DOI:
10.1039/D2MA00868H
(Paper)
Mater. Adv., 2022,
3, 8780-8791
Synthesis and ionic conductivity of calcium-doped ceria relevant to solid oxide fuel cell applications†
Received
16th August 2022
, Accepted 2nd October 2022
First published on 13th October 2022
Abstract
Towards the development of green energy devices, it is necessary to focus on commercial electrolyte materials for intermediate temperature solid oxide fuel cells (IT-SOFCs). Ca-doped ceria (CDC) samples having a composition of Ce(1−x)CaxO2−δ (0.03 ≤ x ≤ 0.1) were synthesized by a facile solid-state route and sintered at a lower temperature (1473 K). X-ray diffraction, Raman, X-ray photoelectron, Fourier-transform infrared, UV–VIS diffuse reflectance, field emission scanning electron microscopy – energy dispersive X-ray with elemental mapping, and electrochemical impedance spectroscopy techniques were used for the characterization of these CDC samples. The 0.10 CDC showed high oxide ion conductivity of 8.01 × 10−3 S cm−1 at 973 K with a lower activation energy of 0.78 eV. The 0.03 CDC, 0.05 CDC, and 0.07 CDC samples exhibited ionic conductivities of 1.66 × 10−4, 4.42 × 10−3, and 5.76 × 10−3 S cm−1 at 973 K with activation energies of 1.65, 1.01, and 0.92 eV, respectively. The present work aims to develop Ca-doped ceria as economically viable electrolytes for IT-SOFCs.
Introduction
Solid oxide fuel cells (SOFCs) are known to convey high electrical proficiency and fuel adaptability with ensured natural advantages. In SOFCs, the hopping mechanism regulates the ions through electrolytes between the cathode and anode. The high ionic and insignificant electronic conductivity, permanence in redox conditions, mechanical strength (among other qualities) are viewed as great properties for electrolyte materials. An assortment of oxide-ion electrolytes have been examined up to this point, similar to zirconia-based oxides, cubic fluorite structure based doped ceria, stabilized δ-Bi2O3 and others. The issues related to their cost, electronic conduction, working and sintering temperature discourage its mass scale commercialization.1 The doped ceria-based ceramics were found to show almost the same results at intermediate temperature (IT), 773–973 K for SOFC applications. The conduction mechanism in doped ceria was influenced by the mobility and distribution of oxygen vacancies. It is created by substituting suitable cations with lower valences than the host lattice ions. The rare earth-doped ceria-based materials have been examined because of their ability to yield high ionic conductivity at lower temperatures and are thus viewed as a prospective electrolyte for IT-SOFCs.2,3 The Sm and Gd ionic radii coordinate well with Ce ions, and hence high oxide-ion conductivity has been accounted for GDC10 and SDC20. The Gd-, Sm-, and Y-doped ceria having a high dopant concentration of 15–20 mol%, showed improved ionic conductivity at elevated temperatures.4,5 In any case, the ideal ionic conductivity at lower temperatures actually has not been accomplished, and subsequently, their expense for 10–20 mol% dopant amount is not economically reasonable.6 Thus, there exists an expansive degree in the advancement of new economically reasonable ceria-based electrolyte materials. The alkaline earth-doped ceria materials have been viewed as substantially more powerful and potential candidate to yield huge ionic conductivity at lower temperatures. For example, Ce (ri = 1.11 Å) doped with Ca (ri = 1.12 Å) has been researched among the alkaline earth-doped ceria-based materials. The ionic conductivities of alkaline earth-doped ceria-based materials are viewed as critically as those of rare earth-doped materials. A high conductivity on the order of 10−3 S cm−1 was reported by Yamashita et al.7 at 873 K for the Ce0.9Ca0.1O2−δ composition. Similarly, Banerjee et al.8 reported 1.29 × 10−2 S cm−1 for oxide ion conductivity at 873 K for the Ce0.8Ca0.2O2−δ composition prepared through the auto-combustion method. Calcium is abundant in nature and cheaper than other rare earth elements,9 and its doping is found to create higher oxygen vacancies. Therefore, it has been regarded as an excellent and advanced oxygen storage material.10 Subsequently, for economical reasonability, cheap dopants such as Ca having sufficient electrochemical performance have been considered favorable. Recently, Schottky junction (SJ)-based n or p-type low-cost semiconductor – ionic conductor composite materials have shown potential to cause in-filed barriers and confine the electron's mobility or holes from the passage of the electrolyte edge to the opposite side. Thus, this prevents the short-circuiting in the SOFCs and may be accountable for the colossal ionic conductivity.11–22 Still, there are few studies related to the Ca-doped ceria framework for IT-SOFCs. From the point of the ionic-radius, compatibility of the dopant, commercial viability, and abundant nature, calcium (Ca) has been picked as a dopant for the host cerium cross-section. In the present work, the CDC samples were prepared by the conventional solid-state method, and calcium has likewise been viewed as a sintering aid for the ceria-based electrolytes.23 Sintering ceria-based electrolytes above 1573 K prompts the reduction of some Ce4+ to Ce3+ ions, causing electronic leakage and affecting the performance of the cell.24 Therefore, attempts have been made to achieve the high densification at a lower sintering temperature of 1473 K than the required high-temperature sintering in the range of 1873–1973 K (Yamashita et al. 1995).8 The oxide ion conductivities of CDC samples are studied as a function of dopant concentrations between the temperature range of 623–973 K in air using electrochemical impedance spectroscopy. This work accordingly plans to investigate the new low-cost Ca-doped ceria ceramics for IT-SOFCs.25
Experimental
The Ca-doped CeO2 ceramic oxide powders with the composition Ce1−xCaxO2−δ (x = 0.03, 0.05, 0.07 and 0.1) are prepared from conventional solid-state route by using cerium oxide [A.R grade 99.99% pure, Sd-Fine Mumbai-India] and hydrated calcium acetate [C4H6O4Ca·XH2O]; [A.R grade 99.99% pure, Sd-Fine Mumbai-India]. The stoichiometric ratios of precursors were blended with isopropyl alcohol manually for 1 h, and calcined at 1273 K for 6 h in a muffle furnace. The obtained powders were again ground and pelletized (10 ϕ × 2 mm dimensions), and sintered at 1473 K in the air for 4 h. The final obtained products having different compositions are hereinafter referred to as 0.03 CDC, 0.05 CDC, 0.07 CDC and 0.10 CDC individually.
