DOI:
10.1039/D1MA00929J
(Review Article)
Mater. Adv., 2022,
3, 1874-1904
Microfluidic sensors based on two-dimensional materials for chemical and biological assessments
Received
7th October 2021
, Accepted 11th January 2022
First published on 12th January 2022
Abstract
There has been an exponential increase in the number of studies of two-dimensional (2D) layered materials for sensing applications since the isolation of graphene in 2004. These materials serve as a supporting substrate and an active sensing element in a variety of health care and environmental applications due to their exceptional and frequently tunable physical, optical, electrical, and electrochemical capabilities. Microfluidic sensors are a relatively new technology that shows promise in health care and environmental applications due to quick response, inexpensive and highly sensitive analysis with high yield. Due to their appealing electrical and electrochemical properties with a variety of structural features and fabrication processes, 2D materials are a good contender for microfluidic sensor applications. As a result, these two-dimensional material-based microfluidic devices can provide a well-controlled microenvironment for both low-cost point-of-care analytics and advanced chemical/biological analysis. Different 2D materials for microfluidic sensors and their possible use in various health care and environmental applications including wearable sensors are critically addressed in this review. Finally, the challenges and prospects for the future of 2D materials in terms of commercial challenges and long-term sustainability are examined.
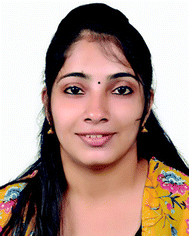
Sithara Radhakrishnan
| Ms Sithara Radhakrishnan received her BTech in Polymer engineering from Mahatma Gandhi University (Kerala, India) in 2016 and MTech in Polymer engineering from Cochin University of Science and Technology (Kerala, India) in 2018. She is currently a Nanoscience doctoral student at the Centre for Nano & Material Science, Jain University, working under Prof. Dr Chandra Sekhar Rout. Her current research focuses on designing and fabrication of 2D material nanocomposite for energy storage applications. |
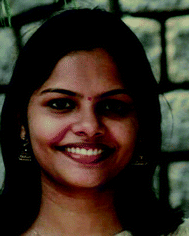
Minu Mathew
| Ms Minu Mathew is a JRF in Chemistry at the Centre for Nano and Material Science (CNMS), Jain University, Bangalore under the supervision of Prof. Chandra Sekhar Rout. She received her MPhil in Chemistry from the Christ University, Bangalore in 2019. She completed her Master's (2014) and Bachelor's (2012) degree in Chemistry from the Mahatma Gandhi University, Kottayam, Kerala, India. Her research interests include synthesis and characterization of two dimensional (2D) materials for energy and environment related applications. |
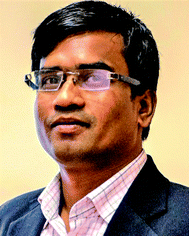
Chandra Sekhar Rout
| Prof. Chandra Sekhar Rout is a full Professor at Centre for Nano & Material Sciences (CNMS), Jain University. Before joining CNMS, He was a DST-Ramanujan Fellow at IIT Bhubaneswar, India (2013–2017). He received his BSc (2001) and MSc (2003) degrees from Utkal University and his PhD from JNCASR, Bangalore (2008) under the supervision of Prof. C. N. R. Rao, Bharat Ratna. He did his postdoctoral research at National University of Singapore (2008–2009), Purdue University, USA (2010–2012) and UNIST, South Korea (2012–2013). He was awarded the prestigious Ramanujan Fellowship and Young Scientist award of Department of Science and Technology, Govt. of India in 2013, emerging investigator award 2017 from Elsevier, IAAM medal 2017 from International Association of Advanced Materials 2017, Young researcher award from Venus International Foundation in 2015. His research interests include preparation and characterization of 2D layered materials and its hybrids for chemical sensors and biosensors, supercapacitors and energy storage devices, field emitters and electronic devices. He has authored more than 180 research papers in international journals and edited 3 books. His current h-index is 44 with total citations ∼9000. He was ranked top 2% scientists in India by the Stanford study in 2019 and 2020. He serves as board members of various reputed journals and is associate editor of “RSC Advances” a journal of Royal Society of Chemistry and “American Journal of Engineering and Applied Sciences” of Science Publications. He has completed several sponsored projects funded by government of India and supervised PhD, postdocs and MSc students. He has delivered more than fifty invited talks in various national and international conferences and travelled to several countries such as USA, UK, Israel, Singapore, South Korea, Brazil and Russia etc. for collaboration purposes. |
1. Introduction
Sensors are electrical devices that typically offer concurrent information on the system's composition by connecting the recognition element to a transducer.1 A perfect sensor would have the highest possible throughput, user-friendly selectivity, and sensitivity, capable of in-field operation, and affordable in cost. It should also meet the world health organization's standards for portable sensors.2 Microfluidic devices, from these perspectives, are fascinating technologies for accomplishing Lab-on-a-Chip (LOC)-based point-of-care applications.3 Sensors employed in a microfluidic platform are referred to as “Microfluidic Sensors.” Microfluidics is an entirely new field that manipulates fluids in channels as small as tens of micrometers in diameter.4 The key advantages of these microfluidic systems include regulated liquid flow, minimal reagent and sample use, rapid analysis, system compactness and parallelization, reduced waste generation, along disposability.5,6 Previously, microfluidics was primarily concerned with incorporating microsensors with fluidic elements (pumps, actuators and valves, so on) and the miniaturization of analytical assays. Micro-fabricated structures were then used to create Micro Total Analysis Systems (μTAS) and LOC. The significant difference between these two was explained by Gupta et al., where μTAS refers to integrating the entire sequence of lab procedures to perform chemical analysis. In contrast, LOC refers to integrating one or more lab processes onto a single chip.7
A range of life science areas, including cell biology, genetic studies, and protein analysis, have benefited from the miniaturization of these microfluidic devices.8 Current research is focused on the creation of sensors for microscale measurement. These devices are now primarily used in various fields of study, including biology, biomedical sciences, chemistry, and engineering.9,10 Microfluidic devices are not only small but may also be used as a platform for analysis systems. Combining the extraordinary features of nanomaterials with microfluidic sensors improves selectivity and sensitivity and has stimulated interest in a broad range of applications. Nanomaterials have a lot of potential in the field because of their biocompatibility, catalytic efficiency, quantum size effect, electrical conductivity, Raman spectrum effect, reactivity, surface and interface effects, and other features of microfluidic sensors.11,12 Nanomaterials can be used to support nanostructure electrodes or act as signal enhancers, labels, and label supports in microfluidic sensors.10 Among these gold and platinum nanoparticles are frequently used as electrodes in microfluidic sensors. Main drawbacks of metal nanoparticles are their small potential window and fouling/passivation produced by contact with materials. Compared to metal nanoparticles, carbon nanomaterials-based electrodes are more stable over a wide potential range and less prone to fouling.13 Carbon nanomaterials, including carbon nanohorns (CNHs), carbon nanotubes (CNT) and carbon nanospheres (CNSs), have already been proven tremendous success in microfluidic sensors.10 Among these CNTs have received the most significant attention due to their exceptional mechanical and electrochemical properties and high thermal and electrical conductivity. However still, their one-dimensional (1D) nature poses a significant problem during fabrication.14 Alternatively. graphene derivatives are gaining popularity among these carbon nanomaterials due to the higher adhesion offered by its flat 2D shape and greater availability of active sites.10 Graphene's unique and exotic features include its ultra-light honey comb structure with a planar density of roughly 0.77 mg m−2 and super thinness (0.35 nm). Because of these features, graphene has piqued the interest of many researchers in the manufacture of microfluidic sensors, and its applications have already been addressed by Chen et al., Sengupta et al., and others.5,15 Inspired by these graphene-based microfluidic sensors in 2015, Yang et al., for the very first time, fabricated a microfluidic sensor using MoS2 for fluorescent DNA detection.16 MXene, a novel material recently added to the 2D material family, may also help overcome existing microfluidic sensors issues. This material, which has excellent biocompatibility as well as outstanding electrical conductivity, is ideal for microfluidic sensors.17 The importance of all these 2D materials in microfluidic sensors is shown in Fig. 1.
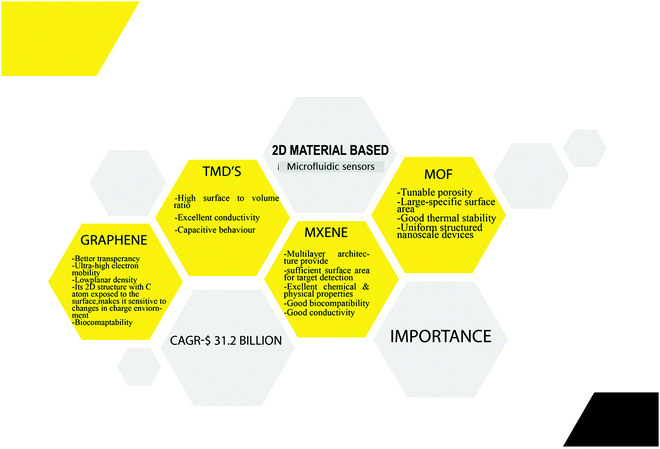 |
| Fig. 1 Importance of 2D materials in microfluidic sensors. | |
Biosensors are advancing due to breakthroughs advancements in manufacturing processes and device integration; according to Frost & Sullivan, the global biosensor industry is poised to increase at a compound annual growth rate (CAGR)of 12 percent from $17.7 billion in 2018 to $31.2 billion by the end of 2023, up from $17.7 billion in 2018.18 Given their immense potential for sensing applications, it's shocking that two-dimensional (2D) materials are still neglected in the field of microfluidic sensors. Although various publications have detailed the most recent achievements of graphene-based microfluidic sensors and LOD for detecting a broad spectrum of analytes from biomolecules to gases and water pollutants, more research is needed.5,12,15 Further research is required despite recent improvements in graphene-based LOD and microfluidic sensors for detecting a wide range of analytes, including biomolecules, gases, and water pollutants. To the best of our knowledge, there is no comprehensive literature review report on the application of 2D material-based microfluidic sensors and their capacity to detect a broad range of analytes.
Furthermore, MXene, TMD, and MOF-based microfluidic sensors are new, with no comprehensive review study available. As a result, a current review concentrating on 2D nanomaterial microfluidic sensors is needed to highlight the advantages of 2D nanomaterial-based microfluidic sensors and to provide ideas for further increasing sensor performance/fabrication for real-world applications. Current breakthroughs in graphene, TMD, MXene, and MOF-based microfluidic sensors will be discussed in this review, with a focus on biomolecule, water and gas pollutant detection. Quick and low-concentration detection using 2D microfluidic sensors are the most appealing advantages of microfluidic sensors. They enable early contaminant detection, early disease diagnosis, and steady environmental monitoring, as well as time savings for routine low-concentration examination. We also look at the benefits and drawbacks of 2D nanomaterial microfluidic sensors and provide recommendations for future studies in this area. We make a concerted effort to emphasize the most important work that may be found using Google Scholar, SciFinder, Web of Science and Scopus. In this case, a literature search was conducted using the Scopus database and the keywords “microfluidic sensor” and “graphene + TMD + MXene + MOF” to select papers for review. The number of articles published in 2D material-based microfluidic sensors is shown in Fig. 2.
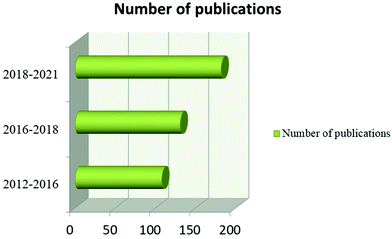 |
| Fig. 2 Number of papers published in 2D material-based microfluidic sensors. | |
1.1 Why 2D materials?
In 2007, Pokropivny and Skorokhod classified nanomaterials into 0D, 1D, 2D and 3D based on their confinement.19,20 Compared to other nanomaterials, two-dimensional materials have numerous advantages which makes them highly desirable for their application in microfluidic sensors. Firstly, they have high surface–area-to-volume ratio, enabling a wide range of interactions between two-dimensional material and analytes.21 This enhanced interaction property was utilized to design and develop sensors for the quantitative detection of alkali, gas, and heavy metals to biologically relevant molecules (e.g., lactate, glucose, DNA and protein).20,22 Further, the high surface to volume ratio property imparts some additional benefits, including greater amount of active sites,and easy surface functionalization. Secondly, 2D materials exhibit a broad spectrum of conductivity, which can be further fine-tuned by configuration and morphology engineering, enabling effective signal transduction via a molecular binding event.23 Additionally, dimensionality engineering methodology can be adopted to tune the inherent characteristics of the 2D materials, such as their fluorescence, conductivity, reactivity, and magnetic permeability.20 Aside from the above mentioned benefits 2D nanomaterials are advantageous in terms of their device integration compatibility.24 Especially, they have excellent compatibility with the existing ultra-thin silicone channel technology, while the integration of the device in 0D materials is difficult, it is challenging to develop electrical contacts with 1D materials and miniature devices with 3D materials.25 Finally, we believe that for future generation microfluidic sensors: the below mentioned properties of 2D materials will be highly beneficial (1) atomically thin two-dimensional material nanosheets offer a greater surface area due to the full surface exposure of the atoms; (2) comparing the basal plane of two-dimensional materials edge sites is chemically more reactive; and the open space of van der Waals allows electrolyte intercalation and (3) with their flexibility and robust laminar structure, 2D materials could be easily integrated into microfluidic beds and flexible wearable sensors.22,26
1.2 Fabrication of microfluidc devices
The methodologies usually employed for the manufacturing of microfluidic sensors can be split into two major groups: top-down and bottom-up. Top-down involves large-scale patterning and reduction of lateral dimensions to the nanoscale. Top-down approaches are further subdivided into three types (i) surface machining (ii) film/bulk machining and (iii) mold-machining. Bottom-up techniques use highly regulated chemical reactions to arrange atoms or molecules to form nanostructures. Table 1 summarizes the advantages and disadvantages of these fabrication strategies.
