DOI:
10.1039/D1MD00313E
(Research Article)
RSC Med. Chem., 2022,
13, 90-97
NIR-II imaging of hepatocellular carcinoma based on a humanized anti-GPC3 antibody
Received
27th September 2021
, Accepted 3rd November 2021
First published on 23rd November 2021
Abstract
Liver cancer, of which hepatocellular carcinoma (HCC) is the most common form, is one of the most lethal cancers worldwide. The five-year survival rate for HCC is below 9%, which can be attributed to late diagnosis and limited treatment options at the late stage. Therefore, safe and efficient imaging strategies are urgently needed to facilitate HCC diagnosis and stage evaluation. The development of the second near infrared window (NIR-II, 1000–1700 nm) fluorescence imaging offers the advantages of enhanced resolutions, deeper penetration depth, and less autofluorescence compared to traditional NIR-I window (700–900 nm) imaging. Herein, an HCC targeted NIR-II fluorescent probe, GPC-ICG, was developed by labelling a humanized anti-GPC3 monoclonal antibody with indocyanine green (ICG). Compared to the negative control IgG-ICG probe, the GPC3-ICG probe demonstrated specific GPC3 targeting capability in vitro. And for GPC3 positive Huh-7 tumor bearing mice, the GPC3-ICG probe specifically accumulated in subcutaneous xenografts, with a tumor–background ratio (TBR) of up to 3. The NIR-II imaging of mice organs ex vivo also indicated that GPC3-ICG specifically targeted Huh-7 tumor tissue. Overall, GPC3-ICG is a promising NIR-II probe for GPC3 targeted imaging of HCC.
Background
Liver cancer is a leading cause of cancer-related death worldwide, especially in developing countries. The most common form of liver cancer is hepatocellular carcinoma (HCC), which is malignant with a reported five-year survival rate below 9%.1 Most HCC patients suffer from chronic liver diseases such as chronic infections with the hepatitis B or C virus, cirrhosis, and non-alcoholic fatty liver disease, which progress into HCC.2–4 Therefore, developing a safe and efficient method for early diagnosis, accurate tracking of HCC tumor tissue, and disease stage evaluation is essential for improving patient survival.5–7
Several imaging modalities are traditionally used in evaluating liver diseases and screening HCC, such as ultrasonography (US), computed tomography (CT), magnetic resonance imaging (MRI), positron emission tomography (PET), and angiography.6–9 Recent clinical studies using optical imaging show the huge potential of this modality, especially with its advantage of being non-invasive.10,11 While optical imaging with wavelengths in the visible spectrum (400–700 nm) and traditional near infrared window I (NIR-I, 700–900 nm) faces some challenges like poor penetration depth and resolution, and autofluorescence,12,13 recent studies suggest that fluorescence imaging in the second near infrared window (NIR-II, 1000–1700 nm) can partially overcome the aforementioned limitations, and NIR-II imaging provides fluorescence images with high signal to background ratios.14–17 Therefore it is desirable to develop appropriate NIR-II probes for imaging different diseases and targets.
Indocyanine green (ICG) is a Food and Drug Administration approved dye for NIR-I imaging in routine clinical use.18–20 Recent studies have found that ICG exhibits a fluorescence signal beyond 1000 nm, and it provides better fluorescence imaging quality in the NIR-II window than in the NIR-I region.21–23 Moreover, the first-in-human study of ICG based NIR-II imaging guided liver-tumor surgery has been reported recently.24 Intraoperative NIR-II imaging has shown higher tumor-detection sensitivity and imaging quality than traditional NIR-I imaging.24 Therefore, ICG as an optical dye covering both NIR-I and II imaging shows high potential for further developing targeted molecular imaging probes.