Characterization
The XRD analysis of CDC samples was carried out using a Rigaku MiniFlex 600 powder X-ray diffractometer (Cu Kα rad., λ = 1.54 Å) instrument. The Archimedes principle was used to determine the relative densities of the pellets.26 The DILOR-JOBIN-YVON-SPEX-623 nm was used to record the Raman spectra of the CDC pellets. XPS spectra of the CDC samples were recorded from a Thermo Fisher Scientific Instrument (Al Kα + hν = 1486.68 eV, spot size 400 μm). The total reflectance (spectral + diffused) spectra of the CDC samples were recorded using a JASCO spectrophotometer, V-77. The Bruker instrument (Nano GmbH Berlin, Germany) was used to record the FESEM images, EDX spectra and elemental mapping of the CDC samples. The a.c. impedance analysis was carried out in air at 1 atm using a CH Instrument (Inc CHI604D, USA). The CDC pellets were silver painted and baked at 773 K for 1 h for electrical connections. The Nyquist plots were obtained between the temperature ranges of 623–973 K and frequency range of 1–106 Hz with an a.c signal of 10 mA. The obtained data were fitted to an equivalent electrical circuit, [R(QR)(QR)] with ZSimpWin software. The conductivity was calculated using eqn (1):with thickness (t), area of cross-section (A), and R is the resistance.27
Results and discussion
Fig. 1 illustrates the XRD patterns of the pellet CDC samples. The XRD patterns of the powder CDC samples are shown in Fig. 1S as ESI.† The XRD patterns exhibited the same cubic-fluorite structure as per the space group Fm
m. The planes of the preferential peaks were indexed as per the ICDD card no. 34-0394. It was observed that no peaks of CaO or other impure phases were detected in all XRD patterns of the powder and pellet CDC samples. The XRD analysis revealed that the samples consist of a characteristic lattice structure that matches the standard CeO2.28,29 A trivial expansion in the lattice constant upon doping has been attributed to the substitution of the Ce ion by Ca, which caused strain and a slight deformation of the structure. To hold the charge balance, the initiated oxygen vacancies in the cerium lattice will extend the unit cell to limit the strain.30,31 Likewise, expansion of the lattice constant changes the d-spacing, causing a shift of the diffraction peaks to bring down the 2θ positions. Hence, the lattice parameters of the CDC samples increase with the dopant (Ca) concentration, as shown in Table 1. Furthermore, the high-temperature sintering of the pellets (1473 K) results in sharp peaks of increased intensity with large particle sizes, indicative of the highly crystallinity due to the grain growth.32,33
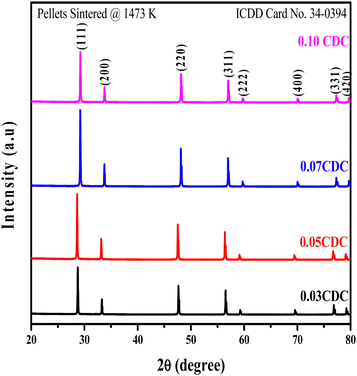 |
| Fig. 1 XRD pattern of CDC pellets sintered at 1473 K. | |
Table 1 Structural parameters of CDC pellets sintered at 1473 K
Samples |
Average crystallite size (D) (nm) Scherrer |
Lattice constant (a = b = c) (Å) |
Volume (Å)3 |
Dislocation density (δ) (line per m2 × 10−15) |
Specific surface area (m2 g−1) |
Bandgap, Eg (eV) |
Relative density (%) |
0.03 CDC |
67.7 |
5.413 |
158.6 |
0.218 |
0.128 |
2.98 |
94.97 |
0.05 CDC |
84.7 |
5.414 |
158.6 |
0.139 |
0.981 |
2.88 |
96.78 |
0.07 CDC |
86.1 |
5.418 |
159.1 |
0.134 |
0.968 |
2.82 |
95.12 |
0.10 CDC |
93.8 |
5.419 |
159.3 |
0.113 |
0.885 |
2.80 |
95.58 |
The Scherrer equation was used to determine the crystallite size from the width of the preferential planes. The dislocation density was found to increase with dopant content.
The lower values of dislocation density observed in the CDC pellets are likely due to the excellent crystallinity of the CDC samples.34 The computed theoretical surface area of the CDC samples appreciably varied with the dopant content and dislocation density.35 The average crystallite size, dislocation density and specific surface area values are summarized in Table 1. The relative density of the CDC pellets was found to be around 95% and is listed in Table 1. The relative density of the CDC pellets shows an increasing trend with dopant concentrations.
Fig. 2 shows the Raman spectra of the CDC pellets. The presence of a distinct intense peak at 464–461 cm−1 has been credited to the F2g Raman active mode of the fluorite structure. This is because of the symmetrical breathing mode of the Ce–O8 vibration unit.36–38 Furthermore, the Raman active mode F2g shifts to a lower frequency amid a rise in the dopant (Ca) content. The adjustment of the bond length, cross-section dispersion, and nuclear geometry of CeO2, which is the result of Ce–O bond breaking, emerged because of the generation of oxygen vacancies during the replacement of Ca-ions into the cerium lattice. The produced oxygen vacancies are facilitated with Ce and Ca ions, and decrease to change the length of the Ce–O and Ca–O bonds, and determine the moving of the F2g mode to bring down the frequencies. In Ca-doped CeO2, the F2g Raman-active mode showed more extensive and asymmetric peaks in contrast to the unadulterated CeO2. This asymmetric nature and expansion in the FWHM upsides of the F2g mode result from the improved lattice strain after doping, prompting the production of an excessive amount of oxygen vacancies.39–42 Furthermore, no other peaks are detected for impurities (like CaO), which in corroboration with the XRD study, established the formation of single-phase solid solutions.
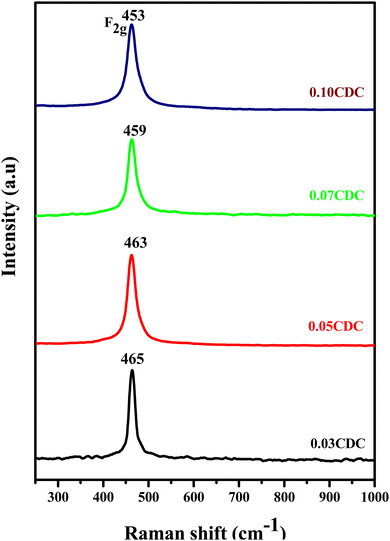 |
| Fig. 2 Raman spectra of the CDC pellets. | |
The XPS analysis was employed to study the microstructure and chemical state of the CDC samples. Fig. 3(a)–(c) shows the typical XPS spectra of the 0.05 CDC, 0.07 CDC and 0.10 CDC samples sintered at 1473 K. The presence of three different sections at around 880–890 eV, 895–910 eV, and at 916 eV of the Ce 3d core-level spectra is evident in all CDC samples.43 The existence of the Ce4+ state consigned to the 4f0 orbital transition is designated by the peak at 916 eV.44 The charge transfer from the oxygen 2p to Ce 4f states could resulted in the three doublets having configurations of 3d 4f0, 3d4f1 (L), and 3d 4f2 (L)2.45 The Ce 3d spectra of these samples displayed the six characteristic peaks in the binding energy (BE) range of 870 to 930 eV. The Ce 3d XPS spectrum consists of two spin–orbit splitting of multiplets of 3d5/2 and 3d3/2 core holes. The final state of 4f0–O 2p6 was represented by u′′′ and v′′′, and corresponds to high binding energy peaks. The u′′, v′′, u and v represent the 4f1–O 2p5 and 4f2–O 2p4 states and correspond to lower binding energy peaks.46 The binding energy peaks observed for the 3d5/2 core hole multiplets (0.05 CDC: 883.3, 889, 898), (0.07 CDC: 883.2, 888.8, 898 eV) and (0.10 CDC: 882.4, 887.5, 897 eV) are nearly in the same range, and a marginal decrease in the binding energy for V′′′ has been observed in all samples. The decreasing trend may be attributed to the increasing content of the dopant. Similarly, the binding energy peaks observed for the 3d3/2 core hole multiplets (0.05 CDC: 900.5, 907.6, 916.2), (0.07 CDC: 900.2, 907.6, 917 eV) and (0.10 CDC: 899.4, 906.7, 915.17 eV) are in good agreement with those that have been reported. The Ce 3d XPS spectra consist of some indistinguishable overlapped peaks corresponding to both Ce4+ and Ce3+ states, which make the spectra more complex. Most of the peaks in all of the spectra correspond to the Ce4+ state, and the BE peaks at 889, 888.8 and 887.8 eV of 3d5/2 and 900.5, 900.2 and 899.4 eV of 3d3/2 appeared for the 0.05 CDC, 0.07 CDC and 0.10 CDC samples, correspond to the Ce3+ state respectively.