Table 1 Fabrication techniques of microfluidic sensors
Fabrication techniques |
Advantages |
Disadvantages |
Chemical wet etching |
Simple process, cheap, high selectivity with controllable etching rate |
Chemical contamination, orientation dependant, under cutting and poor repetability |
Plasma etching |
High reproducablilty, easy to control, high feature resolution and no liquid chemical waste |
Poor selectivity, high cost, potential radiation damage |
Soft lithography |
Low cost, ability to manufacture 3D geometries, and great resolution (down to a few nm)206 |
Pattern distortion, prone to defect206 |
Optical lithography/conventional photolithography |
Microscale features benefit from high wafer throughputs207 |
Typically, a smooth surface is required to begin with, with chemical post-treatment required207 |
Injection molding |
Complex geometry, fine details, and 3D geometries are simple to produce, with a low cycle time, large production, and high automation208 |
Exclusively used for thermoplastics, expensive mould, difficult to produce huge undercut shapes208 |
Hot embossing |
Microstructure replication that is cost-effective, precise, and quick, as well as mass production209 |
Complex 3D structures are difficult to construct since they are limited to thermoplastics209 |
Laser photoablation |
Large format production, rapid210 |
Limited materials, multiple treatment sessions210 |
1.3 Detection principles of microfluidic sensors
The large-scale integration of numerous microfluidic components, such as micropumps, microfluidic mixers, microchannels, microvalves and other elements to handle and regulate fluids at the microscale, is involved in integrated microfluidic devices.27 They are widely employed in biological, chemical, and medicinal research. There have already been numerous thoughtful review articles on related topics.27–30
In the realm of chemical, biological diagnosis, or analysis on microfluidic devices, various detection methods are available. Microfluidic detection methods are divided into three categories: mass spectrometry methods,31–33 electrochemical34–36 and optical methods.29,37,38 Because of their selectivity and sensitivity, electrochemical and optical approaches are the most commonly used. In addition to the basic technologies mentioned above, methodologies such as acoustical,39,40 nuclear magnetic resonance (NMR)41,42 spectroscopy and magneto-resistive43 are being combined with microfluidics for sensing applications. As shown in Fig. 3, the common optical detection methods include direct detection by detecting light properties such as absorbance,44 luminescence45–47 and fluorescence48–50-based approaches, as well as light property modulation detections like surface plasmon resonance (SPR) detection.51–53 These techniques often include evanescent waves,54 fibre optics,30 interferometry,55–57 Raman spectroscopy,58,59 SPR and optical waveguides.30
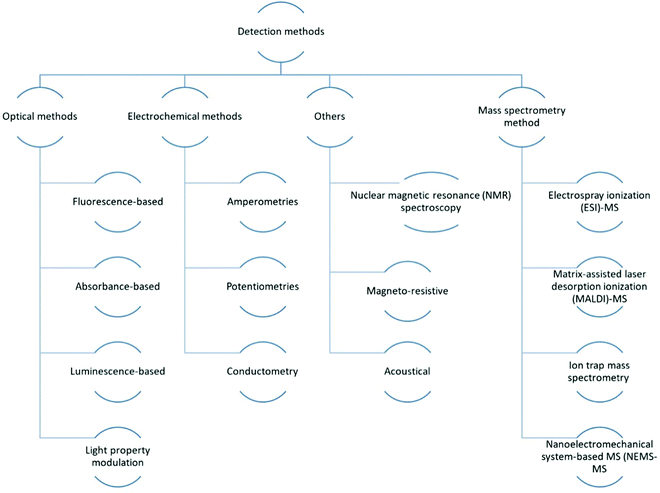 |
| Fig. 3 Detection principles of microfluidic sensors. | |
Electrochemical measurements, which are typically used to detect electroactive species, are based on electrical property modifications of analyte species that undergo redox reactions. There are three types of measurements: amperometries,60–62 conductometry63,64 and potentiometry measures.65 The principles of these methods was already been reviewed.
Mass spectrometry (MS) is capable of performing extremely selective detection by tracking the route of ions in magnetic and/or electric fields, revealing the ions' mass and charge.66 Its major application is in proteomic investigations for protein separation and identification based on protein fragmentation patterns. There are two methods for identifying following separation. The first method is direct detection, which involves searching through the database utilising the different types of proteins obtained. Tandem MS is a more complex method for obtaining protein fragments/ions for sequence tagging.67 For this application, numerous MS configurations30,68,69 have already been designed to connect with microfluidic devices which is shown in Fig. 3.
Other approaches, such as NMR spectroscopy, have been investigated for use in microfluidic detection in addition to the three basic categories. It is a well-developed detection approach in life sciences and chemistry that makes use of the magnetic characteristics of nuclei, as well as chemical shift Knight shift and/or the Zeeman effect, for detection. Biological and chemical analyte species such as proteins and nucleic acids can be detected using NMR spectroscopy. However, the low sensitivity of standard NMR detection techniques has limited its employment in microscale systems. Despite the technological obstacles, NMR remains a viable alternative to optical or electrochemical technologies for microfluidic applications: It does not have the problem of optical accessibility for the ROI, and the data acquisition time is substantially quicker, making time-resolved studies more easier; it has superior detection pervasiveness than electrochemical approaches, which are limited to electroactive substances. As a result, resolving the weak NMR signal problem in microsystems, whether by signal augmentation or better detection instrumentation, will open the door to a wider range of NMR lab-on-a-chip applications.30
1.4 Applications of microfluidic sensors
Microfluidic sensors have been widely used in various life science and medical detection reserach and applications. There have already been numerous thoughtful review articles on related topics. Gao et al. summarised the applications of microfluidic chip technology in food safety sensing.70 Its application in biomedical field was reviewd by Pattanayak et al.71 also in the field of environmental analysis was summarized by Zhang et al.72 Microfluidic sensors also allow for the creation of quick, sensitive, and portable diagnostic tools, allowing for the rapid and accurate identification of a wide range of infections and its application in pathogen sensing was reviewed by Marihofer et al.73
2. Graphene-based microfluidic sensors
Due to its outstanding sensitivity to electronic disruptions when target species (biological, gas or chemical) interact, graphene is now being researched and developed as biological, physical, and chemical electronic sensors for targets such as different biological agents, chemical species, and gases. The ever-increasing usage of sensors in society and the growing demand for devices with the following characteristics: high performance, low power consumption and cost, which is crucial for portable or distant applications, has motivated these research endeavors.74–76 The effectiveness of sensing instruments is highly dependent on the materials used and the design concepts used. Graphene has enormous potential as a sensor material for applications such as LOD, point-of-care, environmental, and industrial monitoring in this regard. Indeed, it has been proposed that graphene technology could develop low-cost, compact sensors with previously unimagined capabilities.77 The advantages of graphene-based microfluidic sensors include rapid diagnosis, real-time detection, low-cost, multi-target analysis, automation, small sample volume, and higher selectivity and sensitivity.78 So far, there have been numerous reviews of graphene-based microfluidic devices. In 2018, Chen et al. published the first review of graphene-based microfluidic devices for different applications such as contaminant detection, virus and bacteria detection, glucose and protein detection, and sensor applications. However, research on graphene-based microfluidic sensors and LOD is still in its early stages, despite predictions that the point-of-care application will be a hot topic.79 Chaudhery Mustansar Hussain and Joydip Sengupta published a review on graphene-based LOD devices the following year, attempting to provide insight into point-of-care devices as well. This paper examines the critical applications of graphene-based microfluidics, including everything from detecting chemical food pollutants to detecting retinal electrical signals and even early stage cancer diagnosis, as well as the fabrication method for graphene-based LOCs (2). In the same year M. Materón et al. discussed about graphene based microfluidic and chip devices for detection of biomolecules.78 Wu et al. published a review on graphene-based microfluidic sensors in 2020, which includes graphene-based microfluidic FET sensors, optical sensors, and biosensors for chemical and biomolecular detection.80 Microfluidic sensors based on graphene can be classified according to applications, such as chemical and biological analysis and other applications. Biological applications are further subdivided into those used for diagnostic purposes and those used for biomolecule detection.
2.1 Graphene-based microfluidic biosensors
Graphene and its oxidized counterparts (such as GO, rGO, and others) have become critical nanomaterials in the domain of biosensors, notably in the construction of low-cost optoelectronic devices, due to their unique optoelectronic capabilities. The research purpose of combining microfluidic technology in biosensing is to implement additional miniaturized devices capable of high selectivity and sensitivity, small throughput, fast response and automated testing. The potential benefits such as portability and high-throughput analysis are realized by combining microfluidic technology and biosensing, resulting in intelligent real-time detection. Graphene nanomaterials could be used to functionalize and recognize a variety of biological analytes because biomolecules come in a variety of sizes.80,81
2.1.1 For cancer detection.
The gold standard for tumor identification, confirmation, and classification is still solid tissue biopsies, which involve the removal of tumor tissue and subsequent cytological and histopathological examination. In any case, the procedure is excruciatingly painful and intrusive which can also result in Inflammation, bleeding, and the spread of cancerous cells. Furthermore, the number of samples collected is insufficient in most cases. Because of tumor tissue's spatial and temporal heterogeneity, the results may be inconclusive.82,83 On the other hand, tumor tissues shed various components into the body fluids, including cells, exosomes, proteins and nucleic acids, which could serve as tumor biomarkers that provide information similar to tissue biopsies.84 The current biomarkers for cancer detection were tabulated by Choi et al.85 Although these fluid biopsies can be taken from any biological fluid, blood is the most commonly used because it can detect almost all cancer types. In contrast, saliva or urine can only detect specific types. Despite this, identifying biomarkers in small quantities using blood samples is difficult due to diverse cells and chemicals. As a result, the advancement of sensitive systems for detecting and analyzing low concentrations of biomarkers in body fluids is a way to facilitate personalized medicine for cancer treatment.84 Although microfluidic technologies have a lot of potentials, combining them with two-dimensional graphene improves the sensitivity and selectivity of microfluidic sensors and can help detect cancer biomarkers. In 2012, Cao et al. published the first graphene oxide (GO) based Förster resonance energy transfer (FRET) microfluidic chip for cancer cell detection. Because the aptamer-based microfluidic chip was not producing enough signals, they were inspired to develop this FRET aptasensing microfluidic device, which takes advantage of graphene's fluorescence quenching and DNA adsorption abilities. This FRET on biosensing microfluidic chips was an excellent choice for detecting cancer cells, and the aptamer immobilization and washing steps could be eliminated. This GO-enhanced microfluidic technology is the first graphene-based microfluidic sensor with significant sensitivity and selectivity for cancer cell detection.86 Soon after these studies, Wu et al. developed microfluidic paper-based electrochemical immunodevices to detect cancer biomarkers using a signal amplification strategy obtained by incorporating graphene, HRP, and antibody co-immobilized silica nanoparticles.87 Although several publications and review papers based on graphene and graphene derivatives-based microfluidic devices for cancer detection are available, we are only discussing recent papers, i.e., those published between 2020 and 2021.