In developing disease-targeted molecular imaging probes, molecular targets and binding ligands are crucial. Numerous HCC related biomarkers have been reported in previous studies, including glypican-3 (GPC3), asialoglycoprotein receptors, glycyrrhetinic acid receptors, CD71, homodimeric glycoproteins, somatostatin receptors, CD44, lysosomal-associated protein transmembrane-4β, and retinoic acid receptors.25,26 Among them, GPC3 is an attractive target for HCC imaging. It is a member of the heparan sulfate proteoglycan family and is bound to the cell surface by glycosylphosphatidylinositol (GPI) anchors. GPC3 is specifically over-expressed in over 50% of HCC patients, and its over-expression is correlated with the poor prognosis in HCC patients.27–30 Therefore, ligands targeting the GPC3 protein, such as peptides, antibodies, and aptamers, have been developed for HCC diagnosis and treatment.31–34
In our previous study, a mouse anti-GPC3 monoclonal antibody (mAb) was radiolabelled with 89Zr for positron emission tomography (PET) of HCC patient-derived xenograft (PDX) models. This study demonstrates that the 89Z labelled GPC mAb can achieve high performance in imaging of GPC3-expressing HCC in PDX models.35 In order to avoid the hazardous ionizing radiation and for furthering the potential of optical imaging guided surgery of HCC, here we aimed to develop a GPC3 targeted probe for HCC NIR-II imaging. Specifically, we conjugated the ICG-NHS ester with a novel humanized anti-GPC3 mAb to prepare the probe, GPC3-ICG. We hypothesized that the GPC3-ICG probe can specifically identify GPC3-positive HCC from normal tissue. We further tested the probe in vitro and in vivo and compared its performance to the negative control IgG-ICG probe.
Materials and methods
Synthesis of fluorescence probes
The probes were synthesized according to a previous study.36 A humanized anti-human GPC3 antibody (clone H3K3) was generated by inserting the complementarity determining region (CDR) of a mouse origin anti-GPC3 antibody (clone 1G12) into the human antibody scaffold (this work was done by Creative Biolabs, Inc, Shirely, NY). Anti-human GPC3 mAb or non-targeted human IgG (Sigma-Aldrich, St Louis, MO) in phosphate-buffered saline (PBS) with a concentration of 1 mg mL−1 was adjusted to pH 7.2–7.4. Then ICG-NHS (SwissLumix Sarl, Lausanne, Switzerland) in anhydrous dimethyl sulfoxide was added at a 1
:
30 antibody to ICG dye molar ratio. After incubation at room temperature for 4 hours, the conjugated probes were purified using desalting columns (Thermo Scientific™ Zeba™ Spin Desalting Columns, Thermo Scientific, Waltham, MA).
Characterization of the probes
The absorption of GPC3-ICG was measured, and the 780 nm absorption referred to the ICG concentration.37,38 The antibody concentration was determined by the BCA protein assay (Thermo Scientific™ Pierce™ BCA Kit). To confirm the conjugation of ICG molecules with each antibody molecule, the Beer–Lambert law was used:
Absorption spectra of the GPC3-ICG and IgG-ICG probes were obtained using an ultraviolet-visible-NIR Cary 60 spectrometer (Agilent Technologies, Santa Clara, CA) with background correction. Emission fluorescence spectra were captured using a FluoroMax-4 spectrofluorometer (HORIBA Scientific, JobinYvon, France), at an emission wavelength of 719 nm.
Sodium dodecyl sulfate-polyacrylamide gel electrophoresis (SDS-PAGE) was used to confirm the conjugation between ICG and antibodies. All samples were pretreated with a reducing agent and incubated at 80 °C for 10 minutes. Then, the antibodies labelled with or without ICG were loaded into each well for SDS-PAGE. The NIR-II images of the gel were captured under excitation using an 808 nm laser, followed by Coomassie brilliant blue R250 staining and image capture. To evaluate stability, GPC3-ICG in PBS solution containing 50% FBS was incubated at 37 °C for 5 days. The fluorescence intensity of the mixture was measured every day.
Cell culture
Human hepatocellular carcinoma cell lines Huh-7, Hep G2, and Hep 3B were cultured in Dulbecco's modified Eagle's medium (DMEM) and Eagle's minimum essential medium (EMEM). The medium contained 10% fetal bovine serum (FBS) and 1% antibiotics (penicillin, streptomycin), and cells were cultured at 37 °C in a humidified incubator (Thermo Fisher Scientific, Waltham, MA) with 5% atmospheric CO2. Cells were passaged every 3–4 days. The GPC3 protein expression level of these cell lines was determined and reported in our previous studies.35
In vitro cell studies
The hepatocellular carcinoma cell lines (Huh-7, Hep G2, and Hep 3B) were seeded in a 6-well plate at a density of 5 × 105 cells per well and cultured overnight. The GPC3-ICG and IgG-ICG probes (containing 0.5 μg ICG) were added into each well. After incubation for 40 hours, cells were harvested, and cell pellets were washed twice with PBS and then resuspended in 50 μL of PBS for NIR-II imaging.