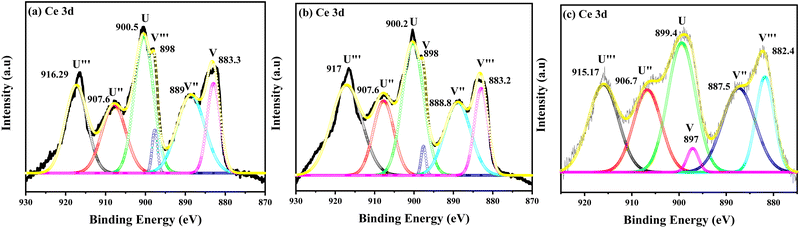 |
| Fig. 3 Ce 3d level experimental and peak fitting XPS spectra of (a) 0.05 CDC, (b) 0.07 CDC, and (c) 0.10 CDC. | |
The XPS spectra of the Ca 2p core-level with deconvolution peaks are shown in Fig. 4(a)–(c) of the CDC samples, and display the 2p1/2 and 2p3/2 states, which are split into two final states, I and II. Due to the spin–orbit splitting, these are distinguishable at around 347.2, 347.2 and 347.1 eV, corresponding to the 2p3/2 state, and 350.3, 350.2 and 352 eV, corresponding to the 2p1/2 state of the 0.05 CDC, 0.07 CDC and 0.10 CDC samples respectively.47,48
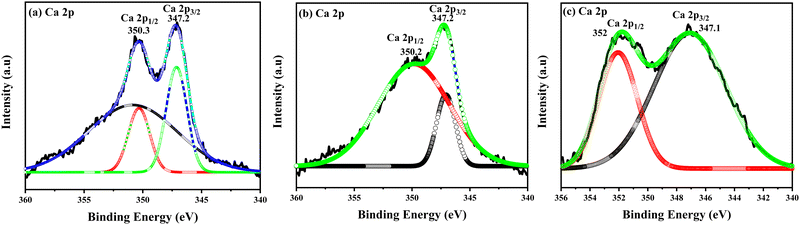 |
| Fig. 4 Ca 2p level experimental and peak fitting XPS spectra of (a) 0.05 CDC, (b) 0.07 CDC, and (c) 0.10 CDC. | |
Fig. 5(a)–(c) show the O1s XPS spectra deconvoluted into the different symmetrical signals of the CDC samples. The O 1s spectra show two different peaks at 528.5, 528.8, and 528.9 eV, and at 531.7, 531.3, and 531 eV for the 0.05, 0.07 and 0.010 CDC, respectively. The sharp intense peaks were attributed to the lattice oxygen of the CDC samples, and the other peaks are assigned to the existence of hydroxyl groups due to exterior contaminations on the sample. The high content of Ca in 0.10 CDC may have shifted the binding energy peak, as observed in Fig. 5(c).49
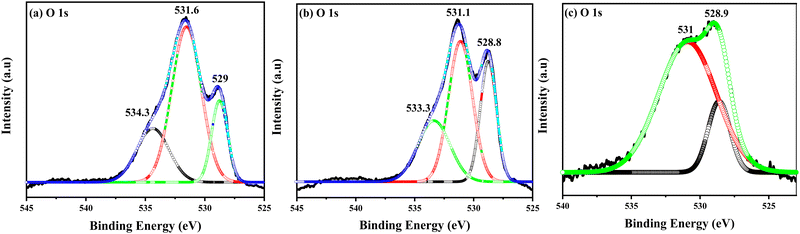 |
| Fig. 5 O 1 s level experimental and peak fitting XPS spectra of (a) 0.05 CDC, (b) 0.07 CDC, and (c) 0.10 CDC. | |
The 1s oxygen spectra were deconvoluted into contributions from the Ca–O, Ce3+–O, and Ce4+–O bound species because the cerium atom in the +3 oxidation state would be more tightly bound than cerium in the +4 state.50 Upon examining the peaks' relative area, a portion of the CeOx exists in the Ce3+ state.51 The obtained XPS spectra of the CDC samples show apparent CeO2 features and the existence of a Ca 2p signal, indicating that the Ca particles have been doped into the CeO2 lattice. This is corroborated by the XRD and Raman analyses of the CDC samples, and matches well with results reported in the literature.52
The diffuse reflectance (DR) UV-visible absorption spectra of the CDC samples are depicted in Fig. 6. The appearance of sharp intense peaks in the ultraviolet absorption region around 290–295 nm is ascribed to the charge transition from the O 2p to Ce 4f, and is assumed to be caused by the oxygen vacancies.53 This red-shift is attributed to the interfacial polaron effect emerging from the electron–phonon interaction.54 The Kubelka–Munk plots of the CDC samples for the optical band gap are shown in Fig. 4S (ESI†). The obtained band gap energy values are summarized in Table 1.
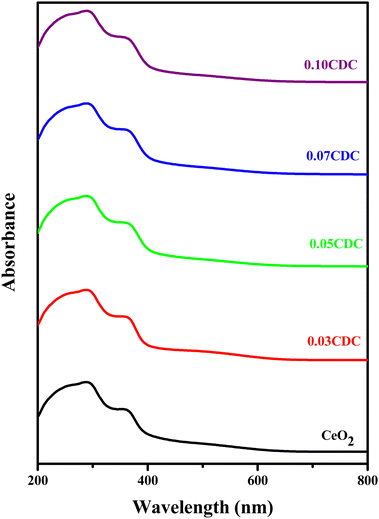 |
| Fig. 6 UV-Visible diffuse reflectance spectra of the CDC samples. | |
The FESEM images of the CDC pellets are shown in Fig. 7. Some particles were seen on the surface. This may be due to the powder, which is used as a bed during the sintering process. Generally, high-temperature sintering yields a larger particle size.55 All CDC samples have been observed to be highly dense with the absence of intra-granular pores. Hence, it may be concluded that these samples achieved good densification. The EDX and elemental mapping of the CDC samples are shown in Fig. 5S (ESI†), and it confirmed the existence of Ce, Ca, and O elements. The EDX analysis revealed that the elemental composition of all CDC samples are in good agreement with those with the proposed stoichiometric amounts. The EDX elemental mapping analysis revealed that the amount of dispersion for Ca was low compared to Ce, and confirmed the uniform distribution of elements throughout the matrix.56
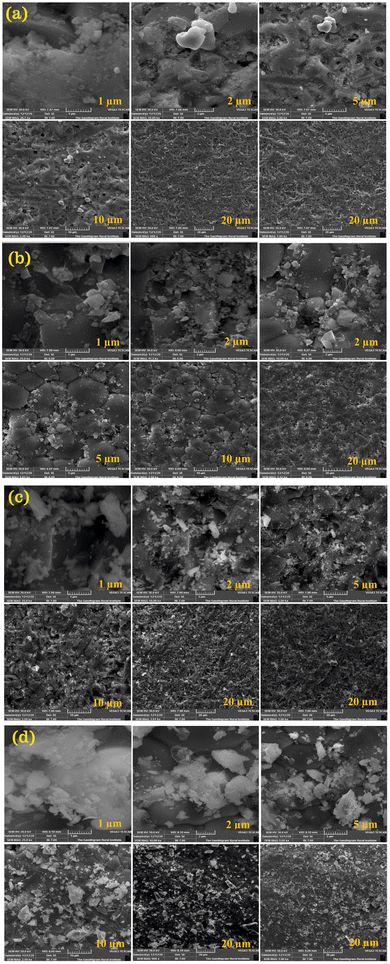 |
| Fig. 7 FE-SEM images of (a) 0.03 CDC, (b) 0.05 CDC, (c) 0.07 CDC, and (d) 0.1 CDC. | |
Fig. 8–11 show the Nyquist plots of the 0.03 CDC, 0.05 CDC, 0.07 CDC, and 0.10 CDC samples, respectively. To distinguish the overlapped grain, grain boundary (GB), and electrode contributions, an equivalent electrical circuit model [R(QR)(QR)] was used, as shown in the inset of Fig. 8. This comparable circuit comprises two RC components in series for the grain resistance (Rg) and GB (Rgb), and others for the electrode polarization (Re). A constant phase element (CPE = Q) is applied rather than a capacitor. This Q is comparable to the dispersion of the capacitor in parallel. The component Rg, Rgb‖Q represents the ionic conductivity through the grain and GB, respectively.57
