at low temperatures, it decreases in a more or less pronounced manner. Such a behavior is an indication of weak or very weak intermolecular antiferromagnetic (AF) interactions. The solid-state structures of type a compounds are characterized by a hydrogen bonded one-dimensional arrangement with short Cu–O⋯HN–Cu contacts (Fig. S4, S5 and Table S5, ESI†). Since the strongest magnetic interaction was expected to be mediated through these contacts, the magnetic susceptibility was modeled using the regular antiferromagnetic quantum S = 1/2 spin chain model with the following spin Hamiltonian,
and the analytical susceptibility of Bonner–Fisher.67–69 As shown in Fig. 11, the magnetic data for 1a, 6a, 7a and 11a are well-reproduced through the whole temperature range with this approach, leading to J/kB = −2.6(1) K and g = 2.10(5) for 1a, J/kB = −1.4(1) K and g = 2.15(5) for 6a, J/kB = −2.8(1) K and g = 2.15(5) for 7a, and J/kB = −1.75(4) K and g = 2.13(5) for 11a.
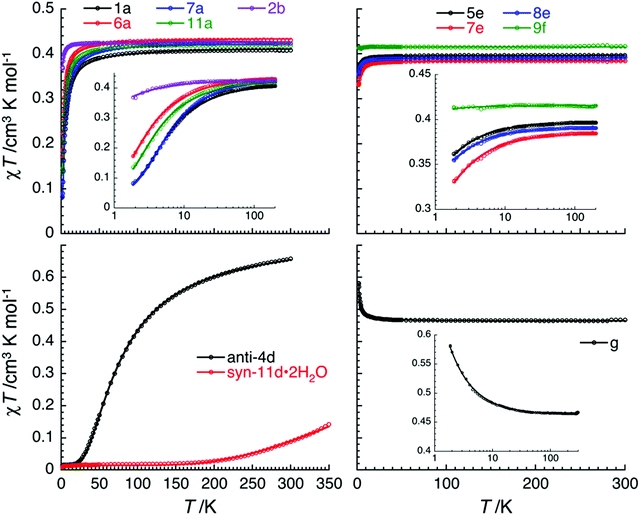 |
| Fig. 11 The temperature dependence of the χT product (where χ is the molar magnetic susceptibility that equals M/H per compound, and T is the temperature), collected at a dc magnetic field of 0.1 T for 1a, 6a, 7a, 11a and 2b (top left), 5e, 7e, 8e and 9f (top right), anti-4d and syn-11d·2H2O (bottom left), and g (bottom right). Insets: Semi-logarithm χT vs. T plots emphasizing the magnetic data at low temperatures. The solid lines are the best fits to the models described in the text. | |
In 2b, 5e, 7e, 8e and 9f, the mononuclear compounds of types b, e and f, the observed supramolecular contacts and possible magnetic pathways are much weaker and involve more than three atoms between spin carriers. Similarly to type a compounds, a supramolecular chain organization is observed for 2b, 5e, 7e and 8e (Fig. S7 and S16, ESI†). The shortest Cu⋯Cu contacts in 2b are 7.0950(2) Å and the ones in type e compounds are in the 6.5514(6)–6.8633(7) Å range. The magnetic susceptibility for 2b, 5e, 7e and 8e was modeled by applying the regular antiferromagnetic quantum S = 1/2 spin chain model67–69 which reproduces very well the experimental data (Fig. 11, top part). The corresponding magnetic parameters are J/kB = −0.21(5) K and g = 2.13(5) for 2b, J/kB = −0.14(3) K and g = 2.06(5) for 5e, J/kB = −0.24(5) K and g = 2.03(5) for 7e, and J/kB = −0.14(2) K and g = 2.04(5) for 8e. The magnetic interactions in these compounds are about 10 times smaller than in type a compounds. In the case of 9f, owing to the co-crystallization of 2a2m1pOHH+ and quin− ions, the mononuclear [Cu(2a2m1pO)2] complex molecules are well-separated, as shown by the shortest Cu⋯Cu contacts which exceed 8.1133(4) Å. Therefore, it is difficult to imagine any significant magnetic interactions. Magnetic interactions of −0.01(1) K were thus estimated from the mean field theory (with 4 next-neighbours) and the Weiss constant of –0.02 K, obtained from the Curie–Weiss fit of the experimental data. It should be underlined that the efficient separation of the [Cu(2a2m1pO)2] complex molecules in 9f leads to virtually magnetically isolated S = 1/2 Cu(II) spins.