Establishment of subcutaneous Huh-7 xenografts in mice
Animal studies were approved and performed in compliance with the Institutional Animal Care and Use Committee (IACUC) at Stanford University (protocol ID: 11580). The experiment was set up by generating subcutaneous xenografts from Huh-7 cell lines as previously described.39,40 Huh-7 cells (2 × 106 cells), obtained from the American Type Cell Culture (ATCC PTA-4583), were suspended in 100 μL of Dulbecco's phosphate buffered saline (DPBS) (Invitrogen Life Technologies, Carlsbad, California), mixed with 100 μL of Corning Matrigel membrane matrix (354234, Corning, Bedford, MA) and injected subcutaneously near the left shoulder of 6–8-week-old male nude mice (Hfh11nu, obtained from The Jackson Laboratory, Bar Harbor, ME). The volume of the tumors was measured twice a week, and then the imaging study was performed when the size of the tumors was about 150 mm3.
In vivo NIR-II imaging of subcutaneous Huh-7 xenografts
Huh-7 tumor bearing mice were divided randomly into two groups (n = 4 each). The same amount of GPC3-ICG and IgG-ICG probes in PBS (contain 4.2 μg ICG) was injected into the mice through the tail vein. NIR-II images were then captured at 4, 24, 48, 72, 96, 144, and 192 hours post-injection. One mouse at 96 h post-injection was chosen in each group randomly and then sacrificed, and the tumor and main organs were harvested for imaging.
All NIR-II images were collected on a two-dimensional InGaAs array NIR-II system (Princeton Instruments, Trenton, NJ). Excitation was carried out using an 808 nm diode laser with an 850 nm filter. Fluorescence emission was collected with a 1000 nm filter (Thorlabs, Newton, NJ).
Image data processing
The region of interest (ROI) for the signal intensity was measured using Image J software (NIH, Bethesda, MD). The tumor-to-background ratio (TBR) was calculated based on the ratio of the mean fluorescence intensity of the tumor region to that of the background region. In this study, the muscle region on the forelimb was chosen as the background.
Statistical analysis
The absorbance spectra and fluorescence spectra were normalized using GraphPad Prism 8 (GraphPad Software Inc, La Jolla, CA) for Windows. Statistical data and continuous variables were expressed as means ± standard deviation (SD). The t-test was used to perform all inter-group comparisons. Statistical significance was defined as p < 0.05.
Results and discussion
Synthesis and characterization of the GPC3-ICG probe
A schematic representation of the antibody-ICG probe is shown in Fig. 1a. After purification using a 7 kDa desalting column, the probes (GPC3-ICG and IgG-ICG) were obtained as green aqueous solutions. The optical properties of these probes compared with those of free ICG were then determined. The absorption spectra of GPC3-ICG, IgG-ICG, and free ICG were similar in the 550–1000 nm wavelength range (Fig. 1b). The conjugation reaction between ICG and the antibody does not affect the absorbance of ICG at 808 nm which is used as the excitation wavelength in our subsequent studies. The probes also showed an obvious absorbance peak at 280 nm, which is generally regarded as the featured absorbance peak of the protein (Fig. 1c). To investigate the emission properties of the probes, NIR-I fluorescence spectra were obtained, and the results showed that the GPC3-ICG and IgG-ICG probes displayed the same fluorescence spectrum under 719 nm wavelength excitation. Compared to free ICG, the probes showed an about 10 nm redshift because of its conjugation with a protein (Fig. 1d). For NIR-II fluorescence emission properties, under 808 nm laser irradiation, both IgG-ICG and GPC3-ICG showed strong NIR-II fluorescence as expected (Fig. 1e). Therefore, the probes prepared were used for NIR-II imaging in the following studies.