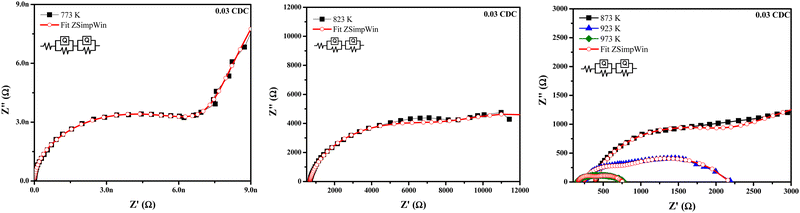 |
| Fig. 8 Nyquist plots of 0.03 CDC measured at temperatures between 773–973 K. | |
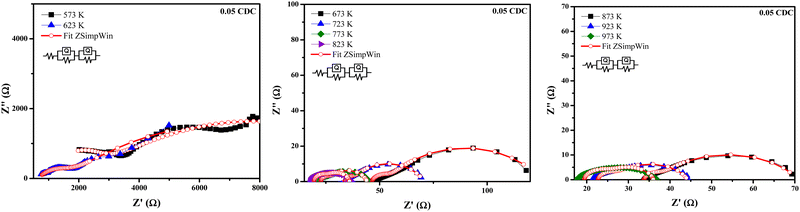 |
| Fig. 9 Nyquist plots of 0.05 CDC measured at temperatures between 573–973 K. | |
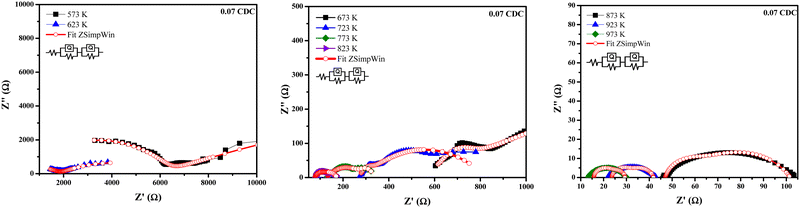 |
| Fig. 10 Nyquist plots of 0.07 CDC measured between temperatures between 573–973 K. | |
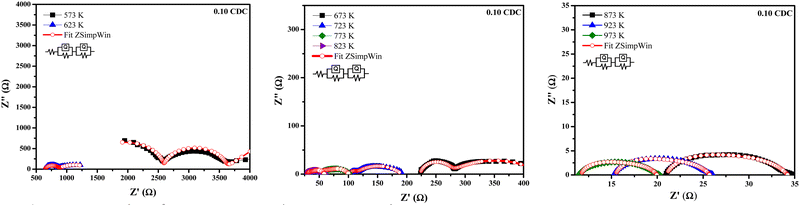 |
| Fig. 11 Nyquist plots of 0.07 CDC measured at temperatures between 573–973 K. | |
The total resistance is calculated by using the equation:58
where
Rg and
Rgb are the grain and GB resistance, respectively.
Impedance analysis revealed that the grain and GB conductivity are a function of the temperature rise for all CDC samples. The high and intermediate frequency ranges of the CDC samples for the grain and GB contributions exhibited a rapid ion migration process. The impedance data obtained from the ZSimpWin software confirmed the ionic conduction in all CDC solid solutions. The oxygen ion vacancies on the surface of the CDC are responsible for the ionic conduction. The lower grain resistance (Rg) values at higher temperatures show that all CDC samples have better ionic conductivity.16 The impedance parameters of all CDC samples extracted using the ZSimpWin software are shown in Tables 2–5. The total oxide ion conductivity is estimated by utilizing eqn (1) and is shown in Table 6. The obtained capacitance values in the range of 10−12–10−8 F indicated the grain and GB conduction process.59–61
Table 2 The EIS fitted data of the 0.03 CDC at different temperatures, 773–973 K
Temp. (K) |
R
g (Ω) |
R
gb (Ω) |
R
e (Ω) |
Chi-squared |
773 |
67 760 |
37 810 |
1247 |
1.207 × 10−1 |
823 |
6884 |
10 720 |
667.5 |
9.354× 10−4 |
873 |
5546 |
1440.0 |
383.1 |
3.241× 10−3 |
923 |
1577 |
371.40 |
233.5 |
1.276× 10−3 |
973 |
439.9 |
171.30 |
157.8 |
1.285 × 10−3 |
Table 3 The EIS fitted data of the 0.05 CDC at different temperatures, 573–973 K
Temp. (K) |
R
g (Ω) |
R
gb (Ω) |
R
e (Ω) |
Chi-squared |
573 |
11 200 |
1309 |
915.0 |
1.043 × 10−3 |
623 |
10 460 |
983.3 |
653.5 |
6.416 × 10−4 |
673 |
160.9 |
488.2 |
297.6 |
7.340 × 10−4 |
723 |
146.2 |
327.9 |
275.1 |
3.292 × 10−4 |
773 |
59.28 |
278.8 |
91.77 |
1.069 × 10−3 |
823 |
7.242 |
72.77 |
46.26 |
6.226 × 10−3 |
873 |
3.518 |
33.53 |
33.73 |
1.398 × 10−2 |
923 |
17.59 |
3.943 |
22.67 |
3.286 × 10−2 |
973 |
10.66 |
5.888 |
19.44 |
4.480 × 10−2 |
Table 4 The EIS fitted data of the 0.07 CDC at different temperatures, 573–973 K
Temp. (K) |
R
g (Ω) |
R
gb (Ω) |
Q–n (n) |
Chi-squared |
573 |
3229 |
5796 |
0.7399 |
7.710 × 10−4 |
623 |
1823 |
4664 |
0.3423 |
2.662 × 10−4 |
673 |
69.21 |
2981 |
0.2269 |
1.705 × 10−4 |
723 |
537.5 |
26.02 |
1 |
6.719 × 10−4 |
773 |
164.9 |
45.55 |
0.8398 |
4.398 × 10−3 |
823 |
58.45 |
38.01 |
0.6798 |
1.498 × 10−3 |
873 |
41.38 |
13.36 |
0.9396 |
6.413 × 10−3 |
923 |
7.665 |
10.57 |
0.7859 |
3.286 × 10−2 |
973 |
7.734 |
10.49 |
0.8609 |
2.675 × 10−2 |
Table 5 The EIS fitted data of the 0.10 CDC at different temperatures, 573–973 K
Temp. (K) |
R
g (Ω) |
R
gb (Ω) |
R
e (Ω) |
Chi-squared |
573 |
1354 |
2528 |
778.8 |
1.332 × 10−3 |
623 |
541.3 |
209.4 |
647.0 |
1.412 × 10−4 |
673 |
47.24 |
164.3 |
221.9 |
7.690 × 10−5 |
723 |
80.73 |
8.665 |
102.0 |
8.563 × 10−4 |
773 |
36.17 |
13.02 |
51.57 |
4.881 × 10−3 |
823 |
7.804 |
20.51 |
30.29 |
1.878 × 10−2 |
873 |
8.115 |
11.46 |
19.01 |
4.648 × 10−2 |
923 |
8.461 |
5.119 |
15.77 |
6.557 × 10−2 |
973 |
5.611 |
1.23 |
13.33 |
1.220 × 10−1 |
Table 6 Total oxide ion conductivity for the CDC samples
|
Conductivity, σt (S cm−1) |
Temp. (K) |
0.03 CDC |
0.05 CDC |
0.07 CDC |
0.10 CDC |
573 |
— |
7.62 × 10−6 |
1.34 × 10−6 |
1.41 × 10−5 |
623 |
— |
8.33 × 10−6 |
7.91 × 10−6 |
7.29 × 10−5 |
673 |
3.84 × 10−8 |
1.46 × 10−4 |
1.68 × 10−5 |
2.58 × 10−4 |
723 |
1.88 × 10−7 |
1.50 × 10−4 |
9.10 × 10−5 |
6.12 × 10−4 |
773 |
9.64 × 10−7 |
2.81 × 10−4 |
2.43 × 10−4 |
1.11 × 10−3 |
823 |
5.78 × 10−6 |
1.11 × 10−3 |
5.31 × 10−4 |
1.92 × 10−3 |
873 |
1.45 × 10−5 |
2.57 × 10−3 |
9.36 × 10−4 |
2.79 × 10−3 |
923 |
5.22 × 10−5 |
3.38 × 10−3 |
2.81 × 10−3 |
4.03 × 10−3 |
973 |
1.66 × 10−4 |
4.42 × 10−3 |
5.76 × 10−3 |
8.01 × 10−3 |
The effect of temperature on the oxide ion conductivity was determined by using the Arrhenius relation.