Wu et al. investigated a high-performance microfluidic sensor for the early detection of ovarian cancer. This microfluidic sensor is fabricated with a GO-assembled substrate that helps immobilize the antibody where the large surface area property of graphene results in enhanced immobilization of capture antibodies. They designed a microfluidic chip with multiple parallel channels shown in Fig. 4a and a microprinted capture antibody barcode above the detection substrate (Fig. 4b). This captured antibody barcode was then printed over GO substrate. The sample loading chip is made of microwells (Fig. 4c). Each microwell covers an entire array of the capture antibody barcode, allowing the immobilized antibodies to capture all ten biomarkers in the sample material. The biomarker antigen is then incubated for 10 minutes with the immobilized antibody before the fluorescence-labeled detection is specifically conjugated with the antigen, as shown in Fig. 4d and e. This microfluidic sensor was able to detect all of the traditional ovarian cancer indicators and inflammatory factors with an ultra-low detection limit of roughly 0.01 μ mL−1 and 1 pg mL−1. As a result, this microfluidic sensor can detect ovarian cancer at an early stage. These antibodies all have a specific reaction with the respective biomarkers in the detection chip. As shown in Fig. 4g, coupled antigen–antibody pairs have significantly higher fluorescence intensity than mismatched pairs.88 Niu et al. reported using an antibody-functionalized GO lab-on-chip device to isolate and analyze circulating tumor cells (CTC) from the bladder of cancer patients. They employed a GO chip coated with a biotinylated anti-EpCAM and anti-EGFR antibody mixture to capture CTCs from whole blood. Immunofluorescence staining for cytokeratin (cancer cell marker), DAPI (nuclei), EGFR and HER2 (invasive phenotype markers), and CD45 (white blood cell marker) were used to count CTCs in patient samples. CTCs were defined as cells with a DAPI + CK + CD45-phenotype.89
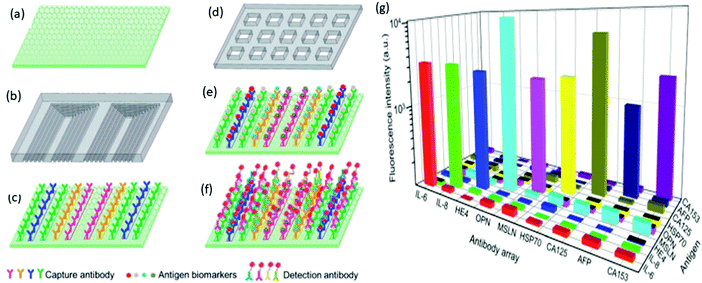 |
| Fig. 4 (a) Substrate for the detection of ovarian cancer biomarker (b) chip was microprinted (c) antibody capturing by the barcode which was printed on the substrate (d) sample filling chip (e) biomarker antigens caught by antibody barcode (f) fluorescence labeling for detection of biomarkers (g) detection specificity of the prepared chip. Source: reprinted from Y. Wu, C. Wang, P. Wang, C. Wang, Y. Zhang and L. Han, A high-performance microfluidic detection platform to conduct a novel multiple-biomarker panel for ovarian cancer screening, RSC Adv., 2021, 11(14), 8124–8133. | |
A growing amount of attention is being paid to combining the appealing qualities of aptamers with the particular benefits of electrochemical detection technique. Electrochemistry gives new avenues for integrating aptamer interactions with the signal-generating component, allowing for speedy, low-cost protein diagnostics.90 As a result, an electrochemical approach for detecting EGFR was created. Using these advantages of the electrochemical detecting technique, Wang et al. built an origami-paper-device using graphene nanocomposite. Wax printing was used to generate the device's hydrophilic and hydrophobic regions, which define fluid channels. On the other hand, the electrochemical electrodes were created using screen printing (shown in Fig. 5a). By simply folding the paper to allow valving or flow, the system facilitated sample processing and detection. Thionine (THI)/amino-functionalized graphene (NH2–GO)/gold particle (AuNP) nanocomposites were fabricated as working electrodes (WE) also immobilize aptamers via Au–S bonds (Fig. 5b). Here the amino-functionalized graphene exhibited a corrugated structure and thus resulted in enhanced surface area of the electrode; hence they can load a large amount of TFI molecule and AuNP. They presynthesized thiol-modified anti-EGFR aptamers and used a simple electrochemical detection approach that did not require tagging of either antigen or aptamer. For EGFR, this suggested aptasensor has a LOD of around 5 pg mL−1 and a linear range of 0.05 to 200 ng mL−1. The electrochemical response was shown to be lower when the EGFR aptamer was immobilized on the electrode surface compared to graphene-modified electrodes (Fig. 5c). Insulating aptamers appear to have hampered electron transport, resulting in a reduction in current responsiveness. More insulating immunocomplexes would form with a greater concentration of antigens, resulting in a decreased electrochemical current. The CV analysis showed that the electrochemical paper-based aptasensor could be constructed and operated satisfactorily. Electron transport appears to have been impeded by insulating aptamers, resulting in a decrease in current responsiveness. Also, more insulating immunocomplexes would form with a higher concentration of antigens, resulting in a lower electrochemical current. The CV results demonstrated that the electrochemical paper-based aptasensor was successfully manufactured and functioned. Similar results were obtained from DPV, where the modified electrode showed a DPV response with a peak current of roughly 16.99 μA, which was lowered to 14.98 μA after aptamer immobilization. This suggested aptasensor unites low-cost paper-based microfluidic devices with an electrochemical detection approach that is extremely sensitive. The origami approach streamlined the device even more and reduced sample consumption.91 Another paper-based electrochemical sensor for the early quantitative detection of pancreatic cancer was recently published by Prasad et al. Pancreatic ductal adenocarcinoma (PDAC) is another significant cancer killer. People with PDAC have higher levels of PEAK1 protein expression than healthy people. A cheap, disposable, unique yet straightforward paper-based electrochemical immunosensor was created to trace the PDAC biomarker PEAK1 using a standard sandwich-type immunoassay. They developed this sensor over paper-based electrodes using a stencil printing technique. Then GO was simply drop cast over paper-based electrodes for covalent modification to incorporate the capturing antibody (Anti-PEAK1). This immunosensing device uses gold nanoparticle (AuNPs)-tagged Anti-PEAK1 bioprobes to detect PEAK1. With a LOD of around 10 pg mL−1, this sensor displayed remarkable sensitivity during PEAK1 detection.92 Torul et al. recently developed another paper-based electrochemical sensor based on gold nanoparticle/graphene and Au nanoparticle/MoS2 for the selective and sensitive identification of miRNA-155 and miRNA-21, which are the most significantly altered miRNAs in the majority of cancer types. Torul et al. constructed (Fig. 6a) the hydrophobic barriers of this paper-based sensor using wax printing, similar to Wang et al.91 work, and the three-electrode system using a stencil technique, identical to Prasad et al.92 The sequence of a complementary miRNA target was identified using differential pulse voltammetry (DPV) with a thiol-linked synthetic DNA probe placed onto the working electrode in a redox[Fe(CN)6] solution. Hybridization was recognized on the paper-based biosensor based on the difference in redox probe signal in the presence/absence of miRNA hybridization. Here hybridization efficiency is calculated using the equation and using voltammograms given in Fig. 6b and c
|  | (1) |
where Δ
I =
Ihybrid –
Iprobe and the highest HE % calculated was around 37.1% for miRNA-155 and miRNA-21 target hybridization. The LOD was around 0.19 μg mL
−1 for miRNA-155, whereas it was around for miRNA-21. The sensitivity was reported around 295.3 and 351.1 μA mL μg
−1 cm
−2 for AuNP/RGO-modified paper electrode comparing AuNP/MoS
2 – modified paper electrode.
93 Using a continuous and straightforward coating process (
Fig. 7a), graphene and poly(3,4-ethylene dioxythiophene):poly(styrenesulfonate) (PEDOT:PSS) modified paper-based EIS aptasensor were constructed by Yen
et al. for carcinoembryonic antigen (CEA) detection. This glycoprotein CEA has a molecular mass of almost 180–200 kDa.
94,95 It acts as a tumor marker and is critical in cancer detection and treatment. The presence of high levels of this tumor marker has been linked to various cancers, including breast, colorectal, ovarian, pancreatic, lung, and gastric cancers. This low-cost aptasensor detects CEA in a linear range of 0.77–14 ng mL
−1 in standard buffer solutions and human serum samples. In both samples, the LOD for CEA was 0.45 ng mL
−1 and 1.06 ng mL
−1, respectively.
96
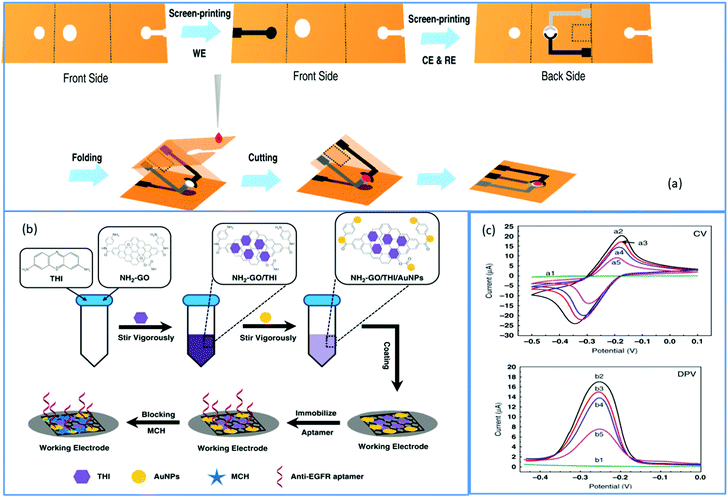 |
| Fig. 5 (a) Fabrication procedure of origami paper-based sensor (b) modification process (c) CV and DPV response of the fabricated sensor. Source: reprinted from Y. Wang, S. Sun, J. Luo, Y. Xiong, T. Ming, J. Liu, et al., Low sample volume origami-paper-based graphene-modified aptasensors for label-free electrochemical detection of cancer biomarker-EGFR, Microsyst. Nanoeng., 2020, 18, 6(1), 1–9. | |
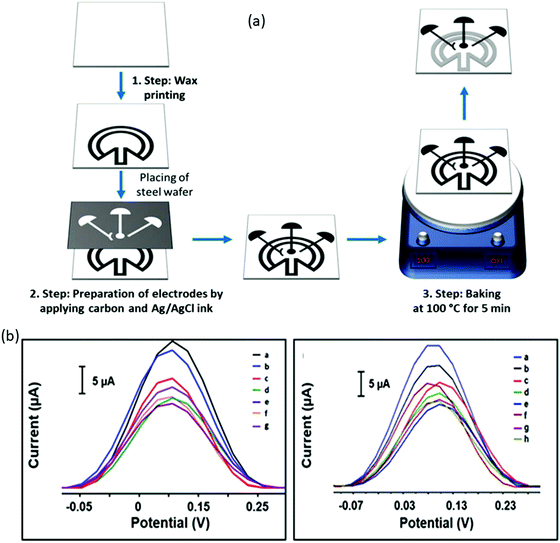 |
| Fig. 6 (a) Steps involved in the fabrication of paper-based sensor for detection of microRNAs (b) Voltammogramms in the absence of microRNAs. Source: reprinted from H.Torul, E. Yarali, E. Eksin, A. Ganguly, J. Benson, U. Tamer, et al., Paper-Based Electrochemical Biosensors for Voltammetric Detection of miRNA Biomarkers Using Reduced Graphene Oxide or MoS2 Nanosheets Decorated with Gold Nanoparticle Electrodes, Biosensors., 2021, 11(7), 236. | |
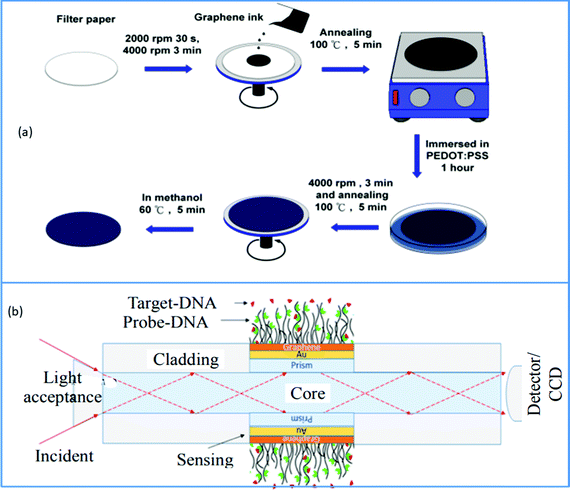 |
| Fig. 7 (a) Steps involved in the fabrication and modification of electrochemical paper-based working electrode for the detection of CEA. Source: reprinted from Y.-K. Yen, C.-H. Chao, Y.-S. Yeh, A Graphene-PEDOT:PSS Modified Paper-Based Aptasensor for Electrochemical Impedance Spectroscopy Detection of Tumor Marker, Sensors., 2020, 20(5), 1372. (b) Schematic illustration of four-layered model for fiber optic SPR biosensor for detection of BRCA1 and BRCA2 breast cancer genes. Source: reprinted from Hossain MdB, Islam MdM, Abdulrazak L. F., Rana MdM, Akib TBA, M. Hassan, Graphene-Coated Optical Fiber SPR Biosensor for BRCA1 and BRCA2 Breast Cancer Biomarker Detection: a Numerical Design-Based Analysis, Photonic Sens., 2020, 10(1), 67–79. | |
Since pristine graphene lacks a semiconducting gap, it cannot be used in electronic devices. As a result, one of the most practical methods of controlling graphene's semiconducting properties is doping.97 Boron-doped graphene is of particular interest because it can stimulate various chemical and electrochemical activities in the graphene basal plane.98,99 Aside from boron-doped graphene, boron-doped diamond-graphene hybrid structures were another hotspot in carbon materials research. However, its use in immunosensors is limited. Hongji et al. realized this and created a boron-doped diamond (BDD)/vertical boron-doped graphene (BG) composite film coated with Au nanoparticle via CVD and electroplating, resulting in a high surface area and electrocatalytic activity. This immunosensor was capable of simultaneously quantifying carcinoma antigen 125 (CA 125) and CEA at concentrations of 0.5–100 pg mL−1 and 0.5–100 mU mL−1, respectively, with LOD around 0.15 pg mL−1. The simultaneous detection of CA125 and CEA was accomplished utilising a sandwich-type signal acquisition method that employed an electrochemical sensor based on BDD/Au-VBG as a working electrode, TB and Fc as redox probes, and Au–TiO2 particles as a carrier.100
BRCA1 and BRCA2 are two specific breast cancer susceptibility genes discovered in human DNA. The BRCA (breast cancer abbreviation, typically pronounced bracka) genes are passed down from their parents to men and women. BRCA1 and BRCA2 are tumor suppressor genes that inhibit cancers from developing when they are activated. BRCA1 and BRCA2 mutations, on the other hand, are responsible for almost half of all hereditary breast cancer cases. As a result, the early detection of these genes is crucial. Breast cancer is currently diagnosed using mammography, PCR screening, ultrasound and other methods. However, due to its small size, this tumor is difficult to detect.101,102 The surface plasmon resonance approach is now being utilized to collect data of DNA molecular bonding, allowing BRCA1 and BRCA2 breast tumors to be immediately detected to address the demand for effective and precise ways of breast cancer screening. Several methods are adopted to excite surface Plasmon, including coupling with fiber optics, prisms, nanoparticles, grating, etc. But increasing the sensitivity of this type of biosensor is a difficult task. In this regard, graphene is one of the materials explored extensively due to its excellent features. This substance has been shown to boost SPR sensitivity in various studies, both theoretically and empirically. The review work done by Nurrohman et al. discussed the latest development in graphene-based SPR biosensors.103 Taking advantage of the most notable features of these graphene-based SPR biosensors, Hossain et al. described a very effective fiber-optic SPR biosensor for monitoring BRCA1 and BRCA2 breast cancer. They used an attenuated total reflection (ATR) approach to identify deoxyribonucleic acid (DNA) hybridization and individual point mutations in the BRCA1 and BRCA2 genes. Fig. 7b depicts the structural layout of this newly designed graphene-based fiber optic SPR biosensor. This suggested sensor may detect precisely matched and mismatched DNA interactions between probing and target DNA based on differences in the SPRF and SPR angle. This graphene-based SPR biosensor's improved sensitivity is owing to graphene's property to absorb light.104