The magnetic properties of the dinuclear Cu(II) complexes, anti-[Cu2(quin)2(2eaeO)2] (anti-4d) and syn-[Cu2(quin)2(3a1pO)2]·2H2O (syn-11d·2H2O), were also studied in the 1.85–300 K temperature range (Fig. 11). In both, a pair of Cu(II) sites is bridged by two alkoxide oxygens. It has been well-documented in the literature70–74 that in this type of complexes the nature and magnitude of the magnetic interaction between the S = 1/2 Cu spins depend strongly on subtle differences in the geometry of the Cu(μ-OR)2Cu moiety. The Cu–(μ-O)–Cu angle (θ) was identified as the primary factor: for angles smaller than 95.7°, ferromagnetic couplings are expected, while antiferromagnetic ones are observed for larger angles.70–74 Other geometric factors, such as the planarity of the Cu2(μ-O)2 moiety and the out-of-plane shift of the carbon atom of the bridging alkoxide, known as τ, usually add minor corrections to the main effect of the θ angle. It was also shown that small values of θ are associated with larger values of τ. In syn-11d·2H2O with θ being 103.25(8) and 103.85(8)° and τ being 3.85(17) and 3.99(16)°, a strong AF interaction is expected, while a weaker one is likely for anti-4d with θ = 100.09(9)° and τ = 38.0(2)°. The room temperature χT value, 0.089 cm3 K mol−1 for syn-11d·2H2O and 0.66 cm3 K mol−1 for anti-4d, is lower than the one expected for two magnetically isolated S = 1/2 spins (0.75 cm3 K mol−1), confirming the presence of dominant AF interactions. On lowering the temperature, the χT product decreases. Below 20 K, it vanishes to nearly zero for both compounds, indicating that strong antiferromagnetic interactions induce a singlet ground state, ST = 0. To evaluate the intradimer magnetic interaction, the magnetic data were fitted to a simple S = 1/2 spin dimer model with the following Hamiltonian,
and the analytical susceptibility expression of Bleaney and Bowers.75 An excellent agreement between the experimental data and the model is observed with J/kB = −550(10) K and g = 2.1 (fixed) for syn-11d·2H2O, and J/kB = −69.5(5) K and g = 2.01(5) for anti-4d. It is worth mentioning that as usually done for systems with a singlet ground state, Curie impurities have been included in this model allowing the estimation of their presence at 3.7 and 3.8% of a S = 1/2 spin.
Singlet and triplet states of syn-[Cu2(quin)2(3a1pO)2] were calculated by optimizing the X-ray structure without restrictions in bulk acetonitrile. According to −2J = E(S = 1) − E(ST = 0),72 the sign and the magnitude of the magnetic coupling constant can be estimated as −163.1 K. The calculations predict a singlet ground state also for an anti isomer, although no direct bonding was located between the copper(II) ions by AIM analysis in either case (Fig. 12).58 The calculated value of the magnetic coupling constant for isolated syn-[Cu2(quin)2(3a1pO)2] differs from the experimental one for bulk syn-11d·2H2O. For the optimized syn-[Cu2(quin)2(3a1pO)2], θ amounts to 97.8° and τ to 43°. In line with the previous discussion, a smaller value of θ is, as expected, associated with a larger τ and a weaker AF interaction.