|  | (3) |
where
σ,
Ao,
T,
Ea, and
K are the conductivity, pre-exponential constant, temperature, activation energy, and Boltzmann constant, respectively. The Arrhenius plots of the total, grain and GB conductivity of the CDC samples are shown in
Fig. 12, and the determined activation energies are shown in
Table 7. It has been observed that the activation energy decreases with a rise in the dopant content. The grain conductivity contribution is a major factor for the total oxide ion conductivity in all CDC samples.
62 Thus, the amount of calcium doping affects the conductivity of the ceria. With the ascent in the dopant (Ca) content, a huge expansion in the grain conductivity was seen because of the improvement of the charge transporters emerging from the creation of oxygen vacancies as a result of doping. Hence, this prompted the expansion of the crystal structure.
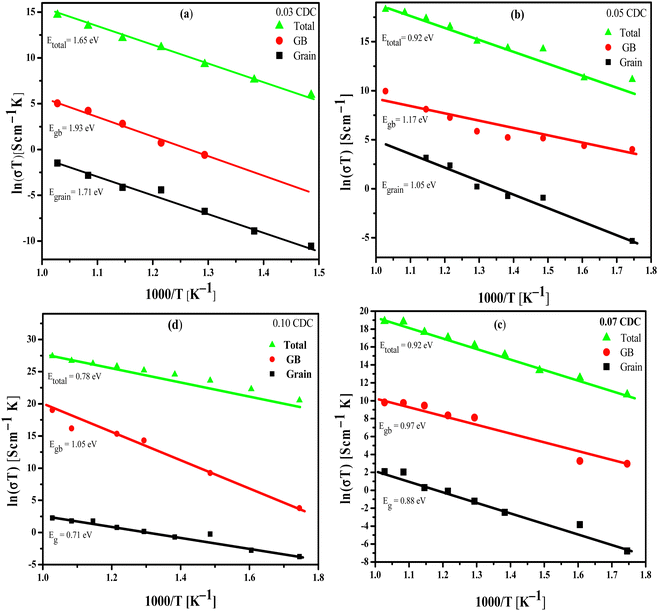 |
| Fig. 12 Arrhenius plots of the total, grain boundary and grain conductivity for (a) 0.03 CDC, (b) 0.05 CDC, (c) 0.07 CDC and (d) 0.10 CDC. | |
Table 7 The activation energy of the CDC between the temperatures, 573–973 K
Sample |
Activation energy, Ea (eV) |
Grain |
GB |
Total |
0.03 CDC |
1.17 |
1.93 |
1.65 |
0.05 CDC |
1.05 |
1.17 |
1.01 |
0.07 CDC |
0.88 |
0.97 |
0.92 |
0.10 CDC |
0.71 |
1.05 |
0.78 |
The rearranged anion opening at the surface and connection with the electrode assumes a significant part in the improvement of the oxide ion conductivity. The change in the surface oxygen vacancies fundamentally adjusts the physical and chemical properties of the materials. The insertion of the Ca2+ dopant into the cerium lattice generates the negatively charged defect, and hence increases the anion vacancies. The effect of the Ca2+ doping in CeO2 is depicted by using Kröger–Vink notation as shown in eqn (4).
|  | (4) |
At elevated temperature, the surplus electrons and anion vacancies are in equilibrium with the gas phase, as shown in eqn (5).63 The surplus electrons may arise due to small Ce3+ polarons, which is in corroboration with the obtained XPS data and small conduction bandwidth.
| 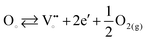 | (5) |
Thus, the improved mobility for the oxygen ions could lead to the high oxide ion conductivity for the CDC samples.64 Since calcium has likewise been viewed as a sintering aid for the ceria-based electrolytes, in this way it helps to achieve the high densification at a lower sintering temperature.65,66 Subsequently, with the ascent in the Ca content, the augmentation in the relative densities of these CDC samples has been clearly observed, as displayed in Table 1. The high densification of the CDC samples could facilitate the versatility of charge transporters at GB, and consequently it assists with improvement on the conductivity of the CDC. From the obtained results, it has been seen that the high oxide conductivities of 1.66 × 10−4, 4.42 × 10−3, 5.76 × 10−3, and 8.01 × 10−3 S cm−1 were recorded at 973 K with lower activation energies (Ea) of 1.65, 1.01, 0.92 and 0.78 eV for the 0.03 CDC, 0.05 CDC, 0.07 CDC, and 0.10 CDC, respectively.
The conductivity improves as a function of the dopant concentration. The 0.10 CDC shows the most noteworthy conductivity in the order of 8.01 × 10−3 S cm−1 at 973 K with a lower Ea of 0.78 eV, among all CDC samples studied here. The obtained conductivity values for the CDC were viewed as competent when contrasted with the ionic conductivity of 7.7 × 10−2 S cm−1 of Ce0.8Sm0.2O2−δ,67 3.6 × 10−2 S cm−1 of Ce0.9Gd0.1O2−δ,68 8.89 × 10−3 S cm−1 of Ce0.9La0.1O2−δ69 at 973 K, 5.9 × 10−3 S cm−1 of Ce0.9La0.1O2−δ70 and 9.4 × 10−4 S cm−1 of Ce0.85La0.15O2−δ at 873 K71 like potential electrolyte materials of IT – SOFCs. The obtained conductivity for 0.10 CDC in the order of 8.01 × 10−3 S cm−1 at 973 K with Ea of 0.78 eV can likewise be contrasted best with the conductivities of Ce0.9Ca0.1O2−δ (8.02 × 10−3, 6.31 × 10−3, 1.13 × 10−3 S cm−1 at 973 K with activation energies of 0.84, 0.86 and 0.89 eV) sample, synthesized from the combustion route using citric acid, glycine, and urea as a fuel, as reported by Ong et al.72 The high grain conductivity of the prepared CDC may be connected with the lower confinement of the dopant (Ca) at the GB.
Along these lines, bringing down the comparison space charge impacts could bring about a huge augmentation of oxide ion conductivity. In the current work, the 0.05, 0.07, and 0.10 CDC synthesized from the facile solid-state route showed the ionic conductivity in the order of 10−3 S cm−1 at 973 K. The lower Ea obtained for 0.1 CDC (0.78 eV) is nearly identical to the oxide ion conductivity of Ce0.9Ca0.1O1.9 synthesized from the auto – combustion route with citric acid, glycine and urea as fuels, as reported by Ong et al.58 Thus, the substantially more affordable Ca-doped ceria (CDC) materials have showed significant outcomes in contrast to those more costly rare-earth doped ceria-based materials, and could assist with decreasing the expense for SOFCs. Consequently, Ca-doped ceria-based ceramics could be viewed as potential electrolyte materials for IT-SOFC applications.