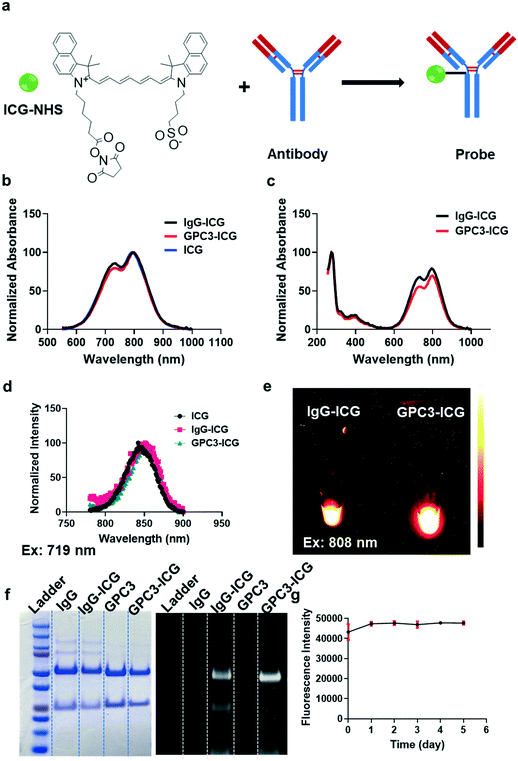 |
| Fig. 1 Synthesis and characterization of GPC3-ICG and IgG-ICG. (a) A schematic representation of the synthesis of GPC3-ICG and IgG-ICG. (b) Absorbance spectra of IgG-ICG, GPC3-ICG, and ICG between 550 and 1000 nm. (c) Absorbance spectra of IgG-ICG and GPC3-ICG between 250 and 1000 nm. (d) Fluorescence spectra of ICG, IgG-ICG, and GPC3-ICG under irradiation at 719 nm. (e) NIR-II imaging of IgG-ICG and GPC3-ICG with 808 nm laser irradiation. (f) SDS-PAGE of IgG, IgG-ICG, GPC3, and GPC3-ICG. The gel was stained using coomassie brilliant blue R250 (left panel) and captured by NIR-II imaging (right panel). (g) The stability of GPC3-ICG in FBS for 5 days at 37 °C. Data are presented as mean ± SD, n = 3. | |
After successful synthesis of both probes, we verified the conjugation between ICG and the antibodies by SDS-PAGE. After treatment with a reducing agent, GPC3-ICG and IgG-ICG were each separated into two clear protein bands (the light chain and heavy chain of the antibodies), and the positions were the same with those of the free GPC3 and IgG proteins (Fig. 1f, left panel). Additionally, the protein bands of GPC3-ICG and IgG-ICG were clearly displayed in gel, and they also had a NIR-II fluorescence signal under 808 nm laser irradiation (Fig. 1f, right panel). These results indicated that the conjugation was successful. Meanwhile, it was found that each antibody was labeled with two ICG molecules. Moreover, GPC3-ICG also exhibited excellent stability in FBS solution at 37 °C, and the NIR-II fluorescence intensity barely decreased for up to five days (Fig. 1g).
GPC3-ICG and IgG-ICG were successfully prepared, and they showed similar optical properties to those of free ICG. All these data suggest that GPC3-ICG and IgG-ICG are suitable for further investigation for NIR-II imaging in vitro and in vivo.
Cell uptake of GPC3-ICG
Cell uptake experiments with different GPC3 positive HCC cell lines including Huh-7, Hep G2, and Hep 3B were performed to evaluate the cellular uptake efficiency of the GPC3-ICG and IgG-ICG probes. After incubation with different probes, cell pellets were collected, and the NIR-II images were captured. Fig. 2a shows that GPC3-ICG was specifically uptaken by these HCC cells at a high level, while IgG-ICG showed minimum accumulation in the cells. The different cell lines also displayed different efficiencies for the uptake of GPC3-ICG as expected, which is caused by their different GPC3 expression levels. The Hep G2 cell line exhibited the highest GPC3 expression level among the three cell lines, and Hep 3B and Huh-7 showed similar expression levels of GPC3 in our previous study.35 Quantification analysis of the fluorescence signal intensities in each cell pellet is shown in Fig. 2b. Significant differences were observed between the GPC3-ICG and IgG-ICG groups (p < 0.001). GPC3-ICG demonstrated excellent targeting efficiency and specificity in these HCC cells.