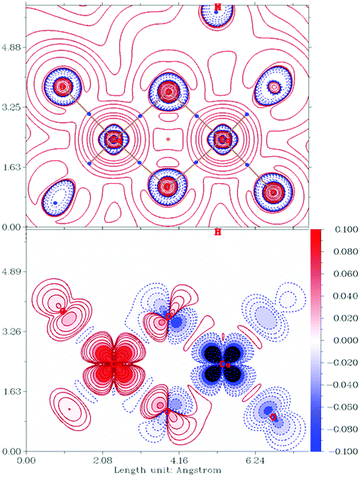 |
| Fig. 12 Bond critical points (bcps) and corresponding bond paths of the calculated electron density of the singlet syn-[Cu2(quin)2(3a1pO)2] in the Cu2(μ-O)2 plane. The contour map of the Laplacian of the electron density (∇2ρ) is also plotted (above); the electron spin-density plot for the singlet ground state of the same species (below). Solid lines and red tones correspond to positive values whereas dashed lines and blue tones are for negative values. | |
The magnetic susceptibility of [Cu(quin)2]n (g) was also studied. This compound features an infinite chain structure with a 4.8617(2) Å distance between adjacent copper(II) ions, bridged by two anti(eq)-syn(ax)-carboxylate groups. On lowering the temperature down to 60 K, the χT product is quasi-constant with the 0.46 cm3 K mol−1 value being in good agreement with the presence of a S = 1/2 spin. At lower temperatures, χT slightly increases (up to 0.58 cm3 K mol−1 at 1.85 K and 0.1 T) suggesting dominant, but very weak, ferromagnetic interactions between spin carriers. A Heisenberg chain model of ferromagnetically coupled S = 1/2 spins with the following spin Hamiltonian,
, was employed to fit the experimental data. Using the analytical expression of susceptibility proposed by Baker et al.,76 the experimental data are remarkably well reproduced down to 1.85 K with J/kB = +0.04(1) K and g = 2.22(5). As expected, the double carboxylate bridges mediate weak ferromagnetic interactions between S = 1/2 Cu(II) spins.77
3.6. Infrared spectroscopy
The presence of quinaldinate and amino alcohol ligands is clearly evident from infrared spectra. Owing to the overlap of some of their characteristic absorption bands and the engagement of NH2 and OH groups in hydrogen bonds, not all relevant features of the two ligand types can be unambiguously assigned. It may be noted that compounds with the same solid-state structure have very similar infrared spectra. The identification of the quinaldinate absorptions was facilitated by the spectrum of [Cu(quin)2]n (g), a homoleptic complex. Frequencies of the νas(COO−) and νs(COO−) vibrational modes, listed in Table S18 (ESI†), are of particular importance as they are sensitive to the carboxylate binding mode.78 The difference in their positions is known as the Δ value. Magnitudes of Δ for all our compounds with monodentate carboxylates are in line with the expectations.78 However, the Δ criterion fails for [Cu(quin)2]n (g) as the frequency difference amounts to 272 cm−1. Its magnitude is larger than expected for a bidentate bridging carboxylate coordination.78 In the case of [Cu(2a2m1pO)2](2a2m1pOHH)2(quin)2 (9f), only non-coordinated quinaldinate ions are present. Their role of counter anions is evident from the spectrum: the νas(COO−) vibration is associated with the band at 1553 cm−1 and that of the νs(COO−) vibration with the band at 1372 cm−1. As a result, the value of Δ amounts to 181 cm−1. The ionic type e compounds exhibit carboxylate bands at almost the same positions as 9f. It should be noted that a set of four peaks with medium intensity, i.e., at 1567, 1510, 1463 and 1436 cm−1 for g, invariably appears in the spectra of all quinaldinate complexes at approximately the same frequencies.
A close survey of the infrared data of our copper(II) compounds shows that no information about the binding mode of the amino alcohol ligand can be extracted from their spectra. The ν(N–H) and ν(O–H) vibrations can be told apart only in rare cases. Due to extensive participation of NH2 and OH groups in hydrogen bonding, N–H and O–H stretches often appear as broad bands.79 The expected region for the NH2 deformation band in primary amines, 1650–1590 cm−1,79 overlaps with the most intense quinaldinate signature band, the νas(COO−) band. Therefore, these bands could not be unambiguously assigned. The presence of alcohol in the compound may be seen in another spectral region: the region of C–O stretching absorptions. The ν(C–O) absorptions are seen as strong bands with their frequencies sensitive to the nature of the alcohol.79 The alcohols in the bonded state are reported to absorb very broadly and diffusely near 650 cm−1 owing to the out-of-plane C–OH deformation.79 Our compounds with amino alcohols also reveal a band of medium intensity in this region (Table S19, ESI†).
The spectra of type a complexes are the least uniform: the number and the frequencies of the bands in the ν(N–H)/ν(O–H) region differ for all. The ν(C–O) spectral region reveals intense bands: 1a with a primary alcohol 2aeOH features a band at 1034 cm−1, 7a with a secondary alcohol 1a2bOH absorbs at 1137–961 cm−1 and 6a with a tertiary alcohol 1a2m2pOH displays several bands in the 1178–958 cm−1 region. One of the common spectral features for 1a, 6a, 7a and 11a is four absorptions in the region of νas(COO−) and N–H bending vibrational mode, as exemplified by 1604, 1587, 1562 and 1550 cm−1 bands for 1a. The high-frequency band at ca. 1604 cm−1 was tentatively assigned to the νas(COO−) vibration and the rest to the N–H bending vibrations. The trans-[Cu(quin)2] structural fragment leaves its fingerprint in the shape and frequencies of four bands in the out-of-plane C–H region. It is to be noted that the crystallization solvent molecules and a different solid-state structure of 11a·3a1pOH make its infrared spectrum markedly different from that of its unsolvated counterpart 11a.