Conclusion
The Ca-doped ceria (CDC) samples having composition Ce(1−x)CaxO2−δ [x = 0.03–0.1] were successfully prepared by using a cost-effective and conventional solid – state method. All CDC were sintered at lower temperature of 1473 K than required. The XRD, Raman, XPS, and FESEM – EDX analysis confirmed the formation of the single-phase solid solution of these CDC. The 0.10 CDC showed an essentially upgraded oxide ion conductivity in the order of 8.01 × 10−3 S cm−1 at 973 K with a lower activation energy, Ea = 0.78 eV, in contrast to the other CDC. The critical improvement in the physical and electrochemical properties of the CDC through solid – state synthesis has been accomplished. Thus, monetarily reasonable CDC samples might be viewed as a potential electrolyte material for IT-SOFC applications.
Author contributions
Dr Naeemakhtar Momin: investigation, conceptualization, writing – original draft preparation, Prof. J. Manjanna: supervision, writing – review & editing, Prof. Satoru Kobayashi: resources, visualization, Dr Aruna S. T.: project administration, supervision, Dr Senthil Kumar S: formal analysis, validation, Dr G.P. Nayaka: resource.
Conflicts of interest
The authors declare that they have no known competing financial interests or personal relationships that could have appeared to influence the work reported in this paper.
Acknowledgements
The authors greatly acknowledge the financial support from (i) VGST-CESEM, GRD – 746, Ministry of IT, BT and S & T, Govt. of Karnataka; (ii) DST-FIST, Govt. of India [SR/FST/CSI-273/2016]; and (iii) Rani Channamma University, Belagavi – Interdisciplinary Research Project, 2022-23.
References
- J. B. Goodenough, Oxide-ion electrolytes, Annu. Rev. Mater. Res., 2003, 33, 91, DOI:10.1146/annurev.matsci.33.022802.091651.
- M. Mogensen, Physical, chemical and electrochemical properties of pure and doped ceria, Solid State Ionics, 2000, 129, 63, DOI:10.1016/S0167-2738(99)00318-5.
- T. Ahmad, M. Shahazad, M. Ubaidullah, J. Ahmed, A. Khan and A. M. El-Toni, Structural characterization and dielectric properties of ceria-titania nanocomposites in low ceria region, Mater. Res. Express, 2017, 4, 125016, DOI:10.1088/2053-1591/aa9c51.
- T. Zhang, J. Ma, L. Kong, S. Chan and J. Kilner, Aging behavior and ionic conductivity of ceria-based ceramics: A comparative study, Solid State Ionics, 2004, 170, 209, DOI:10.1016/j.ssi.2004.03.003.
- K. Eguchi, T. Setoguchi, T. Inoue and H. Arai, Electrical properties of ceria-based oxides and their application to solid oxide fuel cells, Solid State Ionics, 1992, 52, 165, DOI:10.1016/0167-2738(92)90102-U.
- Y. Zheng, H. Gu, H. Chen, L. Gao, X. Zhu and L. Guo, Effect of Sm and Mg co-doping on the properties of ceria-based electrolyte materials for IT-SOFCs, Mater. Res. Bull., 2009, 44, 775, DOI:10.1016/j.materresbull.2008.09.021.
- K. Yamashita, K. V. Ramanujachary and M. Greenblatt, Hydrothermal synthesis and low temperature conduction properties of substituted ceria ceramics, Solid State Ionics, 1995, 81, 53, DOI:10.1016/0167-2738(95)99031-H.
- S. Banerjee and P. S. Devi, Understanding the effect of calcium on the properties of ceria prepared by a mixed fuel process, Solid State Ionics, 2008, 179, 661, DOI:10.1016/j.ssi.2008.04.008.
- H. Yahiro, T. ohuchi, K. Eguchi and H. Arai, Electrical properties and microstructure in the system ceria-alkaline earth oxide, J. Mater. Sci., 1988, 23, 1036, DOI:10.1007/BF01154008.
- Y. Ma, X. Wang, H. A. Khalifa, B. Zhu and M. Muhammed, Enhanced ionic conductivity in calcium doped ceria – carbonate electrolyte: A composite effect, Int. J. Hydrogen Energy, 2012, 37, 19401, DOI:10.1016/j.ijhydene.2011.09.122.
- Y. Lin, S. Fang, D. Su, K. S. Brinkman and F. Chen, Enhancing grain boundary ionic conductivity in mixed ionic–electronic conductors, Nat. Commun., 2015, 6, 6824, DOI:10.1038/ncomms7824.
- J. Garcia-Barriocanal, A. Rivera-Calzada, M. Varela, Z. Sefrioui, E. Iborra, C. Leon, S. J. Pennycook and J. Santamaria, Colossal ionic conductivity at interfaces of epitaxial ZrO2:Y2O3/SrTiO3 heterostructures, Science, 2008, 321, 676, DOI:10.1126/science.1156393.
- B. Wang, Y. Cai, C. Xia, J.-S. Kim, Y. Liu, W. Dong, H. Wang, M. Afzal, J. Li, R. Raza and B. Zhu, Semiconductor-ionic membrane of LaSrCoFe-oxide-doped ceria solid oxide fuel cells, Electrochim. Acta, 2017, 248, 496, DOI:10.1016/j.electacta.2017.07.128.
- Y. Lu, J. Li, L. Ma, Z. Lu, L. Yu and Y. Cai, The development of semiconductor-ionic conductor composite electrolytes for fuel cells with symmetrical electrodes, Int. J. Hydrogen Energy, 2021, 46, 9835, DOI:10.1016/j.ijhydene.2020.05.240.
- Y. Lu, M. Akbar, J. Li, L. Ma, B. Wang and C. Xia, A p–n–n heterostructure composite for low-temperature solid oxide fuel cells, J. Alloys Compd., 2022, 890, 161765, DOI:10.1016/j.jallcom.2021.161765.
- M. A. K. Y. Shah, N. Mushtaq, S. Rauf, N. Akbar, Y. Xing, Y. Wu, B. Wang and B. Zhu, Advanced fuel cell based on semiconductor perovskite La–BaZrYO3−δ as an electrolyte material operating at low temperature 550 °C, Int. J. Hydrogen Energy, 2020, 45, 27501, DOI:10.1016/j.ijhydene.2020.07.011.
- L. Li, Q. Shi, L. Huang, C. Yan and Y. Wu, Green synthesis of faujasite-La0.6Sr0.4Co0.2Fe0.8O3−δ mineral nanocomposite membrane for low temperature advanced fuel cells, Int. J. Hydrogen Energy, 2021, 46, 9826, DOI:10.1016/j.ijhydene.2020.05.275.
- N. Akbar, S. Paydar and Y. Wu, Tuning an ionic-electronic mixed conductor NdBa0.5Sr0.5Co1.5Fe0.5O5+δ for electrolyte functions of advanced fuel cells, Int. J. Hydrogen Energy, 2021, 46, 9847, DOI:10.1016/j.ijhydene.2020.05.220.
- S. Paydar, N. Akbar, Q. Shi and Y. Wu, Developing cuprospinel CuFe2O4–ZnO semiconductor heterostructure as a proton conducting electrolyte for advanced fuel cells, Int. J. Hydrogen Energy, 2021, 9927, DOI:10.1016/j.ijhydene.2020.04.198.
- X. Chen, B. Dong, Q. A. Islam, H. Song and Y. Wu, Semiconductor-ionic properties and device performance of heterogeneous La-doped CeO2–ZnO nanocomposites, Int. J. Hydrogen Energy, 2021, 46, 9968, DOI:10.1016/j.ijhydene.2020.04.174.