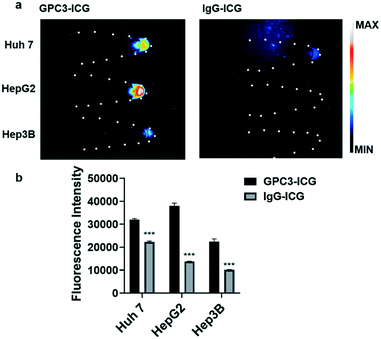 |
| Fig. 2 NIR-II probe uptake in HCC cell lines. (a) Different probes (left GPC3-ICG; right IgG-ICG) were incubated with Huh-7, Hep G2, and Hep 3B cell lines, and NIR-II images of the cell pellets were captured. (b) Quantification analysis of the NIR-II fluorescence intensity in different cell lines. The error bars indicate mean ± SD, **p < 0.01 with two-tailed Student's t-test. | |
NIR-II imaging of the HCC tumor model
When the size of the Huh-7 subcutaneous xenografts grew to about 150 cm3, GPC3-ICG or IgG-ICG was injected into mice through the tail vein. The NIR-II fluorescence images were captured under 808 nm laser irradiation at different time points and quantified using Image J software.
The GPC3-ICG probe was observed to accumulate in the xenografts at 96 hours post-injection (Fig. 3a). The xenografts could be easily distinguished from the normal tissues by NIR-II imaging. A much less intense signal was detected in the xenografts after injection with the negative control IgG-ICG. It was hard to delineate the tumor from normal tissue from the NIR-II images, and only the liver and spleen showed observable fluorescence signals (Fig. 3a). Quantification of the NIR-II fluorescence intensities in the xenografts showed that the intensity in the GPC3-ICG group is about 1.7 times higher than that in the IgG-ICG group (Fig. 3b). Moreover, for GPC3-ICG, the TBR reached up to 3 at 96 hours post-injection, while the TBR for the IgG-ICG group was only 1.6. In a previous study, ICG without a target ligand just showed 2.25 TBR in Hep G2 tumor-bearing mice.24 GPC3-ICG showed specific HCC targeted imaging capability.
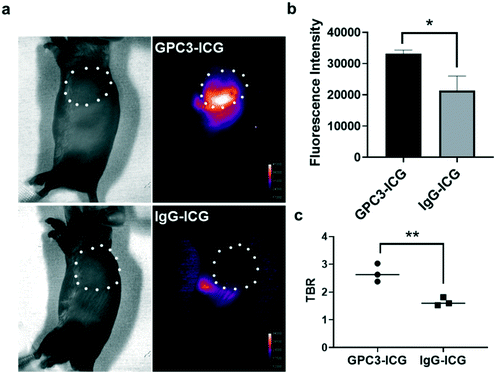 |
| Fig. 3 NIR-II imaging of the Huh-7 subcutaneous xenograft models. (a) NIR-II images of mice at 96 hours post-injection of the probe GPC3-ICG or IgG-ICG. White circles indicate the xenografts. (b) Quantification of fluorescence intensities in the xenografts. (c) The tumor background ratio (TBR) was calculated. *p < 0.05; **p < 0.01 with two-tailed Student's t-test. The error bars indicate means ± S.D. (n = 3). | |
To evaluate the clearance efficiency of the probes, the NIR-II images of the xenografts (left lateral) and normal livers (supine) were captured every day until the probes could not be detected. Fig. 4a indicates that the mice showed a significant NIR-II fluorescence signal after injection of the probes GPC3-ICG and IgG-ICG. The fluorescence intensity of the livers became weaker and weaker with the clearance of the probes, and after 192 hours, the probes were barely detectable. However, within the xenografts, GPC3-ICG and IgG-ICG behaved differently. GPC3-ICG accumulated in the xenografts for up to 144 hours. But the IgG-ICG group did not show much signal enhancement in the xenografts compared to the GPC3-ICG group (Fig. 4a). The quantification analysis results indicated that GPC3-ICG accumulated in the xenografts, with the highest fluorescence intensity at 48 hours post-injection, and the probe remained in the xenografts for up to 144 hours post-injection (Fig. 4b). Meanwhile, for IgG-ICG, it did not show a long-term accumulation behaviour, and the NIR-II fluorescence signal continuously decreased after administration (Fig. 4b). The different accumulation behaviours of GPC3-ICG and IgG-ICG in xenograft tissue are caused by the specific interaction of the anti-GPC3 antibody and GPC3 protein.