The infrared spectra of type b compounds are very much alike. For the 8b and 11b pair, there are four bands in the ν(N–H)/ν(O–H) region. Owing to the secondary nature of the amino alcohol in 2b, the number of peaks in this region is smaller. The spectra of all three reveal two prominent absorptions in the νas(COO−)/N–H bending region: for example, the spectrum of 11b reveals an intense band at 1641 cm−1 and a band at 1594 cm−1. The same absorption pattern may be observed for the spectra of 8c and 11c. Again, their similarity can be traced to the similarity of their solid-state structures.
The spectra of the dinuclear complexes should be free of ν(O–H) absorptions in keeping with the formulation as an amino alcoholate rather than an alcohol complex. This expectation is realized for compounds that do not contain solvent molecules of crystallization, i.e., methanol, 3-amino-1-propanol or water. The spectrum of anti-[Cu2(quin)2(2eaeO)2] (anti-4d) differs from the spectra of the 3a1pO−anti dimers, anti-11d·4MeOH, anti-11d·2MeCN and anti-11d·2MeOH·2(3a1pOH). In the spectrum of anti-4d, the ν(N–H) absorption occurs as a single sharp band at 3183 cm−1. Broad bands, belonging to solvent molecules of 3-amino-1-propanol and/or methanol for anti-11d·2MeOH·2(3a1pOH) and anti-11d·4MeOH, mask the ν(N–H) bands of the coordinated amino alcoholate. The presence of solvent molecules is apparent also from the characteristic region of the C–O stretching vibrations: for anti-11d·4MeOH, a very intense band may be observed at 1022 cm−1, whereas for anti-11d·2MeOH·2(3a1pOH) a band occurs at 1051 cm−1. Their intensities diminish with time, in line with the rapid loss of solvent molecules. The presence of the acetonitrile solvent molecules in anti-11d·2MeCN is ascertained by the sharp band at 2249 cm−1.79 The spectra of syn-8d, syn-9d and syn-10d are very much alike. It is to be noted that unambiguous identification by means of X-ray crystallography was accomplished only for syn-8d. Based on the similarity of their spectra, it can be claimed with a high probability that their solid-state structures are very similar. All three reveal two sharp bands that can be attributed to ν(N–H) absorptions. For syn-8d, the peaks occur at 3251 and 3145 cm−1. syn-11d·2H2O also features two bands at almost the same frequencies. The water solvate has an additional broad band at 3469 cm−1 whose origin lies in the ν(O–H) vibrations of solvent water molecules.
The spectral features of type e ionic compounds with chelating amino alcohol ligands are very similar. They display two bands in the ν(N–H)/ν(O–H) region, at 3236 and 3122 cm−1 (5e). Surprisingly, in spite of the same binding mode employed by the amino alcohol ligands, the spectra are markedly different from the spectra of type b complexes. The spectrum of [Cu(2a2m1pO)2](2a2m1pOHH)2(quin)2 (9f), a compound which contains both the amino alcoholate ligand and the cationic form of the parent amino alcohol, reveals the ν(N–H) vibration of the NH2 group as a sharp absorption of medium intensity at 3315 cm−1. A broad absorption that spans the 3200–2500 cm−1 region is attributed to the ν(N–H) and ν(O–H) absorptions of the hydrogen bonded NH3+ and OH entities. The region of the N–H bending vibrations reveals three bands, at 1644, 1602 and 1582 cm−1. The ν(C–O) vibrations may be seen as strong bands at 1055 and 1010 cm−1.