- Q. Shi, J. Chen, Y. Xing, B. Zhu and Y. Wu, Semiconductor heterostructure SrTiO3/CeO2 electrolyte membrane fuel cells, J. Electrochem. Soc., 2020, 167, 054504, DOI:10.1149/2.0082005jes.
- Y. Xing, Y. Wu, L. Li, Q. Shi, J. Shi, S. Yun, M. Akbar, B. Wang, J. S. Kim and B. Zhu, Proton shuttles in CeO2/CeO2−δ core–shell structure, ACS Energy Lett., 2019, 4, 2601, DOI:10.1021/acsenergylett.9b01829.
- K. Yamashita, K. V. Ramanujachary and M. Greenblatt, Hydrothermal synthesis and low temperature conduction properties of substituted ceria ceramics, Solid State Ionics, 1995, 81, 53, DOI:10.1016/0167-2738(95)99031-H.
- Y. Dong, S. Hampshire, B. Lin, Y. Ling and X. Zhang, High sintering activity Cu–Gd co-doped CeO2 electrolyte for solid oxide fuel cells, J. Power Sources, 2010, 195, 6510, DOI:10.1016/j.jpowsour.2010.03.053.
- B. Zhu, X. Liu, M. Sun, S. Ji and J. Sun, Calcium doped ceria-based materials for cost-effective intermediate temperature solid oxide fuel cells, Solid State Sci., 2003, 5, 1127, DOI:10.1016/S1293-2558(03)00123-7.
- T. S. Zhang, J. Ma, H. T. Huang, P. Hing, Z. T. Xia, S. H. Chan and J. A. Kilner, Effects of dopant concentration and aging on the electrical properties of Y-doped ceria electrolytes, Solid State Sci., 2003, 5, 1505, DOI:10.1016/j.solidstatesciences.2003.10.001.
- M. G. Chourashiya and L. D. Jadhav, synthesis and characterization of 10% Gd doped ceria (GDC) deposited on NiO-GDC anode-grade-ceramic substrate as half cell for IT-SOFC, Int. J. Hydrogen Energy, 2011, 36, 14984, DOI:10.1016/j.ijhydene.2010.12.083.
- B. Feng, I. Sugiyama, H. Hojo, H. Ohta, N. Shibata and Y. Ikuhara, Atomic structures and oxygen dynamics of CeO2 grain boundaries, Sci. Rep., 2016, 6, 20288, DOI:10.1038/srep20288.
- W. H. Weber, K. C. Hass and J. R. McBride, Raman study of CeO2: Second-order scattering, lattice dynamics, and particle-size effects, Phys. Rev. B: Condens. Matter Mater. Phys., 1993, 48, 178, DOI:10.1103/PhysRevB.48.178.
- X. Liu, S. Chen and X. Wang, Synthesis and photoluminescence of CeO2:Eu3+ phosphor powders, J. Lumin., 2007, 127, 650, DOI:10.1016/j.jlumin.2007.03.014.
- S. Patil, S. Seal, Y. Guo, A. Schulte and J. Norwood, Role of trivalent La and Nd dopants in lattice distortion and oxygen vacancy generation in cerium oxide nanoparticles, Appl. Phys. Lett., 2006, 88, 243110, DOI:10.1063/1.2210795.
- M. Kurian and C. Kunjachan, Investigation of size dependency on lattice strain of nanoceria particles
synthesised by wet chemical methods, Int. Nano Lett., 2014, 4, 73, DOI:10.1007/s40089-014-0122-7.
- T. Ahmad and A. K. Ganguli, Reverse micellar route to nanocrystalline titanates (SrTiO3, Sr2TiO4, and PbTiO3): Structural aspects and dielectric properties, J. Am. Ceram. Soc., 2006, 89, 1326, DOI:10.1111/j.1551-2916.2005.00886.x.
- R. Tholkappiyan and K. Vishista, Combustion synthesis of Mg–Er ferrite nanoparticles: Cation distribution and structural, optical, and magnetic properties, Mater. Sci. Semicond. Process., 2015, 40, 631, DOI:10.1016/j.mssp.2015.06.076.
- A. K. Tripathi, M. C. Mathpal, P. Kumar, M. K. Singh, M. A. G. Soler and A. Agarwal, Structural, optical and photoconductivity of Sn and Mn doped TiO2 nanoparticles, J. Alloys Compd., 2015, 622, 37, DOI:10.1016/j.jallcom.2014.09.218.
- M. Zawadzki, Preparation and characterization of ceria nanoparticles by microwave-assisted solvothermal process, J. Alloys Compd., 2008, 454, 347, DOI:10.1016/j.jallcom.2006.12.078.
- T. Suzuki, I. Kosacki, H. U. Anderson and P. Colomban, Electrical conductivity and lattice defects in nanocrystalline cerium oxide thin films, J. Am. Ceram. Soc., 2004, 84, 2007, DOI:10.1111/j.1151-2916.2001.tb00950.x.
- R. Kostić, S. Aškrabić, Z. Dohčević-Mitrović and Z. V. Popović, Low-frequency Raman scattering from CeO2 nanoparticles, Appl. Phys. A: Mater. Sci. Process., 2008, 90, 679, DOI:10.1007/s00339-007-4345-6.
- J. E. Spanier, R. D. Robinson, F. Zhang, S.-W. Chan and I. P. Herman, Size-dependent properties of CeO2−y nanoparticles as studied by Raman scattering, Phys. Rev. B: Condens. Matter Mater. Phys., 2001, 64, 245407, DOI:10.1103/PhysRevB.64.245407.
- W. F. Zhang, Y. L. He, M. S. Zhang, Z. Yin and Q. Chen, Raman scattering study on anatase TiO2 nanocrystals, J. Phys. D: Appl. Phys., 2000, 33, 912, DOI:10.1088/0022-3727/33/8/305.
- H. C. Choi, Y. M. Jung and S. Bin Kim, Size effects in the Raman spectra of TiO2 nanoparticles, Vib. Spectrosc., 2005, 37, 33, DOI:10.1016/j.vibspec.2004.05.006.
- V. V. Pushkarev, V. I. Kovalchuk and J. L. D’Itri, Probing defect sites on the CeO2 surface with dioxygen, J. Phys. Chem. B, 2004, 108, 5341, DOI:10.1021/jp0311254.
- X. Garcia, L. Soler, N. J. Divins, X. Vendrell, I. Serrano, I. Lucentini, J. Prat, E. Solano, M. Tallarida, C. Escudero and J. Llorca, Ceria-based catalysts studied by near ambient pressure x-ray photoelectron spectroscopy: A review, Catalysts, 2020, 10, 286, DOI:10.3390/catal10030286.
- M. A. M. Khan, W. Khan, M. Ahamed and A. N. Alhazaa, Microstructural properties and enhanced photocatalytic performance of Zn doped CeO2 nanocrystals, Sci. Rep., 2017, 7, 1, DOI:10.1038/s41598-017-11074-7.
- S. A. Acharya, V. M. Gaikwad, S. W. D’Souza and S. R. Barman, Gd/Sm dopant-modified oxidation state and defect generation in nano-ceria, Solid State Ionics, 2014, 260, 21, DOI:10.1016/j.ssi.2014.03.008.
- P. Datta, P. Majewski and F. Aldinger, Study of gadolinia-doped ceria solid electrolyte surface by XPS, Mater. Charact., 2009, 60, 138–143, DOI:10.1016/j.matchar.2008.08.002.
- X. L. Zhao, J. J. Liu, T. Xiao, J. C. Wang, Y. X. Zhang, H. C. Yao, J. S. Wang and Z. J. Li, Effect of Ca co-dopant on the electrical conductivity of Gd-doped ceria, J. Electroceram., 2012, 28, 149, DOI:10.1007/s10832-012-9696-5.