Biological field-effect transistors (BioFETs) have yet to be recognized, despite their potential for substantially higher sensitivity and much faster quantification, with sensing devices primarily constrained to two-three electrodes electrochemical biosensors.105,106 Graphene is employed as a sensing material in BioFETs because of its distinctive electrical and chemical characteristics. These graphene-based BioFETs exhibit a higher efficiency when run in an electrolyte gated approach without solid-state dielectric due to the electric double layer formed between the graphene and electrolyte.107,108 This electrochemical gate is more effective than a typical SiO2 back gate with a thickness of hundreds of nanometers.109 Sotirios et al. reported an electrolyte gated PCB-based FET DNA for the first time. To enable label-free DNA sensing, graphene ink was drop-casted to produce the transistor channel, and PNA probes were placed on the graphene channel. After a fully inkjet-printing compatible construction approach, it was demonstrated that the sensor could specifically recognize the complementary DNA sequence. The first FET using a PCB was a core board as the substrate and PCB electrodes as the transistor source, drain, and gate pads. To maximize downsizing and integration, the traditional bulky reference electrode is substituted with an in-plane PCB electrode. This design hasn't gotten much attention in the literature. Fig. 8b given here shows that with reduction of gate voltage Ids value decreases. This identifies the gate influence on Ids and reveals the graphene's p-type behavior. The absence of apparent Schottky barriers at the graphene–electrode contact is indicated by the linearity of these curves. The LOD of this PCB-based FET was around 1 nm calculated using the curve shown in Fig. 8c, which is relatively high without microfluidic integration and biochemistry optimization. Furthermore, ssDNA detection is effective at this range of concentrations with excellent sensitivity (30.1 mV decade−1). Their study demonstrate the inkjet printing's potential to incorporate semiconductor chips into Lab-on-PCB platforms seamlessly for the first time. This opens the way for inkjet-printed Lab-on-PCB platforms driven by printed, electronic circuits with significantly greater intricacy and processing capacity.110 Niazul et al. suggested a biosensor utilizing a graphene field-effect transistor (GFET) functionalized with target-binding aptamers for detecting the thrombin protein biomarker using a typical microfluidic fabrication approach. This sensor was used with microfluidic devices to produce good sensing performance. The previously published GFET-based microfluidic sensor, on the other hand, was not suitable for real-time applications since it lacked LOD in the picomolar range as well as a detection range in micromolecular concentration.111
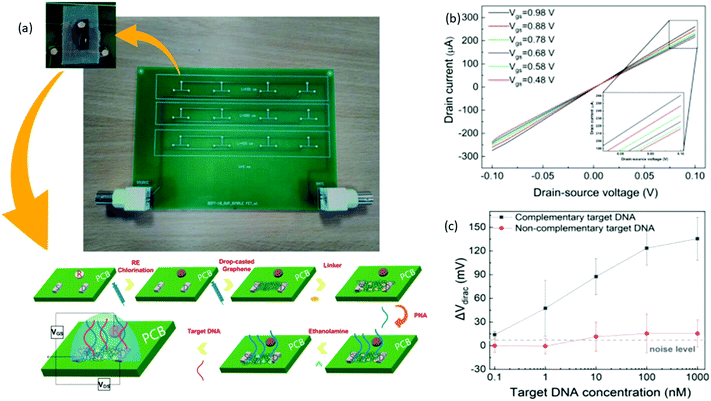 |
| Fig. 8 (a) Printed circuit board-based graphene FET sensor (b) output curves of the graphene electrolyte-gated transistor (c) VDirac shifts for various complementary and non-complementary target DNA concentrations. Source: reprinted from S. Papamatthaiou, P. Estrela, D. Moschou, Printable graphene BioFETs for DNA quantification in Lab-on-PCB microsystems, Sci, Rep., 2021, 11(1), 9815. | |
The nanocomposite of rGO and Cys hydrogels could give an exciting substrate with large carboxylic groups leading to higher antibody loading, good stability, and strong surface absorption ability, leading to increased target molecule sensitivity.112 Singh et al. developed a dual-modality microfluidic sensor for cardiac myoglobin sensing utilizing this Cys–rGO hydrogel. A patterned Au was first modified using rGO–Cys hydrogel before being inserted into a PDMS microchannel via in situ functionalization with specific cardiac myoglobin (cMb) antibodies utilizing EDC-NHS chemistry. Researchers used dual-modality performance employing differential pulse voltammetry and SPR methods to detect the cardiac myoglobin biomarker and perform the real-time binding kinetic study. They used an SPR technique in plasmonic detection mode to find out cMb concentration using antibodies-antigen interaction on the surface of the microfluidic sensor. The surface density of immobilized cMb molecules above the Cys-RGO hydrogel is determined using the equation
| 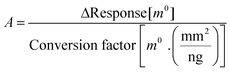 | (2) |
where
A denotes the surface density of immobilized cMb.
Eqn (2) is used to calculate the quantity of immobilized cMb using this surface density. Also, the number of cMAbs bound onto the hydrogel is determined using
eqn (3) | Amount = Surface density (ng mm−2) × surface area (mm−2) | (3) |
|  | (4) |
The number of cMAb molecules over the surface of this sensor was around 1.38 × 10
15. The SPR angle was also shown to rise with increasing concentration, which can be attributed to improved kinetic binding between this cMb and cMb conjugated hydrogels. Here DPV sensing of the conjugated hydrogel sensor shows LOD of 4 pg mL
−1 with sensitivity around 196.66 μA ng mL
−1 cm
−2. In contrast, the SPR sensing exhibited LOD 10 pg mL
−1 with around 42.86 m ng
−1 mL
−1.
113
2.1.2 For biomolecule detection.
In vitro analysis requires particular biomarkers for preliminary disease diagnosis. Small metabolic molecules such as glucose, dopamine and hydrogen peroxide (H2O2), which have specific concentrations suggesting definite pathogenic processes in biotic fluids, could be utilized as biomarkers to diagnose diseases early.114–116
2.1.2.1 For glucose detection.
The Laser induced graphene (LIG) has been utilized either in its pure state or combined with other composite materials to create microfluidic prototypes to detect glucose.117 LIG-based bioelectrodes were used to develop PDMS-based microfluidic biofuel cells by Prakash et al. This LIG derived from PI sheets was incorporated into a microfluidic device made of PDMS using traditional soft lithography. During the irradiation procedure, the CO2 lasers' power and speed were tuned. They submerged LIG in a linker solution with 1-ethyl-3-(3-dimethyl aminopropyl) carbodiimide to bind the glucose oxidase and lactase on the sensor surfaces. The enzyme solutions were drop-cast on the irradiated surface and left for 2 hours at RT for the immobilization process. PET sheets were sliced using a Y-shaped microchannel to create the microfluidic cells. A microchannel structure was built using PDMS template curing, where this microfluidic cell shows the fluid rate and power density of around 200 μL min−1 and 13 μW cm−2. Here electrochemical redox reactions and polarisation processes in the microfluidic environment contributed to the developed system's exceptional performance. This sensors linear range was around 10–40 mm, and the current increased linearly as the voltage increased.118 Bipolar electrode (BPE)-based electroluminescence (ECL) sensors have recently gained popularity and recognition due to their high specificity, simple instrumentation, good selectivity, lack of background signal and controllability. This device is very simple to operate with quick processing and multiplexing. Manish et al. fabricated a compact 3D printed ECL sensor with LIG-based open BPE. Graphene was synthesized utilizing CO2 laser ablation over polyimide (PI) sheets. They injected H2O2 and luminol into the microchannels to detect H2O2, and when a sufficient voltage is given to the driving electrode, a redox process begins. As a result, on the anodic face of the BPE, an ECL signal is formed, which may be caught using a smartphone. The reaction of H2O2 with luminol is as follows | Luminol – e− – H+ Diazoquinone | (5) |
| H2O2 + Diazoquinone Luminolendoperoxide | (6) |
| Luminolendoperoxide N2 + 3-aminophthalate | (7) |
| 3-Aminophthalate 3-aminophthalate +hγ | (8) |
The BPE anode was treated with GOX and maintained at room temperature (RT) for around 5 minutes to detect glucose. Following that, an equal amount of luminol and glucose was injected into the channel. With optimizing parameters like pH, luminol concentration, applied voltage, channel length and so on, this sensor shows LOD around 0.138 μM and 5.8729 μM for glucose and H2O2, respectively.119 He also built a LIG-BPE-ECL sensing platform using the same laser ablation process, but they made a closed BPE rather than an open BPE. Here Individual sensing of Vitamin C, vitamin B12, and H2O2 with linear ranges of 0.5–1000 nM, 1–1000 M and 0.5–100 M with LOD of 0.109 nM, 0.96 M and 0.303 M were used to verify the performance of this two-channel BPE-ECL-LIG-C device. Following that, a three-channel BPE-ECL-LIG-C device was created and concurrent detection of Vitamin C and Vitamin B12 was also achieved.120 Instead of these two and three-channel electrode systems, they validated a LIG-based single electrode (SE) system for enzymless ECL detection of multiple analytes. Using this sensor, they carried out the detection of H2O2, glucose, dopamine and xanthine in the linear range from 0.1 to 70 μM, 0.1 to 70 μM, 0.1 to 100 μM and 0.1 to 100 μM with a LOD around 1.71, 3.76, 3.40 and 1.25 μM respectively.121 Manish et al. developed an ECL device with a U-shaped bipolar electrode (U-BPE) for enzymless sensing of different biomarkers. With LODs around 2.51, 4.36, 4.01, and 5.32 μM, this ECL sensor was utilized to detect D-glucose, H2O2, choline, and lactate, among other biological analytes.122
Incorporating conductive materials such as graphite, GO and carbon nanotubes into chitosan-modified electrodes leveraging chitosan's biocompatibility was a potential strategy to enhance the selectivity and sensitivity of paper-based electrodes (μPAD). Using this strategy, Rokemono et al. created a chitosan/GO modified PAD for paracetamol and glucose electrochemical sensing by using simple solution casting and UV curing followed by coating with carbon paste. Here the impedance results show that the electron transfer process at the electrode/analyte solution contact accelerates with increasing analyte concentration. CV experiments show a linear response for analyte concentrations ranging from 2.5 to 100 M. Furthermore, regardless of the analyte, the sensitivity of the modified paper-based electrode rises with a different proportion of GO. By utilizing electrode coated with chitosan/GO (5 wt%) composites, the highest sensitivity for paracetamol sensing is 16 mA mM−1 and for glucose, sensing is around 4.4 mA mM−1.123 Cao et al. reported a paper-based microfluidic chips in point-of-care devices (POCD) employing 3D μPAD. Whitesides team's invention of 2D and 3D μPADs in which fluid motion occurs inside diverse microfluidic channel designs due to capillary driving force inspired them to create this electrochemical glucose biosensor. Here the aldehyde functionalized μPAD was manufactured using periodate oxidation. The new 3D μPAD type was made utilizing photolithography, whereas screen printing technically produced 3D paper-based electrodes. This electrochemical sensor's working electrode was made from a Prussian blue-deposited rGO-tetraethylenepentamine (PB/rGO-TEPA) composite. In contrast, the counter/working electrode was aldehyde functionalized and employed to immobilize GOD. This sensor exhibits LOD around 25 μM over a broad linear range of 0.1–25 mM. Because of the excellent wettability of this paper-based electrode, when glucose was dribbled into the hydrophilic detecting region, it immediately came in contact with the GOD immobilized in the hydrophilic area, causing a particular enzyme-substrate interaction to create H2O2, as illustrated in the reaction formulae (1) and (2). The produced H2O2 was disseminated over paper by capillary action and reached the surface of the PB/rGO-TEPA modified electrode almost quickly. The PB immobilized on the surface of electrode was reduced to Prussian white (PW) while the electrode was functioning. Because of PW's excellent electrocatalytic reduction activity, H2O2 may be reduced, and PW can be oxidized to regenerate PB, as illustrated in reaction formulae (11) and (12).
| GOD(FAD) + Glucose GOD (FADH2) + Glucose acid | (9) |
| GOD (FADH2) + O2 GOD(FAD) + H2O2 | (10) |
| KFe3+ [Fe2+ (CN)6](PB) + e− + K+ K2Fe2+ [FE2+ (CN)6](PW) | (11) |
| K2Fe2+ [FE2+ (CN)6](PW) + ½ H2O2 KFe3+[Fe2+ (CN)6](PB) + OH− + K+ | (12) |
The use of this rGO-TEPA/PB composite as electrode modification materials allows for electron transport between the GOD and the electrode and considerably amplifies the current signal and improves reaction sensitivity. As a result, the change in decrease peak current produced by a change in glucose concentration can be utilized to quantify glucose.
124 A graphene-based field-effect transistor (GFET) has been used as a sensor by immobilizing bioreceptors on its surface while offering a controlled environment for monitoring and detecting biological/physiological processes. When an electric field is applied to the graphene surface, charged molecules induce an electrical reaction in GEFT.