4. Conclusions
The Cu(II)–quinaldinate–amino alcohol reaction systems display great structural diversity in their products which may be classified into seven distinctly different structural types. The series encompasses mononuclear, dinuclear and 1D-polymeric species with either intact amino alcohols or amino alcoholate ions serving as ligands. No correlation between the nature of the product and the applied reaction conditions could be discerned. To the best of our knowledge, copper(II) complexes with three out of eleven amino alcohols, 2-methylaminoethanol, 1-amino-2-methyl-2-propanol and 1-amino-2-butanol, have not been prepared prior to this study. The richest system in terms of products was that of 3-amino-1-propanol: it has yielded nine compounds. Two mononuclear 3-amino-1-propanol complexes, trans-[Cu(quin)2(3a1pOH)2] and [Cu(quin)2(3a1pOH)] with a chelating amino alcohol, were subjected to DFT calculations which show their facile interconversion in the solution to proceed via an intermediate five-coordinate species. The dinuclear amino alcoholate complex, [Cu2(quin)2(3a1pO)2], displayed syn- and anti-isomerism. DFT calculations on the isolated isomers revealed the syn isomer as the most stable one with its stability originating from an intramolecular π⋯π stacking interaction between quinaldinates. Furthermore, calculations show that both isomers display antiferromagnetic interactions between S = 1/2 Cu(II) spins. These results qualitatively agree with the experimental value for syn-[Cu2(quin)2(3a1pO)2]·2H2O. In line with previous studies, the nature and strength of the magnetic exchange depends upon the geometry of the Cu(μ-O)2Cu core. The mononuclear complexes trans-[Cu(quin)2L2] (where L denotes amino alcohol) and [Cu(quin)2L] with a bidentate chelating amino alcohol display very weak to weak antiferromagnetic coupling through supramolecular contacts in their solid-state structures. By analogy, [Cu(2a2m1pO)2](2a2m1pOHH)2(quin)2 with an efficient separation of the S = 1/2 Cu(II) spins by the counter ions does not display significant magnetic interactions. [Cu(quin)2]n, the homoleptic quinaldinate complex with an infinite chain structure, is the only one in the series that features weak but nevertheless observable, ferromagnetic behavior.
Author contributions
Conceptualization: B. M. and N. P.; investigation and formal analysis: all authors; writing – review & editing: B. M., N. P., R. C. and J. L. S.
Funding
Research was funded by the Slovenian Research Agency (Junior Researcher Grant for N. P. and the Program Grant P1-0134), MICINN (grant PID2019-110856GA-I00), the CNRS, the University of Bordeaux, the Région Nouvelle Aquitaine and Quantum Matter Bordeaux.
Conflicts of interest
There are no conflicts to declare.
Acknowledgements
M. M. A. thanks the MICINN for a research fellowship. The Supercomputing Centre of Galicia (CESGA) and the Centro de Servicios de Informática y Redes de Comunicaciones (CSIRC), Universidad de Granada are acknowledged for providing the computing time.
References
- M. O’Keeffe, M. A. Peskov, S. J. Ramsden and O. M. Yaghi, Acc. Chem. Res., 2008, 41, 1782–1789 CrossRef PubMed.
- M. O’Keeffe and O. M. Yaghi, Chem. Rev., 2012, 112, 675–702 CrossRef PubMed.
- E. V. Alexandrov, V. A. Blatov, A. V. Kochetkov and D. M. Proserpio, CrystEngComm, 2011, 13, 3947–3958 RSC.
-
G. R. Desiraju, J. J. Vittal and A. Ramanan, Crystal Engineering: A Textbook, World Scientific Publishing Co., Singapore, 2011 Search PubMed.
- G. R. Desiraju, Angew. Chem., Int. Ed., 2007, 46, 8342–8356 CrossRef CAS PubMed.
-
N. N. Greenwood and A. Earnshaw, Chemistry of the Elements, Butterworth-Heinemann, Oxford, UK, 2nd edn, 1997 Search PubMed.
- K. S. Egorova and V. P. Ananikov, Organometallics, 2017, 36, 4071–4090 CrossRef CAS.
-
J. J. R. Fraústo da Silva and R. J. P. Williams, The biological chemistry of the elements: The inorganic chemistry of life, Oxford University Press, Oxford, UK, 2nd edn, 2001 Search PubMed.
- R. A. Festa and D. J. Thiele, Curr. Biol., 2011, 21, R877–R883 CrossRef CAS PubMed.
- V. Tudor, T. Mocanu, F. Tuna, A. M. Madalan, C. Maxim, S. Shova and M. Andruh, J. Mol. Struct., 2013, 1046, 164–170 CrossRef CAS.
- J. W. Sharples and D. Collison, Coord. Chem. Rev., 2014, 260, 1–20 CrossRef CAS PubMed.
- P. Seppälä, E. Colacio, A. J. Mota and R. Sillanpää, Dalton Trans., 2012, 41, 2648–2658 RSC.
- S. S. P. Dias, V. André, J. Kłak, M. T. Duarte and A. M. Kirillov, Cryst. Growth Des., 2014, 14, 3398–3407 CrossRef CAS.
- P. Seppälä, E. Colacio, A. J. Mota and R. Sillanpää, Inorg. Chim. Acta, 2010, 363, 755–762 CrossRef.
- R. Sillanpää, T. Lindgren and L. Hiltunen, Inorg. Chim. Acta, 1987, 131, 85–88 CrossRef.