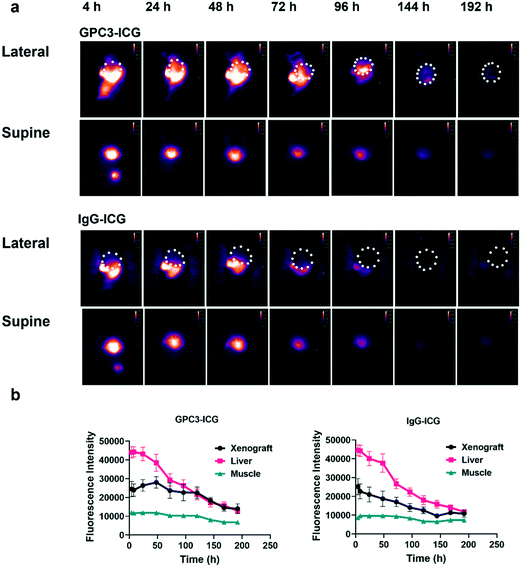 |
| Fig. 4 Accumulation and clearance of the probes in vivo. (a) NIR-II images of the mice after injection of GPC3-ICG (upper panel) or IgG-ICG (lower panel). White circles indicate the tumor tissues with the mice in the left lateral recumbent. NIR-II images were also captured with mice in the supine position to show the fluorescence signals in livers. (b). The error bars indicate means ± S.D. (n = 3). | |
One mouse in each group was sacrificed at 96 hours post-injection to further confirm the biodistribution of GPC3-ICG and IgG-ICG. The xenografts and major organs including the liver, spleen, heart, kidneys, lungs, stomach, and intestines were harvested and imaged ex vivo. For both the GPC3-ICG and IgG-ICG probes, the highest NIR-II fluorescence intensities were observed in the liver and then the spleen (Fig. 5a and b). Only GPC3-ICG (and not IgG-ICG) showed NIR-II fluorescence signals in the xenografts.
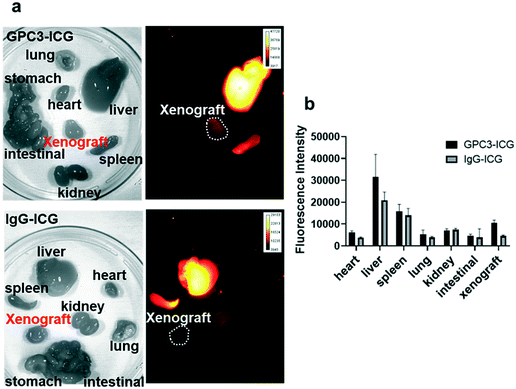 |
| Fig. 5 Biodistribution of GPC3-ICG and IgG-ICG. (a) Ex vivo NIR-II images of the xenografts and major organs (upper panels for GPC3-ICG and bottom panels for IgG-ICG; white circles indicate the xenografts). (b) Quantification of the fluorescence intensities in the xenografts and major organs. The error bars indicate means ± S.D. in the ROI. | |
All the above results further confirmed that the GPC3 targeted probe was able to image the HCC and showed a favourable imaging performance compared to the control probe. And compared to a previous study, GPC3-ICG also exhibited a better TBR than ICG. The humanization of the anti-GPC3 antibody was proposed to minimize the immune response to the antibody. It is expected that GPC3-ICG has the potential to be used for image-guided surgery in clinic.
In this study, we chose the FDA-approved ICG instead of a novel chemical molecule and the humanized anti-GPC3 antibody for synthesizing the probe, because we are aiming to develop a probe with clinically translatable potential. And the standard method can provide a platform for developing more NIR-II probes.
Conclusion
A GPC3 targeted probe, GPC3-ICG, has been synthesized. It shows specific cell uptakes in different GPC3 positive HCC cell lines. GPC3-ICG also demonstrates specific HCC targeted imaging capability, and it can clearly delineate the tumor; the TBR can reach 3 at 96 hours. Overall, GPC3-ICG is a promising NIR-II probe for HCC imaging and has the potential for future translation into clinical applications.
Author contributions
Hui Shi conceived and designed the experiments, under the supervision of Mei-Sze Chua, Zhen Cheng, and Samuel So. Hui Shi performed the fluorescence imaging experiments. Lakshmi Vageesh Huttad and Mingdian Tan developed the animal models. Hongguang Liu provided the synthesis method. All the authors participated in the interpretation of the reported experiments or results. Hui Shi, Mei-Sze Chua and Zhen Cheng wrote and revised the manuscript.
Conflicts of interest
The authors declare that the research was conducted in the absence of any commercial or financial relationships that could be construed as a potential conflict of interest.
References
- A. S. Darvesh, B. B. Aggarwal and A. Bishayee, Curr. Pharm. Biotechnol., 2012, 13, 218–228 CAS.