125 The GFET has been effectively used to fabricate a high-performance glucose monitor thanks to graphene's enhanced electrical conductivity and unique structure. However, the commercially available bulk G-FET devices are enzymatic, and enzyme deactivation commonly compromises the precision and durability of these devices. Furthermore, they are frequently made on solid substrates (such as glass or silicon), limiting their wearability. For non-enzymatic glucose sensing, a flexible ultrathin affinity-based G-FET was fabricated by Yao
et al. This device was fabricated on an adhesive support, which allowed it to be easily peeled off and simplified the operation. The specific pyrene-1-boronic acid (PBA) was used to functionalize the graphene channel. When glucose molecules were injected into these graphene channels, electron-withdrawing PBA molecules recognized them. They formed glucose-boronate ester complexes, which are electron-rich, causing the conductivity of the graphene channel to change. This sensor shows a LOD of around 0.1 μM over a broad detection range of 1–0.5 mM.
126 Wiorek
et al. fabricated an epidermal pad for glucose analysis in perspiration that incorporates temperature and pH correction based on local dynamic sweat change during on-body measurements. The calibration parameters are modified to any change in temperature or pH throughout the athletic exercise using a unique “correction technique,” and this epidermal glucose biosensor (
Fig. 9) has a linear response range within the physiological amounts of glucose in sweat (10–200 M). Furthermore, the sensor also has excellent reversibility, quick response time and good selectivity.
127
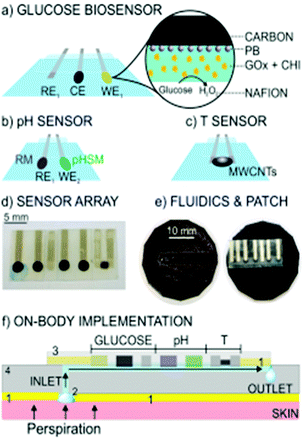 |
| Fig. 9 (a) Glucose, (b) pH potentiometric and (c) temperature sensor (d and e) image of microfluidic sensor (f) illustration of microfluidic sensor attached to the skin. Source: reprinted from A. Wiorek, M. Parrilla, M. Cuartero, G. A. Crespo, Epidermal Patch with Glucose Biosensor: pH and Temperature Correction toward More Accurate Sweat Analysis during Sport Practice, Anal Chem., 2020, 92(14), 10153–10161. | |
2.1.2.2 For other small molecules detection.
Fishlock et al. developed a modified electrochemical paper device (e-pads) for dopamine detection. The electrodes were positioned along the wax-defined microfluidic channel in this capillary flow device, using Whatman filter paper as the porous wicking substrate. The working electrode was implanted directly into Whatman filter paper using the paper as a precursor for LIG. This LIG is obtained by treating Whatman filter paper coated with flame-retardant spray, then treated with CO2 infrared Laser. This treatment helps to produce a 3D porous graphene network with high electrical conductivity and high-surface–area. They prepared this counter and working electrode using Laser alongside the wax defined microfluidic channel where the reference electrode was prepared using inkjet-printed silver. The prepared working electrode based on pristine graphene was functionalized using nitrogen and modified this N-functionalized graphene using MnO2. This microfluidic devices helps in detection of neurotransmitters.128
GEFT was fabricated by Schuk et al. to measure the concentration of L-lactic acids in various fluids. Lactate dehydrogenase enzyme was used to functionalize the active surface of graphene. This work proved graphene's ability to monitor L-lactic acid accumulation in human samples, implying that this material might be employed in more basic and low-cost equipment, such as flexible sensors for POC.129
Cystatin C (Cys C) is a 13 kDa protein made up of a single 120-amino-acid polypeptide chain.130 This serum's abnormally high levels have recently been revealed to be a prognostic sign for various conditions, including neurological problems, cardiovascular disorders, and cancer.131,132 Shalini and Uma Maheswari fabricated a Cys C detecting nano interface electrochemical immunosensor. A glassy carbon working electrode (GCE) was modified with nanocomposites of GO-chitosan (GO-Chit), which was then translated onto a paper-based microfluidic substrate. They fabricated this paper-based substrate using screen printing where carbon ink formulation was screen printed over a wax-coated paper following screen printing of Ag/AgCl paste. Compared with the modified GCE, this paper-based sensor shows a LOD of 2.6 pg μL−1 with a sensitivity of around 15.5 μA ng−1.133 Yang and his team with the concept of a laser-engraved (LEG) wearable bio-sensor that could be mass-produced to detect tyrosine levels (TYR) and uric acid (UA). A chemical sensor (LEG–CS) over polyimide for measuring low-level TYR and UA, a physical sensor (LEG–PS) for temperature and respiration monitoring and a multi-inlet micro-fluidic module for dynamic sweat sampling are all included in this device. Electrochemical sensors use raster engraving, while microfluidic and biological sensors use vector engraving. This sensor had a sensitivity of 0.61 and 3.50 50 μA μM−1 cm−2 for Tyr and UA, respectively, with LOD of 0.74 and 3.6 μM. This sensor-relied on LEG exhibited excellent selectivity at physiologically acceptable levels compared to other sweat studies.134 Stojanovic et al. fabricated a tiny microfluidic chip to detect ascorbic acid selectively. This microfluidic channel was created using the xurography procedure with a microfluidic channel between two silver electrodes. To enhance the conductivity and the electron transfer mechanism, a graphene sheet was placed between the gaps of these electrodes. The sensing mechanism for this microfluidic platform was based on an increase in overall conductivity as ascorbic acid concentration increased, resulting in an increase in capacitive parameters and a decrease in resistive parameters and an increase in the suggested equivalent electrical circuit135
2.1.3 For pathogen detection.
Viral infections are among the leading causes of serious pandemics and infectious illnesses, resulting in billions of dollars in economic losses each year and thousands of deaths. In the instance of COVID-19, global economies were shut down for months, and physical separation, along with severe changes in the social behavior of many persons, resulted in a slew of problems for all countries. As a result, developing a speedy, low-cost, and sensitive viral detection method is crucial for improving human living standards while utilizing biomedicine, bioresearch and security also environmental science. Graphene has emerged as a viable material for microfluidic biosensor fabrication due to its several fascinating properties, including exceptional conductivity, ultrahigh electron mobility, and remarkable thermal conductivity.136 As a result, graphene-based analytical LOC and microfluidic devices have been proven to be capable of detecting a wide spectrum of viruses, from the decades-old Influenza virus to the more recent SARS-CoV-2 virus. Recently Sengupta et al., for the very first time, reviewed graphene-based LOC devices to detect viruses. This review covers the widest variety of viruses detected by graphene-based LOC devices, including SARS-COV.137 Because this section covers most of the current advancements in microfluidic devices for virus detection, we'd want to look into some recent studies that Sengupta et al. did not include. Recently, graphene-based microfluidic sensors were successfully used to detect SARS-CoV-2, prompting researchers to examine current advancements in graphene-based microfluidic platforms for Covid-19 detection. As a result, the major thrust after 2019 was in this area. A powerful urge exists to create a specific and sensitive assay for detecting antibodies to SARS-CoV-2 virus within minutes, if not seconds; preferably, within days of infection, which would be especially valuable in medically deprived areas if the reading could be done on a smartphone. For identifying infections or antibodies, electrochemical sensing is a potential approach. In this method, electrochemical transduction is employed to detect the formation of antibody–antigen complexes. Aside from assay and antigen protocols, the shape and surface chemistry of the electrodes employed in an electrochemical cell have an impact on specificity, sensitivity, and detection time. Ali et al. effectively created a 3D device geometries with high aspect ratio ratios for electrodes for covid-19 detection in compared to a 2D planar construction. This device is built by employing 3D-printing gold micropillar array electrodes with an Aerosol Jet nanoparticle, then functionalizing rGO nanoflakes and immobilizing antigens over the electrode surface with an NHS: EDC chemistry. Electrochemical transduction was used to detect antibodies in the fluid by creating an immunocomplex with antigens over the surface of the 3D electrode. Antibodies against the S1 virus spike and antigen RBD were found at analytical sensitivity levels of 1 × 10−12 and 1 × 10−15 m, respectively. This device is generic, and it can detect biomarkers for various infections such as Zika, Ebola and HIV.138 Sindre et al. developed a label-free biosensor for sensitive, fast, label-free, and amplification-free detection of SARS-CoV-2 using graphene as a working electrode integrated onto a flex printed circuit board (FPCB). Here SARS-CoV-2 capture ssDNA sequence was coupled with biotin to create a biotinylated probe, which was then fixed on a graphene surface modified with streptavidin. The hybridization of target DNA was accomplished using the sensing matrix that was constructed. A user-friendly reservoir chamber module was also built to convey the sample solution to the FPCB device's working electrode. This sensor's effective design boosted its sensitivity and selectivity, allowing it to detect DNA at concentrations ranging from 100 fg mL−1 to 1 g mL−1. With a small amount of material, the electrochemical method paired with FPCB allowed for quick identification of the target sequence (5–20 L). They were able to get a LOD of roughly 100 fg mL−1.139 Another label-free impedance biosensor utilizing the screen-printing method was reported by Ehsan et al. to detect SARS-CoV-2. They demonstrated a low-cost batch production process for a cellulose paper substrate. A highly conductive graphene/carbon ink mixture was screen-printed as a working electrode to immobilize with IgG antibodies in various formats. These formats include 1-pyrenebutanoic acid succinimidyl ester (PBASE) direct format and staphylococcal protein A (Prot A) controlled format shown in Fig. 10, which were both characterized using electrochemical techniques. Fig. 10 depicts the EIS responses of this sensor, where both formats show a rise in the Rct semicircle in the Nyquist plot when the concentration of spike protein increases. However, in the case of Prot A format, the linear range was enlarged three times in magnitude of concentration, which can be attributed to the antibody's ordered orientation via Prot A modifications. The EIS sensors given here can measure concentrations as low as 0.25 fg mL−1, allowing early identification and isolation of the sick person.140
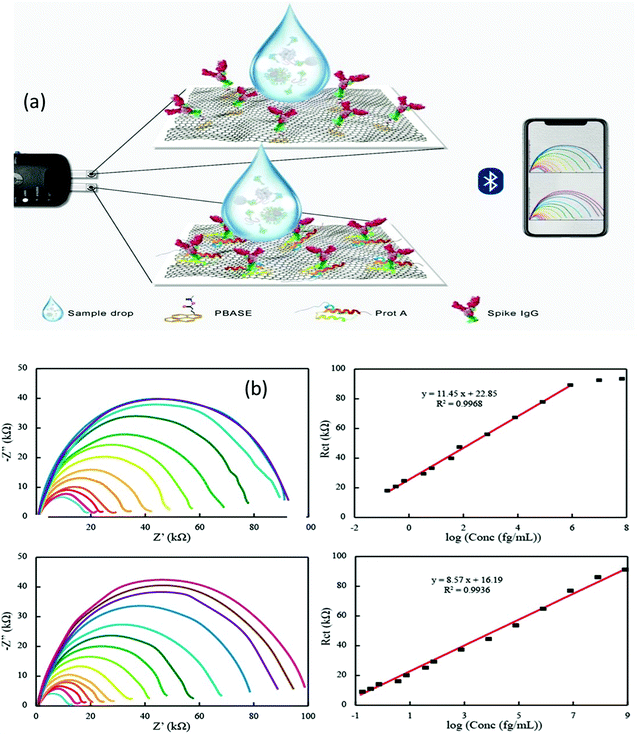 |
| Fig. 10 (a) Schematic illustration of the fabricated sensor (b) sensor response and corresponding calibration plot using PBASE-mediated and ProtA-mediated antibody immobilized electrode on graphene. Source: reprinted from M. A. Ehsan, S. A. Khan, A. Rehman, Screen-Printed Graphene/Carbon Electrodes on Paper Substrates as Impedance Sensors for Detection of Coronavirus in Nasopharyngeal Fluid Samples, Diagnostics., 2021, 11(6), 1030. | |
Other recent articles on graphene-based microfluidic and LOC sensors for viral detection focus on Hepatitis B virus detection (HBV). Because of the high death rates linked with cirrhosis, chronic hepatitis and liver cancer, this is a chronic viral infection that is a serious worldwide health problem. Teengam et al. created a portable immunosensing sensor based on a smartphone that was successful in detecting HBV. The level of HBsAg was measured via amperometric detection and wireless communication via a card-sized NFC potentiostat. The HBsAg level was quantified by amperometric detection using a card-sized NFC potentiostat as wireless communication. This sensor's working electrode was built of screen-printed graphene (SPGE) that has been modified with gold (Au) nanoparticles to improve sensitivity, and β-cyclodextrin (β-CD) was electropolymerized on the surface of Au coated SPGE to trap antibodies. The LOD and linear calibration curve for HBsAg were determined to be 0.17 μg mL−1 and 10–200 μg mL−1.141 They were utilizing the advantages of Electrochemical paper-based devices (ePADs). Boonkaew et al. fabricated an ePAD for sequential immunosensing fluid administration by combining dual flow characteristics (delayed/fast) into a single paper device for simultaneous determination of hepatitis C core antigen (HCVcAg) and hepatitis B surface antigen (HBsAg). The redox reagent was stored in a delayed channel for future electrochemical experiments, while unbound antigens were washed automatically in a fast-flow channel. As a result, the user is unlikely to need the inconvenient multi-step reagent manipulation technique. The suggested ePAD had a LOD of 1.19 pg mL−1 for HCVcAg and 18.2 pg mL−1 for HBsAg.142
2.2 For chemical analysis
Pumera and his colleagues employed graphene-based nanoparticles in LOC devices for electrochemical detection.143 However, they could not identify any advantages to using GO as an electrochemical sensor in a microfluidics system at the outset.144 Later, they realized that graphene that had been electrochemically reduced could be utilized as a sensor with excellent results. They also suggested that utilizing a graphene-based electrochemical sensor could increase the detection limits of other nanomaterials (such as silver nanoparticles).145 Pungjunun et al. created an electrochemical paper-based device to detect lead Pb(II) and tin Sn(II) in canned foods. They made a sensor based on bismuth nanoparticles (BiNPs)/screen-printed graphene electrodes because nanocomposite containing metal nanoparticles and graphene improves the performances of electrochemical sensors synergistically. Because their oxidation potentials are near to each other, simultaneous detection of Pb(II) and Sn(II) results in an overlapping peak. They were able to improve the peak separation between these peaks as well as the signal by adding the surfactant cetyltrimethylammonium bromide (CTAB). Under ideal conditions, both metals have a linear range of 10–250 ng mL−1, and the computed LOD (3SD/slope) for Pb(II) and Sn(II) was 0.44 ng mL−1 and 0.26 ng mL−1, correspondingly.146 On-chip optical gas sensors that use resonance shifts of cavities to measure molecule concentrations provide high sensitivity, real-time detection, and a tiny footprint. However, such sensors are typically hampered by a considerable cross-sensitivity problem induced by environmental temperature variations. To overcome this limitation, Wang et al. studied a dual-mode graphene-on-microring resonator that could precisely quantify gas concentrations while being unaffected by temperature fluctuations. To be more explicit, the modal linear independent responses may be employed to decouple the effects of external temperature variations and gas-induced graphene optical conductivity changes on the effective refractive indices of TE0 and TE1 modes in the resonator. Using this technology, they developed a nitrogen dioxide (NO2) sensor with LOD around 0.5 ppm and sensitivity around 0.02 nm ppm−1. Their research lays the path for developing high-sensitivity, temperature-stable on-chip optical gas sensors.147
3. MXene-based microfluidic sensors
MXenes and their hybrids have shown a great deal of promise in the realm of flexible, portable electronics, such as wearable sensors and microfluidic chips. Because of its flexibility and robust laminar structure, MXene could be easily integrated into microfluidic chips and wearable sensors.148 Sensing electrodes are crucial in the creation of wearable sensors that use the electrochemical technique. In wearing technology, traditional metal-based electrodes have been replaced by innovative 2D materials like MXene to increase sensor performance by expanding contact area and adding appropriate electrical and mechanical qualities.149