- L. J. Farrugia, D. S. Middlemiss, R. Sillanpää and P. Seppälä, J. Phys. Chem. A, 2008, 112, 9050–9067 CrossRef CAS PubMed.
- G. Marin, V. Kravtsov, Y. A. Simonov, V. Tudor, J. Lipkowski and M. Andruh, J. Mol. Struct., 2006, 796, 123–128 CrossRef CAS.
- C. Paraschiv, M. Andruh, S. Ferlay, M. W. Hosseini, N. Kyritsakas, J.-M. Planeix and N. Stanica, Dalton Trans., 2005, 1195–1202 RSC.
- G. Marin, M. Andruh, A. M. Madalan, A. J. Blake, C. Wilson, N. R. Champness and M. Schröder, Cryst. Growth Des., 2008, 8, 964–975 CrossRef CAS.
- F. Sama, A. K. Dhara, M. N. Akhtar, Y.-C. Chen, M.-L. Tong, I. A. Ansari, M. Raizada, M. Ahmad, M. Shahid and Z. A. Siddiqi, Dalton Trans., 2017, 46, 9801–9823 RSC.
- V. T. Yilmaz, Y. Topcu, F. Yilmaz and C. Thoene, Polyhedron, 2001, 20, 3209–3217 CrossRef CAS.
- J. Madarász, P. Bombicz, M. Czugler and G. Pokol, Polyhedron, 2000, 19, 457–463 CrossRef.
- G. Marin, V. Tudor, V. C. Kravtsov, M. Schmidtmann, Y. A. Simonov, A. Müller and M. Andruh, Cryst. Growth Des., 2005, 5, 279–282 CrossRef CAS.
- G. Xu, X. He, J. Lv, Z. Zhou, Z. Du and Y. Xie, Cryst. Growth Des., 2012, 12, 3619–3630 CrossRef CAS.
- T. A. Fernandes, M. V. Kirillova, V. André and A. M. Kirillov, Dalton Trans., 2018, 47, 16674–16683 RSC.
- I. Mantasha, M. Shahid, M. Ahmad, Rahisuddin, R. Arif, S. Tasneem, F. Sama and I. A. Ansari, New J. Chem., 2019, 43, 622–633 RSC.
- A. M. Kirillov, Y. Y. Karabach, M. Haukka, M. F. C. Guedes da Silva, J. Sanchiz, M. N. Kopylovich and A. J. L. Pombeiro, Inorg. Chem., 2008, 47, 162–175 CrossRef CAS PubMed.
- I. A. Ansari, F. Sama, M. Raizada, M. Shahid, M. Ahmad and Z. A. Siddiqi, New J. Chem., 2016, 40, 9840–9852 RSC.
- C. R. Groom, I. J. Bruno, M. P. Lightfoot and S. C. Ward, Acta Crystallogr., Sect. B: Struct. Sci., Cryst. Eng. Mater., 2016, 72, 171–179 CrossRef CAS PubMed.
- P. Seppälä, R. Sillanpää and A. Lehtonen, Coord. Chem. Rev., 2017, 347, 98–114 CrossRef.
- M. Andruh, Pure Appl. Chem., 2005, 77, 1685–1706 CrossRef CAS.
- M. Andruh, Chem. Commun., 2007, 2565–2577 RSC.
- A. M. Kirillov, M. V. Kirillova and A. J. L. Pombeiro, Coord. Chem. Rev., 2012, 256, 2741–2759 CrossRef CAS.
- A. M. Kirillov, M. N. Kopylovich, M. V. Kirillova, E. Y. Karabach, M. Haukka, M. F. C. Guedes da Silva and A. J. L. Pombeiro, Adv. Synth. Catal., 2006, 348, 159–174 CrossRef CAS.
- A. M. Kirillov, M. V. Kirillova, L. S. Shul’pina, P. J. Figiel, K. R. Gruenwald, M. F. C. Guedes da Silva, M. Haukka, A. J. L. Pombeiro and G. B. Shul’pin, J. Mol. Catal. A: Chem., 2011, 350, 26–34 CrossRef CAS.
- A. M. Kirillov, M. N. Kopylovich, M. V. Kirillova, M. Haukka, M. F. C. Guedes da Silva and A. J. L. Pombeiro, Angew. Chem., Int. Ed., 2005, 44, 4345–4349 CrossRef CAS PubMed.
- N. Podjed, P. Stare, R. Cerc Korošec, M. M. Alcaide, J. López-Serrano and B. Modec, New J. Chem., 2020, 44, 387–400 RSC.