- M. Yan, T. Mori, J. Zou, F. Ye, D. R. Ou and J. Drennan, TEM and XPS analysis of CaxCe1−xO2−y (x = 0.05–0.5) as electrolyte materials for solid oxide fuel cells, Acta Mater., 2009, 57, 722–731, DOI:10.1016/j.actamat.2008.10.014.
- J. L. M. Rupp, T. Drobek, A. Rossi and L. J. Gauckler, Chemical analysis of spray pyrolysis gadolinia-doped ceria electrolyte thin films for solid oxide fuel cells, Chem. Mater., 2007, 19, 1134, DOI:10.1021/cm061449f.
- A. Kossoy, H. Cohen, T. Bendikov, E. Wachtel and I. Lubomirsky, Water adsorption at the surface of pure and Gd-doped ceria, Solid State Ionics, 2011, 194, 1–4, DOI:10.1016/j.ssi.2011.05.011.
- S. A. Acharya, V. M. Gaikwad, S. W. D’Souza and S. R. Barman, Gd/Sm dopant-modified oxidation state and defect generation in nano-ceria, Solid State Ionics, 2014, 260, 21, DOI:10.1016/j.ssi.2014.03.008.
- P. Datta, P. Majewski and F. Aldinger, Study of gadolinia-doped ceria solid electrolyte surface by XPS, Mater. Charact., 2009, 60, 138, DOI:10.1016/j.matchar.2008.08.002.
- D. Manoharan and K. Vishista, Optical Properties of Nano-crystalline cerium dioxide synthesized by single step aqueous citrate-nitrate gel combustion method, Asian J. Chem., 2013, 25, 9045, DOI:10.14233/ajchem.2013.14984.
- K. Amarsingh Bhabu, J. Theerthagiri, J. Madhavan, T. Balu, G. Muralidharan and T. R. Rajasekaran, Cubic fluorite phase of samarium doped cerium oxide (CeO2)0.96Sm0.04 for solid oxide fuel cell electrolyte, J. Mater. Sci.: Mater. Electron., 2016, 27, 1566, DOI:10.1007/s10854-015-3925-z.
- V. Thangadurai and P. Kopp, Chemical synthesis of Ca-doped CeO2 intermediate temperature oxide ion electrolytes, J. Power Sources, 2007, 168, 178, DOI:10.1016/j.jpowsour.2007.03.030.
- P. Venkataswamy, D. Jampaiah, A. E. Kandjani, Y. M. Sabri, B. M. Reddy and M. Vithal, Transition (Mn, Fe) and rare earth (La, Pr) metal doped ceria solid solutions for high performance photocatalysis: Effect of metal doping on catalytic activity, Res. Chem. Intermed., 2018, 44, 2523, DOI:10.1007/s11164-017-3244-5.
- A. Ali, R. Raza, M. Kaleem Ullah, A. Rafique, B. Wang and B. Zhu, Alkaline earth metal and samarium co-doped ceria as efficient electrolytes, Appl. Phys. Lett., 2018, 112, 043902, DOI:10.1063/1.5005824.
- K. C. Anjaneya, G. P. Nayaka, J. Manjanna, G. Govindaraj and K. N. Ganesha, Preparation and characterization of Ce1−xGdxO2−δ (x = 0.1–0.3) as solid electrolyte for intermediate temperature SOFC, J. Alloys Compd., 2013, 578, 53, DOI:10.1016/j.jallcom.2013.05.010.
- M. V. Ganduglia-Pirovano, A. Hofmann and J. Sauer, Oxygen vacancies in transition metal and rare earth oxides: Current state of understanding and remaining challenges, Surf. Sci. Rep., 2007, 62, 219, DOI:10.1016/j.surfrep.2007.03.002.
- M. F. García-sánchez, J. M. Peko, A. R. Ruiz-salvador, G. Rodríguez-gattorno and Y. Echevarría, An elementary picture of dielectric spectroscopy in solids: Physical basis, J. Chem. Educ., 2003, 80, 1062–1063, DOI:10.1021/ed080p1062.
- N. Momin, J. Manjanna, K. S. Rane, A. Kumar, S. Senthilkumar and S. T. Aruna, Structural and ionic conductivity of Cu-doped titania (Ti0.95Cu0.05O2−δ) for high temperature energy devices, Ceram. Int., 2021, 47, 10284, DOI:10.1016/j.ceramint.2020.06.277.
- K. Zhao and Y. Du, Calcium-doped ceria materials for anode of solid oxide fuel cells running on methane fuel, J. Power Sources, 2017, 347, 79, DOI:10.1016/j.jpowsour.2017.01.113.
- J. Maier, Electronic transport in single crystals of Gd-doped ceria, J. Electrochem. Soc., 2007, 156, 583–587, DOI:10.1149/1.2722530.
- H. Song and U. S. Ozkan, Changing the oxygen mobility in Co/ceria catalysts by ca incorporation: Implications for ethanol steam reforming, J. Phys. Chem. A, 2010, 114, 3796, DOI:10.1021/jp905608e.
- S. Banerjee, P. S. Devi, D. Topwal, S. Mandal and K. Menon, Enhanced ionic conductivity in Ce0.8Sm0.2O1.9 unique effect of calcium co-doping, Adv. Funct. Mater., 2007, 17, 2847, DOI:10.1002/adfm.200600890.
- N. Momin and J. Manjanna, Ionic conductivity of Ce0.91Ca0.09O2−δ as an electrolyte for intermediate temperature solid oxide fuel cells, Res. J. Chem. Environ., 2021, 25(12) DOI:10.25303/2512rjce001009.
- N. Jaiswal, D. Kumar, S. Upadhyay and O. Parkash, Ceria co-doped with calcium (Ca) and strontium (Sr): A potential candidate as a solid electrolyte for intermediate temperature solid oxide fuel cells, Ionics, 2014, 20, 45, DOI:10.1007/s11581-013-0936-8.
- A. Z. Liu, J. X. Wang, C. R. He, H. Miao, Y. Zhang and W. G. Wang, Synthesis and characterization of Gd0.1Ce0.9O1.95 nanopowder via an acetic-acrylicmethod, Ceram. Int., 2013, 39, 6229, DOI:10.1016/j.ceramint.2013.01.044.
- N. Momin, J. Manjanna, L. D’Souza, S. T. Aruna and S. Senthil Kumar, Synthesis, structure and ionic conductivity of nanocrystalline Ce1−xLaxO2−δ as an electrolyte for intermediate temperature solid oxide fuel cells, J. Alloys Compd., 2022, 896, 163012, DOI:10.1016/j.jallcom.2021.163012.
- S. Dikmen, P. Shuk and M. Greenblatt, Hydrothermal synthesis and properties of Ce1−xLaxO2−δ solid solutions, Solid State Ionics, 1999, 126, 89, DOI:10.1016/S0167-2738(99)00146-0.
- N. Jaiswal, S. Upadhyay, D. Kumar and O. Parkash, Ionic conductivity investigation in lanthanum (La) and strontium (Sr) co-doped ceria system, J. Power Sources, 2013, 222, 230, DOI:10.1016/j.jpowsour.2012.08.095.
- P. S. Ong, Y. P. Tan, Y. H. Taufiq-Yap and Z. Zainal, Improved sinterability and conductivity enhancement of 10 mol% calcium-doped ceria using different fuel-aided combustion reactions and its structural characterisation, Mater. Sci. Eng., B, 2014, 185, 26, DOI:10.1016/j.mseb.2014.01.016.
|
This journal is © The Royal Society of Chemistry 2022 |
Click here to see how this site uses Cookies. View our privacy policy here.