3.1 MXene-based microfluidic biosensors
3.1.1 For biomolecules detection.
Electrochemical biosensors built using the MXene-incorporated microfluidic chip are relatively new, yet they are quickly gaining popularity. Li et al. used an alternate approach, a cellulose-paper-based substrate, to create a highly integrated sensing (HIS) paper to detect lactate and glucose in sweat. They made this sensor utilizing a screen-printing approach using MXene/Methylene blue as the active material and foldable cellulose substrates as sweat analysis pads. This sensor's 3D layout optimizes sweat absorption by limiting fluid gathering and thereby lowering user discomfort. The fabricated device was made up of a sweat adsorbing vortex-patterned layer (the layer in touch with the skin), a 3D electrode layer, and a fast diffusion layer where the independent 3D position of three-electrode helps in gaining stability. After folding the aforementioned HIS paper-based substrate into a multi-layer structure, a well-designed 3D diffusion channel was created by linking hydrophilic regions of each layer. MXene-incorporated paper-based sensors are pretty unfamiliar and rapidly emerging. This is the first time such a revolutionary dual-channel system has been reported. With a sensitivity of 0.49 μA mM−1 and 2.4 nA μM−1, this HIS electrochemical sensor can detect lactate and glucose at the same time. MXene acts as a highly sensitive interface, in this case, allowing the biomolecule to be immobilized. In contrast, its modification with MB allows for charge migration and improves the sensor's sensitivity.150
Zhang et al. reported a portable electrochemical patch sensor for real-time monitoring of potassium [K+] ion concentration in human sweat. They used a layer-by-layer deposition method over PET substrate to fabricate this unique all-solid-state [K+] ion-selective electrode based on MXene/MWCNT hybrid structure. For real-time detection of [K+] ions, this flexible patch sensor was coupled into a battery-free RF energy harvesting Near Field Communication (NFC) wireless patch system. In this electrochemical patch sensor, a microfluidic channel was constructed utilizing 3D printing technology to collect human sweat and reduce sensor surface contamination. This sensor showed a sensitivity of roughly 63 mV dec−1, which may be amplified to 173 mV dec−1 utilizing NFC, with a linearity of 1 to 32 mM.151
Another electrochemical microfluidic device for the analysis of creatinine, uric acid, and urea in whole blood was disclosed by Liu et al., which can be used to assess bodily conditions during hemodialysis shown in Fig. 11a. This four-layered microfluidic device was created for continuous and direct multicomponent analysis of whole blood. A blood flow channel was on the top layer, a dialysis membrane was on the second layer, an isotonic solution channel was on the third layer, and a detecting chamber with a sensing electrode was on the bottom layer (screen printed MXene). To enhance the efficiency of this microfluidic electrochemical sensor, methylene blue (MB) was loaded onto this screen-printed electrode following immobilization with urease with the help of glutaraldehyde for urea measurement. The simultaneous detection of many analytes was achieved thanks to the synergistic effects of MXene electrocatalysis, enzymes immobilized on MXene and probes/ions adsorbed by MXene nano-sheets. The microfluidic chip was carefully designed to suit the requirements of complete blood analysis while also allowing for easy electrode replacement for long-term application. This chip was utilized to test the renal function in 105 healthy persons, with a gold standard being a conventional biochemical analysis apparatus. The content of Cre, urea and UA obtained by the chip method matched that obtained by the golden approach, and there was no statistical difference between the two procedures. The electrocatalytic mechanism of UA in the sensor is depicted in Fig. 11b. MXene adsorbs UA molecules, which form hydrogen bonds with the functional groups of MXene, increasing the effective concentration of UA at the electrode/electrolyte interface for subsequent electrocatalysis. In the instance of Cr shown in Fig. 11c, the Cre molecules will be entirely chelated with Cu ions, especially in the presence of excess copper (Cu) ions, and adsorption on MXene will be mediated by electrostatic interactions.17
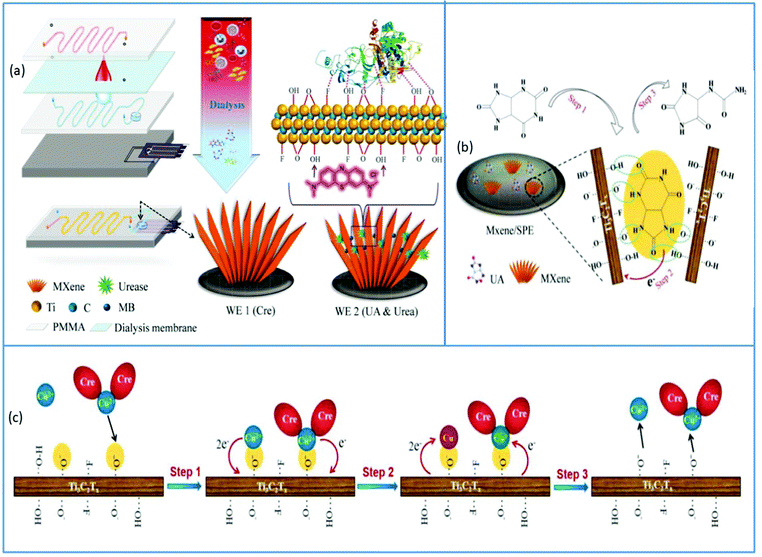 |
| Fig. 11 (a) Schematic illustration of the fabrication of MXene based microfluidic sensor (b) UA detection mechanism and (c) creatinine detection mechanism. Reprinted with J. Liu, X. Jiang, R. Zhang, Y. Zhang, L. Wu, W. Lu, et al., MXene-Enabled Electrochemical Microfluidic Biosensor: Applications toward Multicomponent Continuous Monitoring in Whole Blood, Adv. Funct. Mater., 2019, 29(6), 1807326. with permission from John Wiley and Sons. | |
3.2 For chemical analysis
Because of its inherent high hydrophilicity, chemical composition, and robust electrochemical nature, MXene-based sensors are a viable option for pollution detection.152 The potential applicability of MXene-based sensors for environmental applications has been described in a vast number of reviews. MXene based Microfluidic sensors for environmental applications, on the other hand, are still in their infancy. Yang et al. revealed the first on-chip SERS sensor for gas detection. The use of SERS in conjunction with microfluidic technology aids in detecting a tiny trace of gases. The microfluidic channel can consistently control small gas flow, which helps to overcome the reproducibility concerns plaguing SERS. Extra adsorption materials with high surface areas are the most popular technique for improving the efficiency of a gas sensor. However, when it comes to microfluidic sensors, such materials restrict the analyte away from the SERS hotspot while simultaneously interfering with the microflow. As a solution, they created a honeycomb-like 3D substrate using layer-by-layer colloidal self-assembly, which was then translated into a microfluidic chip using a process they devised. By permitting in situ vortices in the SERS hot zone to raise detection sensitivity by increasing molecule residence time. Following this, an ultra-flexible MXene adhered onto this 3D substrate that serves as a universal adsorption layer for various VOCs (i.e., explosives-related indole, odoriferous benzaldehyde and 2,4-dinitrotoluene (DNT)). Compared to typical lithography-fabricated plasmon-based sensors, this transfer method helps to minimize manufacturing costs, which is desirable for practical application. They employed a standard least-square analysis (CLS) approach to split the SERS spectrum and identify the actual concentration of each constituent utilizing chromatic barcodes to determine the accurate concentration of these VOCs. This microfluidic sensor exhibited LOD of around 10 and 50 ppb for DNT and indole.153
4. TMD-based microfluidic sensors
Many nanomaterial-based detectors have been incorporated into microfluidic systems to improve sensing efficiency, sensitivity, and detection limits.154 TMDs with unique electrochemical properties, on the other hand, is gaining popularity due to their atomically thin layered 2D architectures, which allow for easy device fabrication and patterning. Graphene and graphene derivative nanomaterials have been studied extensively to offer ultra-sensitive detection in microfluidic devices, especially lab-on-chip systems.155 TMDs also have 2D layered structure compared to graphene with appealing features such as capacitive solid behavior, superior conductivity, and a high surface-to-volume ratio, making them promising candidates for increasing microfluidic device performance.155
4.1 TMD-based microfluidic biosensors
4.1.1 For biomolecules detection.
Recently, in fluorometric biosensors, many of the nanomaterials have been reported as nanoquenchers due to their high quenching efficiencies, high surface area and good biocompatibilities, such as graphene oxide,156 gold nanoparticles157 and carbon nanotubes.158 Very recently, MoS2 nanosheets were reported as efficient dye quenchers because of their ability to spontaneously absorb single-stranded DNA (ssDNA) using van der Waals force between the nucleobases and basal plane MoS2, displaying high fluorescence quenching ability, which was used for small molecules and DNA detection.159 The quenching ability of MoS2 is unstable and would be impacted by oxygen and moisture present in the atmosphere.160 Besides, like graphene, the electrochemically active sites can be significantly reduced due to its aggregation tendency in practical applications.161 It is critical to conduct studies as soon as possible after synthesizing MoS2 solution, which isn't appropriate for a typical DNA test.162 However, the combination of other materials with MoS2 may circumvent this constraint. A novel microfluidic biosensor based on MoS2/MWCNT nanocomposite with 3D architecture was fabricated by yang et al. for the detection of DNA molecules. When compared to MoS2, the generated MoS2/MWCNT aqueous solution combined with microfluidics shown high quenching stability for over 75 days, which is acceptable for a regular DNA test. The microfluidic channels greatly reduce the sample volume and so ∼fmol DNA detection can be displayed with a detectable fluorescence color in a matter of minutes using the microfluidic assay. The constructed sensor had a linear range of 0–50 nM and could detect 1 nM DNA. It enables high-throughput DNA analysis in a simple and quick manner. The large scale sensing studies of DNA could be possible by using the strong quenching stability of MoS2/MWCNT aqueous system connected with microfluidics, which reduced the time for sensing studies and thereby describing that MoS2/MWCNT can be a favorable nanocomposite for fluorimetric sensing.162
A little amount of research has been conducted to investigate the use of TMDs in increasing the performance of analytical LOC microfluidic devices. Considering these facts, Toh et al. presented simple, cost-effective and sensitive fabrication of LOC electrochemical microfluidic devices for H2O2 detection. They integrated group 6 2D layered TMDs (MoS2, WS2, MoSe2 and WSe2) prepared by t-BuLi exfoliation as detector surfaces inside the LOC devices via drop-casting method and evaluated their response for heme-based H2O2 biosensor. The high activity of the 1T phase is related to the high density of active catalytic sites on both edges and basal plane, high conductivity, capacitive behavior and high electrical conductivity, making them excellent for sensing applications. The exfoliation with t-BuLi generates single to few-layered TMDs containing metallic 1T phase. Fig. 12a depicts the chronoamperometric reactions of this 1T-phase WS2 to varied H2O2 concentrations. The reduction currents observed with the 1T-phase WS2-based LOC device outperformed the other 2D TMD materials. They demonstrated superior electrocatalytic activity, a LOD of 2.0 nM, a wide range of linear responses (20 μM to 20 nM and 2 mM to 100 nM), and good selectivity for real-time analysis.155
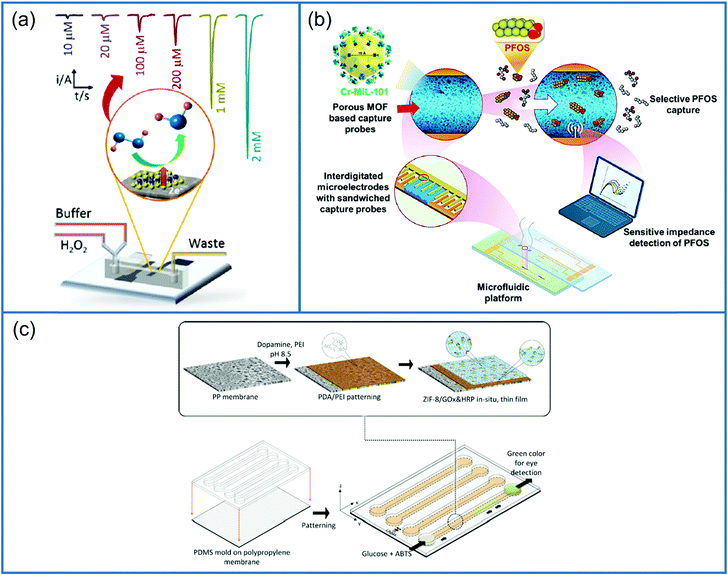 |
| Fig. 12 (a) LOC based on TMD material for detection of H2O2. Reprinted with R. J. Toh, C. C. Mayorga-Martinez, J. Han, Z. Sofer, M. Pumera, Group 6 Layered Transition-Metal Dichalcogenides in Lab-on-a-Chip Devices: 1T-Phase WS2 for Microfluidics Non-Enzymatic Detection of Hydrogen Peroxide, Anal Chem., 2017, 89(9), 4978–4985. (2020) American chemical society. (b) Schematic of perfluorooctanesulfonate (PFOS) detection using MOF based microfluidic sensors reprinted with Y. H. Cheng, D. Barpaga, J. A. Soltis, V. Shutthanandan, R. Kargupta, K. S. Han, et al., Metal–Organic Framework-Based Microfluidic Impedance Sensor Platform for Ultrasensitive Detection of Perfluorooctanesulfonate, ACS Appl. Mater. Interfaces., 2020, 12(9), 10503–10514. Permission from (2020) American chemical society. (c) Fabrication of bio-composite containing MOF patterning reprinted with W. Qi, L. Zheng, S. Wang, F. Huang, Y. Liu, H. Jiang, et al., A microfluidic biosensor for rapid and automatic detection of Salmonella using metal–organic framework and Raspberry Pi, Biosens. Bioelectron., 2021, 178, 113020. Permission from (2020) American chemical society. | |