- D. B. G. Williams and M. Lawton, J. Org. Chem., 2010, 75, 8351–8354 CrossRef CAS PubMed.
- B. Modec, N. Podjed and N. Lah, Molecules, 2020, 25, 1573 CrossRef CAS PubMed.
-
Agilent, CrysAlis PRO, Agilent Technologies Ltd, Yarnton, Oxfordshire, England, 2014 Search PubMed.
- O. V. Dolomanov, L. J. Bourhis, R. J. Gildea, J. A. K. Howard and H. Puschmann, J. Appl. Crystallogr., 2009, 42, 339–341 CrossRef CAS.
- G. M. Sheldrick, Acta Crystallogr., Sect. A: Found. Adv., 2015, 71, 3–8 CrossRef PubMed.
- G. M. Sheldrick, Acta Crystallogr., Sect. C: Struct. Chem., 2015, 71, 3–8 Search PubMed.
- A. L. Spek, Acta Crystallogr., Sect. D: Biol. Crystallogr., 2009, 65, 148–155 CrossRef CAS PubMed.
- L. J. Farrugia, J. Appl. Crystallogr., 2012, 45, 849–854 CrossRef CAS.
- C. F. Macrae, I. J. Bruno, J. A. Chisholm, P. R. Edgington, P. McCabe, E. Pidcock, L. Rodriguez-Monge, R. Taylor, J. van de Streek and P. A. Wood, J. Appl. Crystallogr., 2008, 41, 466–470 CrossRef CAS.
-
M. J. Frisch, G. W. Trucks, H. B. Schlegel, G. E. Scuseria, M. A. Robb, J. R. Cheeseman, G. Scalmani, V. Barone, B. Mennucci, G. A. Petersson, H. Nakatsuji, M. Caricato, X. Li, H. P. Hratchian, A. F. Izmaylov, J. Bloino, G. Zheng, J. L. Sonnenberg, M. Hada, M. Ehara, K. Toyota, R. Fukuda, J. Hasegawa, M. Ishida, T. Nakajima, Y. Honda, O. Kitao, H. Nakai, T. Vreven, J. A. Montgomery Jr., J. E. Peralta, F. Ogliaro, M. Bearpark, J. J. Heyd, E. Brothers, K. N. Kudin, V. N. Staroverov, T. Keith, R. Kobayashi, J. Normand, K. Raghavachari, A. Rendell, J. C. Burant, S. S. Iyengar, J. Tomasi, M. Cossi, N. Rega, J. M. Millam, M. Klene, J. E. Knox, J. B. Cross, V. Bakken, C. Adamo, J. Jaramillo, R. Gomperts, R. E. Stratmann, O. Yazyev, A. J. Austin, R. Cammi, C. Pomelli, J. W. Ochterski, R. L. Martin, K. Morokuma, V. G. Zakrzewski, G. A. Voth, P. Salvador, J. J. Dannenberg, S. Dapprich, A. D. Daniels, Ö. Farkas, J. B. Foresman, J. V. Ortiz, J. Cioslowski and D. J. Fox, Gaussian 09 (Revisions B.01 and E.01), Gaussian, Inc., Wallingford, CT, 2013 Search PubMed.
- G. A. Petersson, A. Bennett, T. G. Tensfeldt, M. A. Al-Laham, W. A. Shirley and J. Mantzaris, J. Chem. Phys., 1988, 89, 2193–2218 CrossRef CAS.
- G. A. Petersson and M. A. Al-Laham, J. Chem. Phys., 1991, 94, 6081–6090 CrossRef CAS.
- D. Andrae, U. Häußermann, M. Dolg, H. Stoll and H. Preuß, Theor. Chim. Acta, 1990, 77, 123–141 CrossRef CAS.
- A. D. Becke, J. Chem. Phys., 1993, 98, 5648–5652 CrossRef CAS.
- J. P. Perdew, J. A. Chevary, S. H. Vosko, K. A. Jackson, M. R. Pederson, D. J. Singh and C. Fiolhais, Phys. Rev. B: Condens. Matter Mater. Phys., 1992, 46, 6671–6687 CrossRef CAS PubMed.
- J. P. Perdew, Phys. B, 1991, 172, 1–6 CrossRef CAS.
- S. Grimme, S. Ehrlich and L. Goerigk, J. Comput. Chem., 2011, 32, 1456–1465 CrossRef CAS PubMed.
- A. V. Marenich, C. J. Cramer and D. G. Truhlar, J. Phys. Chem. B, 2009, 113, 6378–6396 CrossRef CAS PubMed.