- J. D. Yang and L. R. Roberts, Nat. Rev. Gastroenterol. Hepatol., 2010, 7, 448–458 CrossRef PubMed.
- J. Balogh, D. Victor, 3rd, E. H. Asham, S. G. Burroughs, M. Boktour, A. Saharia, X. Li, R. M. Ghobrial and H. P. Monsour Jr., J. Hepatocell. Carcinoma, 2016, 3, 41–53 CrossRef PubMed.
- Q. M. Anstee, H. L. Reeves, E. Kotsiliti, O. Govaere and M. Heikenwalder, Nat. Rev. Gastroenterol. Hepatol., 2019, 16, 411–428 CrossRef PubMed.
- S. Lin, K. Hoffmann and P. Schemmer, Liver Cancer, 2012, 1, 144–158 CrossRef CAS PubMed.
- J. Fu and H. Wang, Cancer Lett., 2018, 412, 283–288 CrossRef CAS PubMed.
- C. Ayuso, J. Rimola and A. N. García-Criado, Abdom. Imaging, 2012, 37, 215–230 CrossRef.
- T. Hennedige and S. K. Venkatesh, Cancer Imaging, 2013, 12, 530–547 CrossRef PubMed.
- B. I. Choi and J. M. Lee, J. Hepatobiliary Pancreat. Sci., 2009, 17, 369–373 CrossRef.
- Y. Zhang, D. H. Brooks, M. A. Franceschini and D. A. Boas, J. Biomed. Opt., 2005, 10, 011014 CrossRef.
- I. Georgakoudi and K. P. Quinn, Annu. Rev. Biomed. Eng., 2012, 14, 351–367 CrossRef CAS PubMed.
- E. İ. Altınoğlu and J. H. Adair, Wiley Interdiscip. Rev.: Nanomed. Nanobiotechnol., 2010, 2, 461–477 Search PubMed.
- G. Hong, A. L. Antaris and H. Dai, Nat. Biomed. Eng., 2017, 1, 1–22 CrossRef.
- F. Ding, Y. Zhan, X. Lu and Y. Sun, Chem. Sci., 2018, 9, 4370–4380 RSC.
- G. Hong, J. C. Lee, J. T. Robinson, U. Raaz, L. Xie, N. F. Huang, J. P. Cooke and H. Dai, Nat. Med., 2012, 18, 1841–1846 CrossRef CAS.
- A. L. Antaris, H. Chen, K. Cheng, Y. Sun, G. Hong, C. Qu, S. Diao, Z. Deng, X. Hu, B. Zhang, X. Zhang, O. K. Yaghi, Z. R. Alamparambil, X. Hong, Z. Cheng and H. Dai, Nat. Mater., 2015, 15, 235–242 CrossRef.
- G. Hong, J. T. Robinson, Y. Zhang, S. Diao, A. L. Antaris, Q. Wang and H. Dai, Angew. Chem., Int. Ed., 2012, 51, 9818–9821 CrossRef CAS.
- L. van Manen, H. J. M. Handgraaf, M. Diana, J. Dijkstra, T. Ishizawa, A. L. Vahrmeijer and J. S. D. Mieog, J. Surg. Oncol., 2018, 118, 283–300 CrossRef.
- M. V. Marshall, J. C. Rasmussen, I. C. Tan, M. B. Aldrich, K. E. Adams, X. Wang, C. E. Fife, E. A. Maus, L. A. Smith and E. M. Sevick-Muraca, Open Surg. Oncol. J., 2010, 2, 12–25 Search PubMed.
- M. Plante, O. Touhami, X.-B. Trinh, M.-C. Renaud, A. Sebastianelli, K. Grondin and J. Gregoire, Gynecol. Oncol., 2015, 137, 443–447 CrossRef.
- A. L. Antaris, H. Chen, S. Diao, Z. Ma, Z. Zhang, S. Zhu, J. Wang, A. X. Lozano, Q. Fan, L. Chew, M. Zhu, K. Cheng, X. Hong, H. Dai and Z. Cheng, Nat. Commun., 2017, 8, 15269 CrossRef CAS PubMed.
- Z. Starosolski, R. Bhavane, K. B. Ghaghada, S. A. Vasudevan, A. Kaay and A. Annapragada, PLoS One, 2017, 12, e0187563 CrossRef.