4.1.2 For pathogen detection.
Salmonella is a food-borne pathogen, the causative agent of paratyphoid A fever and poses a severe threat to human health.163 Several techniques, including molecular and immunological, for detecting Salmonella, but these methods face limitations such as expensive reagents and cross-reactivity.164 The electrochemical process could overcome all the rules associated with traditional methods. Besides, in this method, a biological recognition element was also used to directly bind with Salmonella and change the electrochemical signal.165 2D materials have recently piqued researchers' interest because of their stability, electron transfer kinetics, and large surface area.166 Because of its large surface area, MoS2 has sparked a lot of attention, although it has low conductivity.167 Compared to graphene with a too tiny bandgap, the bandgap in 2D MoS2 enables a multifold increase in device sensitivity.168 The proper tuning of the bandgap in 2D MoS2 is possible via several methods, whereas in graphene, the modulation of the electronic band structure is complex.169 Furthermore, when 2D MoS2 is changed into tetragonal symmetry, its inherent electronic energy state changes from semiconducting towards conducting, making it appropriate for bioanalyte detection using electrochemical techniques.170 Furthermore, compared to other 2D materials such as graphene, which do not give excellent electrical and chemical properties, 2D MoS2 is compatible with the typical electrochemical system.171 Because the 2D electron–electron correlations between Mo atoms promote the planar electron transfer behavior, MoS2 with layered structures has distinct catalytic and electrochemical properties in perpendicular and plane directions, resulting in differences in electrochemical behaviors the edge and basal planes.172,173 Singh et al. have designed positively charged MoS2 nanosheets functionalized with cetyltrimethyl ammonium bromide for protein conjugation over microfluidics electrode to detect Salmonella typhimurium. Because CTAB provides a positive charge on MoS2 sheets that forbid aggregation by countering the cohesive energy amid exfoliated layers, surfactant-assisted exfoliation for the production of MoS2 could enable stabilization of exfoliated 2D MoS2. CTAB-MoS2 NS deposited the patterned indium titanium dioxide ITO microelectrode using the firm electrostatic contact of positively charged CTAB-MoS2 NS. After this, a Lab on a Chip platform was developed by incorporating microelectrode with the PDMS microfluidic device for S. typhimurium detection via electrochemical impedance technique. The developed microfluidic immunosensor displayed excellent sensing parameters such as sensitivity 1.79 kΩ CFU−1 mL−1 cm−2, the wide linear range of 101 to 107 CFU mL−1 and LOD of 1.56 CFU mL−1.174
5. MOF-based microfluidic sensors
MOF-based biosensors have several advantages, such as porous structures, high catalytic activities, large specific areas, and ease of functionalization for developing highly efficient sensors. Despite the recent progress in preparing and applying different types of MOFs, the environmentally friendly synthesis by controlling their sizes and shapes remains a challenge. Furthermore, the lack of ease in operation, portability, integration and rapid assays in synthesized MOF biosensors limits their broad applicability in environmental analysis, biosensing, and POC detection, particularly in low resource situations. This versatile platform allows for rapid analysis, integrated processing, high portability and analysis of complex biological fluids with high sensitivity, efficiency and multiplexed detection. Among these advantages, microfluidics offers a unique chance for MOFs in controlled synthesis and sensing applications.175,176
5.1 MOF-based microfluidic bisensors
5.1.1 For biomolecules detection.
Impressively, most of the MOF limitations are the advantages of microfluidic systems. The integration of MOF-based biosensors on a microfluidic device can accompany each component providing huge potential for joined high-performance molecular detection in different areas. Researchers in past years have ventured to combine MOFs on microfluidics to develop biosensors benefiting from both MOFs and microfluidics. MOFs can be placed within microfluidic channels due to high pore volume, surface area and large functional groups; the MOFs-based microfluidic sensor can display high analytical performance. Increasing enzyme stability without affecting their activity is possible through biomineralization of enzyme in metal–organic-frame work (enzyme-MOF) composite, which has demonstrated great promise in biosensing devices.176 Mohammed et al. fabricated a microfluidic biosensor based on MOFs and enzymes for glucose detection. However, many enzyme-MOF composites have focused on encapsulating enzyme particles, limiting synthesis flexibility for real-world applications due to the requirement for post-synthesis immobilization over solid support. Hence, the surface patterning and microfluidic system integration offer various advantages for developing a diagnostic sensing device based on the biomineralized enzyme. Polydopamine/polyethyleneimine coating was explored to pattern MOFs/enzymes in microfluidic channels for biosensors assembly in a PDMS-based microfluidic device. Fig. 12c depicts a schematic diagram of the production of bio-composite patterning. They used a cascade reaction of glucose oxidase and HRP enzymes on imidazole zeolitic framework-8 thin film to detect glucose. The ZIF-8/GOx&HRP in situ displayed excellent selectivity and limit of detection at 8 μM with a wide concentration range from 8 μM to 5 mM for glucose detection. Initially, microfluidic devices were developed from PDMS or glass were followed by other polymeric materials such as PVA (poly vinyl alcohol), PMMA (polymethyl methacrylate), polystyrene, thereby widening the scope of microfluidic systems.176 Since 2007, microfluidic paper-based sensors have emerged as a potential platform for several applications, including resource-limited settings and POC diagnostics.177–179 Paper as a microfluidic platform offers various benefits over previously employed MEMS-based nanomaterials because of its ability to absorb aqueous fluids without active pumping, lightweight, thin, transport, easy to store, stack and compatible with biological samples and low cost. In 2019 Ilacas et al. developed MOF (encapsulate GOx) based colorimetric sensor on two microfluidic paper platforms, one lateral flow assay based and the other based on the well configuration for glucose detection, followed Michaelis Menten enzyme kinetics. Furthermore, the LOD differs between platforms; experiments with the LFA-based platform showed sensitivity after 0.5 mM glucose, whereas the well-based μPAD was 0.25 mmoL−1. This well-based μPAD platform is constructed of laminate sheets and multiple layers of wax-printed chromatography paper. The GOx@MOFs species placed between the paper layers reacted with the glucose and KI solutions flowing through the device, producing a yellow-brown color. This device was dried, scanned, and examined, and a relationship between glucose concentrations and average inverse yellow intensity was discovered.180 The devices created using MOFs as biomimetic catalysts should broaden the scope of microfluidic technologies for multiplex analyte detection.
5.1.2 For pathogen detection.
The prevention of food poisoning is possible through the rapid screening of contaminated food. But the time-consuming cell culture prevents on-site detection using currently available bacterial detection methods; Eliza lacks sensitivity and PCR requires complex DNA extraction. Qi et al. fabricated a microfluidic biosensor based on raspberry pi and metal–organic framework NH2-MIL-101 (Fe) with biological signal amplification via mimic peroxidase activity with a self-developed app to evaluate the color image data for the rapid, automatic and sensitive detection of salmonella. These MNB–Salmonella–MOF complexes were prepared using the simultaneous conjugation of target cells with anti-salmonella polyclonal antibodies modified MOFs and anti-salmonella monoclonal antibodies, which were further modified using immune magnetic nanobeads (MNBs) in a microfluidic chip. The complex catalyzed the mixture of H2O2 and OPD (o-phenylenediamine) to form yellow DAP (2,3-diaminophenazine). Finally, the bacterial concentration was calculated from the catalyst (DAP) analysis gathered under narrow-band blue light using a raspberry app. The outcome displayed outstanding ability to detect salmonella with a LOD of 14 CFU mL−1 and concentration ranging from 1.5 × 101 to 1.5 × 107 CFU mL−1.181 This microfluidic biosensor was capable of mixing, labeling, separating and detection. It demonstrated the advantages of using fewer reagents, being small in size, having a quick reaction time, and operating automatically to detect foodborne bacteria on-site.
5.2 For chemical analysis
The demand for sensing techniques that simultaneously provide high selectivity and sensitivity has increased because of public exposure to environmental, biological and chemical contamination from anthropogenic or natural sources and intentional chem-bio threats.182 Developing techniques for highly selective, ultra-sensitive, and rapid in situ identification and quantitation of target contaminants could open up new technical and fundamental possibilities in biological/chemical/environmental/radiological forensics, as well as earlier disease detection and diagnostics and POCT applications. It facilitated the development of affinity-based analytical sensors with two crucial processes in target detection: (1) selective and preferential recognition of target from a sample matrix via particular affinity-based interactions; (2) the conversion of a target binding process into a measurable spectroscopic, magnetic or electrochemical response in a transduction step.183 In light of these findings, a microfluidic impedance sensor based on MOF was developed for in situ detection of perfluorooctanesulfonate (PFOS). PFOS is a severe environmental pollutant that belongs to the per and polyfluoroalkyl substrates class of anthropogenic compounds. Their widespread civilian and industrial use has dramatically increased their earth's abundance. Schematics of PFOS detection are shown in Fig. 12b. This study used a synergic technique for affinity-based target capture using a porous sorbent probe placed on a microfluidic platform to boost detection sensitivity. A probe made of MOF Cr-MIL-101 with high pore volume and surface area was used for detection of PFOS based on the chromium center affinity toward both sulfonate functionalities as well as fluorine tail groups. This MOF based sensor integrated with microfluidic platform displayed ultrahigh sensitivity for the in situ detection of PFOS, the LOD was observed as 0.5 ng L−1, which is similar to that of advanced ex situ techniques.184
6. Other 2D materials-based microfluidic sensors
The thermodynamically most stable form among the phosphorus allotropes is known to be phosphorene or black phosphorus. It is one of the potential candidates for biomedical applications because of its biocompatibility. Degradation is the major obstacle in using phosphorene in spite of its attractive advantages. The lone pair of electrons present in phosphorene reacts with O2 to form an oxide that readily reacts with water to form phosphoric acid, this leads to Phosphorene degradation.185 Marine algal blooms often grow during the summer months. Among these phytoplankton comes under harmful algal blooms (HABs) generate chemically stable compounds which adversely affect human health. Okadaic acid is a group of biotoxins that originate as a byproduct of HAB. It is a polyether fatty acid derivative and has been described as a major toxic compound in Diarrheic Shellfish Poisoning (DSP). In accordance with European standards, the maximum threshold level for OA in seafood is limited to 160 μg kg−1. This low permissible level has prompted researchers to find out effective detection techniques. Ramalingam et al. have developed an electrochemical microfluidic biochip for the monitoring of OA. The aptamer-specific OA was immobilized on the screen-printed carbon electrode modified with phosphorene-gold nanocomposite synthesized without a reducing agent via an in situ one-step method. The signal strength was quantified using a redox pair of potassium ferro–ferri cyanide. The fabricated microfluidic platform improved the sensitivity, portability, and reaction time. The developed device consisted of specific channels for sample mixing and incubation. Altogether, the integrated system comprised of a PDMS microfluidic chip covering an aptamer modified SPCE, as a single detection module for Okadaic acid. The differential pulse voltammograms displayed a detection limit of 8 pM with a linear range between 10—250 nM. The fabricated POCT device can be used to perform on-farm assays in fishing units.186
Silicon can also form as a 2D honeycomb lattice like graphene, and it is named as silicene, both forms have some structural similarities. Even though, the two differ in various aspects including the presene of most stable buckled structure of silicene instead of planar structure in graphene and non-applicable exfoliation method in silicene synthesis because this allotrope does not freely exist in nature. So, the popular method to prepare silicene is to deposit Si atoms on a metal substrate. Amorim et al. presented a theoretical study regarding the interaction between silicene and DNA nucleobases and suggested a possible application as a biosensor. The studies demonstrated that the thymine and adenine are physisorbed on silicene instead of guanine and cytosine, which are poorly chemisorbed via the formation of a Si–O bond. Besides, they studied the adsorption of DNA based on the electronic properties of silicene, explaining that it is possible to distinguish an electronic signal due to the strong interaction of guanine and cytosine with silicene from the poor interaction of adenine and thymine. The non-equillibrium Greens function method is used to explore changes in in the transmission and the conductance caused by adsorption of each nucleobase. These findings suggested that silicene could be explored as an integrated circuit biosensor as part of a LOC device for DNA sequencing.187
The nanomaterials integrated on the biosensors have shown better stability as well as electrical and chemical properties because the integration further amplify the sensor response which improves the selectivity and sensitivity of the biosensor. The semiconducting 2D g-C3N4 has displayed various advantages such as large surface area, better electrochemical reactions, excellent chemical stability, cost effectiveness and a good source of nitrogen.188 The presence of nitrogen improves the features of electron donor/acceptor, increases the wettability with the electrolytes, together with the supply of a large additional pseudocapacitance189 and exceptional chemical consistency. Paper electrodes are effective over screen printed electrodes as they are easily manufactured and cheaper, so making them appropriate for mass production. They do not corrode easily because they are unaffected by the conditions of the external environment, unlike the screen-printed ones. Norovirus is one of the major causes of intense diarrhoea, high fever, acute pain in the stomach and gastroenteritis. Traditional method of monitoring gave us highly promising results but had disadvantages including cost effectiveness, selectivity, sensitivity, and reduced specificity. Rana et al. were chosen graphitic carbon nitride (g-C3N4) particles for the interface of the paper electrodes. An electrochemical biosensor comprised of an electrochemical paper-based analytical device (ePAD) modified with oxidized graphitic carbon nitride (Ox-g-C3N4) was designed for the detection of norovirus DNA. The capture probe DNA (PDNA) modified electrodes were analyzed by CV and DPV, a limit of detection of 100 fM was observed. A non-complementary DNA was introduced on the ePAD modified using oxidized graphitic carbon nitride for testing the response of probe DNA in order to confirm the specificity of the designed biosensor. The developed ePAD sensor is simple, specific and needs a minimum analysis time of 5s.190 The performance of microfluidic biosensors based on 2D materials is shown in Table 2.