- R. Seeger and J. A. Pople, J. Chem. Phys., 1977, 66, 3045–3050 CrossRef CAS.
- R. Bauernschmitt and R. Ahlrichs, J. Chem. Phys., 1996, 104, 9047–9052 CrossRef CAS.
-
R. F. W. Bader, Atom in Molecules: A Quantum Theory, Clarendon Press, Oxford,
UK, 1994 Search PubMed.
- T. Lu and F. Chen, J. Comput. Chem., 2012, 33, 580–592 CrossRef CAS PubMed.
- Multiwfn 3.6, http://sobereva.com/multiwfn/.
- E. R. Johnson, S. Keinan, P. Mori-Sánchez, J. Contreras-García, A. J. Cohen and W. Yang, J. Am. Chem. Soc., 2010, 132, 6498–6506 CrossRef CAS PubMed.
-
C. Y. Legault, CYLview20, Université de Sherbrooke, 2020, http://www.cylview.org.
-
C. E. Housecroft and A. G. Sharpe, Inorganic chemistry, Pearson, Harlow, England, 5th edn, 2018 Search PubMed.
- R. A. Coxall, S. G. Harris, D. K. Henderson, S. Parsons, P. A. Tasker and R. E. P. Winpenny, J. Chem. Soc., Dalton Trans., 2000, 2349–2356 RSC.
- A. W. Addison, T. N. Rao, J. Reedijk, J. van Rijn and G. C. Verschoor, J. Chem. Soc., Dalton Trans., 1984, 1349–1356 RSC.
- C. Janiak, J. Chem. Soc., Dalton Trans., 2000, 3885–3896 RSC.
- J. C. Bonner and M. E. Fisher, Phys. Rev., 1964, 135, A640–A658 CrossRef.
- W. E. Estes, D. P. Gavel, W. E. Hatfield and D. J. Hodgson, Inorg. Chem., 1978, 17, 1415–1421 CrossRef CAS.
- J. W. Hall, W. E. Marsh, R. R. Weller and W. E. Hatfield, Inorg. Chem., 1981, 20, 1033–1037 CrossRef CAS.
-
O. Kahn, Molecular magnetism, Wiley-VCH, New York, 1993 Search PubMed.
- V. H. Crawford, H. W. Richardson, J. R. Wasson, D. J. Hodgson and W. E. Hatfield, Inorg. Chem., 1976, 15, 2107–2110 CrossRef CAS.
- E. Ruiz, P. Alemany, S. Alvarez and J. Cano, J. Am. Chem. Soc., 1997, 119, 1297–1303 CrossRef CAS.
- E. Ruiz, P. Alemany, S. Alvarez and J. Cano, Inorg. Chem., 1997, 36, 3683–3688 CrossRef CAS PubMed.
- L. Merz and W. Haase, J. Chem. Soc., Dalton Trans., 1980, 875–879 RSC.
- B. Bleaney and K. D. Bowers, Proc. R. Soc. London, Ser. A, 1952, 214, 451–465 CAS.
- G. A. Baker, G. S. Rushbrooke and H. E. Gilbert, Phys. Rev., 1964, 135, A1272–A1277 CrossRef.
- M. Murugesu, R. Clérac, B. Pilawa, A. Mandel, C. E. Anson and A. K. Powell, Inorg. Chim. Acta, 2002, 337, 328–336 CrossRef CAS.
- G. B. Deacon and R. J. Phillips, Coord. Chem. Rev., 1980, 33, 227–250 CrossRef CAS.
-
N. B. Colthup, L. H. Daly and S. E. Wiberley, Introduction to Infrared and Raman Spectroscopy, Academic Press, San Diego, CA, 3rd edn, 1990 Search PubMed.
Footnote |
† Electronic supplementary information (ESI) available: Powder X-ray diffraction patterns (Fig. S1), crystallographic data (Tables S1–S3), ORTEP drawings of 1a and syn-8d (Fig. S2 and S3), intermolecular interactions (Fig. S4–S19 and Tables S5–S13), additional geometric parameters (Tables S4 and S14), details on DFT calculations (Tables S15–S17), optimized geometries and infrared spectroscopy data (Tables S18–S19, Fig. S20–S44). CCDC 2076993–2077015. For ESI and crystallographic data in CIF or other electronic format see DOI: 10.1039/d2nj00296e |
|
This journal is © The Royal Society of Chemistry and the Centre National de la Recherche Scientifique 2022 |