- J. A. Carr, D. Franke, J. R. Caram, C. F. Perkinson, M. Saif, V. Askoxylakis, M. Datta, D. Fukumura, R. K. Jain, M. G. Bawendi and O. T. Bruns, Proc. Natl. Acad. Sci. U. S. A., 2018, 115, 4465–4470 CrossRef CAS.
- Z. Hu, C. Fang, B. Li, Z. Zhang, C. Cao, M. Cai, S. Su, X. Sun, X. Shi, C. Li, T. Zhou, Y. Zhang, C. Chi, P. He, X. Xia, Y. Chen, S. S. Gambhir, Z. Cheng and J. Tian, Nat. Biomed. Eng., 2020, 4, 259–271 CrossRef.
- C. R. de Lope, S. Tremosini, A. Forner, M. Reig and J. Bruix, J. Hepatol., 2012, 56, S75–S87 CrossRef.
- F. Zhou, W. Shang, X. Yu and J. Tian, Med. Res. Rev., 2018, 38, 741–767 CrossRef CAS PubMed.
- B. Sun, Z. Huang, B. Wang, Y. Yu, S. Lin, L. Luo, Y. Wang and Z. Huang, Med. Sci. Monit., 2017, 23, 850–855 CrossRef CAS PubMed.
- H. Shirakawa, H. Suzuki, M. Shimomura, M. Kojima, N. Gotohda, S. Takahashi, T. Nakagohri, M. Konishi, N. Kobayashi, T. Kinoshita and T. Nakatsura, Cancer Sci., 2009, 100, 1403–1407 CrossRef CAS PubMed.
- N. Yamauchi, A. Watanabe, M. Hishinuma, K. Ohashi, Y. Midorikawa, Y. Morishita, T. Niki, J. Shibahara, M. Mori, M. Makuuchi, Y. Hippo, T. Kodama, H. Iwanari, H. Aburatani and M. Fukayama, Mod. Pathol., 2005, 18, 1591–1598 CrossRef CAS.
- M. Capurro, I. R. Wanless, M. Sherman, G. Deboer, W. Shi, E. Miyoshi and J. Filmus, Gastroenterology, 2003, 125, 89–97 CrossRef CAS.
- Y. L. Lee, B. C. Ahn, Y. Lee, S. W. Lee, J. Y. Cho and J. Lee, J. Pept. Sci., 2011, 17, 763–769 CrossRef CAS.
- J. O. Park, Z. Stephen, C. Sun, O. Veiseh, F. M. Kievit, C. Fang, M. Leung, H. Mok and M. Zhang, Mol. Imaging, 2011, 10, 69–77 CrossRef CAS.
- K. Ofuji, K. Saito, T. Yoshikawa and T. Nakatsura, J. Hepatocell. Carcinoma, 2014, 1, 35–42 Search PubMed.
- H. H. Han, Y. J. Qiu, Y. Y. Shi, W. Wen, X. P. He, L. W. Dong, Y. X. Tan, Y. T. Long, H. Tian and H. Y. Wang, Theranostics, 2018, 8, 3268–3274 CrossRef CAS PubMed.
- X. Yang, H. Liu, C. K. Sun, A. Natarajan, X. Hu, X. Wang, M. Allegretta, R. D. Guttmann, S. S. Gambhir, M. S. Chua, Z. Cheng and S. K. So, Biomaterials, 2014, 35, 6964–6971 CrossRef CAS PubMed.
- Y. Suo, F. Wu, P. Xu, H. Shi, T. Wang, H. Liu and Z. Cheng, Adv. Healthcare Mater., 2019, 8, e1900974 CrossRef PubMed.
- M. Ogawa, N. Kosaka, P. L. Choyke and H. Kobayashi, Cancer Res., 2009, 69, 1268–1272 CrossRef CAS PubMed.
- E. M. McCorquodale and C. L. Colyer, Electrophoresis, 2001, 22, 2403–2408 CrossRef CAS.
- W. Wei, M.-S. Chua, S. Grepper and S. K. So, Mol. Cancer, 2011, 10, 1–12 CrossRef PubMed.
- W. Wei, M. S. Chua, S. Grepper and S. K. So, Mol. Cancer, 2009, 8, 76 CrossRef.
|
This journal is © The Royal Society of Chemistry 2022 |
Click here to see how this site uses Cookies. View our privacy policy here.