Table 2 Microfluidic devices based on two-dimensional materials
Target/analyte |
Detection principle |
Sensing material |
Substrate |
LOD |
Ref. |
Graphene based microfluidic sensors |
Multiple biomarkers for the early detection of ovarian carcinoma |
SERS |
Graphene oxide |
Silicon wafer |
1 pg mL−1 and 0.01 U mL−1 |
88
|
Epidermal growth factor receptor (EGFR) |
CV and DPV method |
Amino-functionalized graphene (NH2-GO)/thionine (THI)/gold particle (AuNP) |
Paper-based substrate |
5 pg mL−1 |
91
|
Pseudopodium-enriched atypical kinase one, SGK269 (PEAK1) biomarkers of pancreatic cancer |
CV and DPV method |
Graphene oxide |
Paper-based substrate |
10 pg mL−1 |
92
|
microRNAs (miRNA-155 and miRNA-21) biomarkers of lung cancer |
DPV |
Rgo or MoS2 nanosheet decorated with gold nanoparticles |
Paper-based substrate |
|
AuNPs/RGO |
|
AuNPs/MoS2 |
93
|
|
|
|
|
miRNA-21 |
12 nM |
|
51.6 nM |
|
|
|
|
|
miRNA-155 |
25.7 nM |
|
59.6 nM |
|
Carcinoembryonic antigen (CEA) detection |
Impedance studies |
Graphene-PEDOT:PSS modified |
Paper based substrate |
1.06 ng mL−1 in human serum samples |
96
|
Carcinoma antigen 125 |
CV, DPV and impedance studies |
Au-boron-doped graphene (BG)/boron-doped diamond (BDD) composite film |
VBG/BDD |
0.15 pg mL−1 |
100
|
BRCA1 and BRCA2 mutation genes |
Attenuated total reflection (ATR) |
Graphene coated fiber-optic SPR biosensor |
Fiber |
— |
104
|
ssDNA detection |
Transfer characteristics |
Graphene |
Lab-on-printed circuit board |
1 nm |
110
|
Thrombin protein biomarker |
SPR |
Cys-Rgo hydrogel |
PDMS |
4 pg mL−1 |
113
|
Glucose |
Electrochemical studies |
Laser induce graphene (LIG) |
PET |
— |
118
|
Glucose and H2O2 |
Electrochemiluminescence (ECL) |
Laser induce graphene (LIG) |
PI |
Glucose |
H2O2 |
119
|
|
|
|
|
0.138 μM |
5.8729 μM |
|
Vitamin B12, Vitamin C and H2O2 |
Electrochemiluminescence (ECL) |
Laser induce graphene (LIG) |
PI |
Vitamin B12 |
Vitamin C |
H2O2 |
120
|
|
|
|
|
0.109 nM |
0.96 M |
0.303 M |
|
H2O2, glucose, dopamine and xanthine |
Electrochemiluminescence (ECL) |
Laser induce graphene (LIG) |
PI |
H2O2 |
Glucose |
Dopamine |
Xanthine |
121
|
|
|
|
|
1.71 μM |
3.76 μM |
3.40 μM |
1.25 μM |
|
D-Glucose, H2O2, choline and lactate |
Electrochemiluminescence (ECL) |
Laser induce graphene (LIG) |
PI |
D-Glucose |
H2O2 |
Choline |
Lactate |
122
|
|
|
|
|
2.51 μM |
4.36 μM |
4.01 M |
5.32 μM |
|
Paracetamol and glucose |
Impedance studies |
Chitosan/GO modified PAD |
Paper based substrate |
— |
124
|
Glucose |
Electrochemical studies |
Prussian blue-deposited rGO-tetraethylene pentamine (PB/rGO-TEPA) |
Paper based substrate |
25 μM |
125
|
Glucose |
Transfer characteristics |
Pyrene-1-boronic acid (PBA) functionalized graphene |
PDMS |
0.1 μM |
126
|
L-Lactic acid |
Transfer characteristic studies |
Graphene-based transistor (GFET) |
PDMS |
— |
127
|
Glucose |
Electrochemical studies |
Graphene modified with PB |
Flexible polyester sheets |
— |
128
|
Cystatin C |
Electrochemical studies |
GO-chitosan (GO-Chit) |
Paper-based substrate |
2.6 pg μL−1 |
133
|
Uric acid and thyrosine |
Electrochemical studies |
Laser engraved graphene |
Polyimide |
UA |
|
Tyr |
|
134
|
|
|
|
|
0.74 μM |
|
3.6 μM |
|
|
Ascorbic acid |
Impedance studies |
|
PVC foil |
125.01 μM |
135
|
SARS-CoV-2 |
Electrochemical transduction |
Functionalized rGO nanoflakes |
PDMS |
2.8 × 10−15 m |
138
|
SARS-CoV-2 |
Electrochemical studies |
|
|
100 fg mL−1 |
139
|
SARS-CoV-2 |
Impedance studies |
Graphene/carbon ink mixture |
Paper-based substrate |
0.25 fg mL−1 |
140
|
Hepatitis B Virus (HBV) |
Amperometric detection |
Screen-printed graphene electrode |
PVC |
0.17 μg mL−1 |
141
|
Hepatitis C core antigen (HCVcAg) and hepatitis B surface antigen (HBsAg) |
Electrochemical studies |
Graphene |
Paper-based substrate |
HCVcAg |
|
HBsAg |
|
142
|
|
|
|
|
1.19 pg mL−1 |
|
18.2 pg mL−1 |
|
|
Sn(II) and Pb(II) |
Electrochemical studies |
Bismuth nanoparticles (BiNPs)/screen printed graphene electrode |
Paper-based substrate |
Sn(II) |
|
Pb(II) |
|
146
|
|
|
|
|
0.26 ng mL−1 |
|
0.44 ng mL−1, |
|
|
|
NO2 gas |
(RI) sensors |
Graphene |
On-chip |
0.5 ppm |
147
|
|
MXene based microfluidic sensors |
Creatinine, uric acid, and urea |
Electrochemical studies |
MXene/methylene blue |
PMMA |
Creatinine |
|
Uric acid |
Urea |
17
|
|
|
|
|
1.2 × 10−6 m |
|
5 × 10−6 m |
— |
|
Glucose and lactate |
Electrochemical studies |
MXene/Prussian blue (MXene/PB) |
Ecoflex substrate |
H2O2 |
|
Glucose |
Lactate |
150
|
|
|
|
|
67 × 10−9 m |
|
0.33 × 10−6 m |
0.67 × 10−6 m |
|
Potassium [K+] ion |
Electrochemical studies |
MXene/MWCNT |
PET |
— |
|
|
|
151
|
Indole, odoriferous benzaldehyde and 2,4-dinitrotoluene (DNT) |
SERS |
|
|
Indole |
|
DDT |
|
153
|
|
|
|
|
10 ppb |
|
50 ppb |
|
|
|
TMDs based microfluidic sensors |
Salmonella typhimurium
|
CV and DPV |
CTAB-MoS2 |
PDMS |
1.56 CFU mL−1 |
174
|
DNA |
Fluorescence |
3D MoS2/MWCNT |
PDMS |
1 nM |
162
|
H2O2 |
Chronoamperometry |
1T WS2 |
PDMS |
2.0 nM |
155
|
|
MOF based microfluidic biosensors |
Glucose |
Colorimetry |
ZIF-8/GOx/HRP |
PP |
8 μM |
176
|
Glucose |
Colorimetry |
GOx Zr-PCN-222 |
Paper based substrate |
0.25 mmoL−1 |
180
|
PFOS |
Electrochemical technique |
Cr-MIL-101 |
PP |
0.5 ng L−1 |
211
|
Salmonella typhimurium
|
Colorimetry |
NH2-MIL-101 Fe |
PDMS |
14 CFU mL−1 |
181
|
Other 2D materials |
Okadaic acid |
DPV |
Phosphene |
PDMS |
8 pM |
186
|
Norovirus |
CV and DPV |
Ox-g-C3N4 |
Paper |
100 fM |
190
|
|
Microfluidic wearable biosensors based on 2D materials |
Glucose |
Photodetection |
MoS2 |
PDMS |
<0.1 mM |
195
|
Cortisol |
Electrochemical Technique |
Ti3C2Tx/LBG |
PDMS |
— |
203
|
Glucose |
Lactate |
Electrochemical Technique |
Ti3C2Tx/PB |
Ecoflex sheet |
Glucose |
|
Lactate |
|
202
|
|
|
|
|
35.3 μA Mm−1 cm−2 |
|
11.4 μA Mm−1 cm−2 |
|
|
7. Next-generation biosensors
7.1. Wearable microfluidic biosensors
Wearable biosensors have got a lot of attention from numerous fields since they allow body-worn sensors to transmit crucial data wirelessly. As a result, the Internet of Things (IoT) phenomena has spawned a plethora of opportunities. Wearables have revolutionized healthcare technology by enabling users' diagnosis and prognosis through a minute collection of physiological signals, mechano-transduced forces, or body fluids. However, this is difficult to implement due to the difficulties in recovering signals from the human body. Furthermore, the high benefit requirements on body-worn sensors need them to be flexible, lightweight, and water-resistant.191 There have been technological advancements in recent years, such as incorporating microfluidics in wearables, which are confident in their ability to overcome some of these issues. Microfluidic wearable biosensor technologies are the use of microfluidics in wearable biosensor applications. Microfluidics has numerous key-value innovations in this area. It primarily develops the sensing element's core with microstructures for liquid management or storage.192 By allowing accurate adjustment of liquid amounts, sensing accuracy and reliability can be considerably increased. This is especially beneficial because human bodily fluids are frequently released in small quantities. In this scenario, microstructures require significantly lower liquid quantities, lowering the patient's complexity. Aside from liquid handling, microstructures can also serve as physical containers for the storage and discharge solutes at regular intervals. Furthermore, the micropatterned components can act as an electrical communication route in rigid platforms. These allow for high flexibility while maintaining high electrical conductivity in microelectromechanical systems (MEMS).193 This highlights the novel concept of investigating microfluidic components and creating wearable microfluidics for continuous healthcare monitoring. Also graphene and graphene-like layered 2D materials such as MXenes, TMDs and MOF have gained immense importance as supporting substrates and transduction components in a wide variety of biosensing applications. The unique qualities of 2D materials, such as the high density of active sites over a vast surface, make them ideal for biosensing applications.194 2D materials can be tuned to display selective responses toward the target analytes with excellent sensitivity by surface chemistry modification via defect engineering or surface functionalization. 2D materials are an essential paradigm of nanomaterials for health care applications and next-generation biosensors because of their layer-dependent band structure and the ability to create heterostructures. 2D materials are useful for sensing applications that require high sensitivity because of their atomic thinness and large surface area, which result in a robust response to surface adsorption process. When combined with tunable band structure, desirable electrical properties, and a wide range of optical phenomena, these characteristics make 2D materials a strong contender for biosensing applications.194
Wearable smart contact lenses have piqued the interest of many people due to their ability to immediately monitor environmental and physiological information from the body and eyeball fluids. Smart contact lenses equipped with highly sensitive glucose sensors could pave the way for continuous non-invasive monitoring of biomarkers in tears. The integrated circuit chips or sensors must be biocompatible and stable compared to the eyeball.195 Transparent and flexible atomically thin electronic materials, such as mono-to-few-layered 2D TMDs, could be appropriate building blocks for this application. The issue, however, lies in the selection of the manufacturing technique and the same materials. Furthermore, unlike conventional circuit chips and bulk sensors, which are often sandwiched between lens substrates and coupled to eye drops via microfluidic sensing ducts,196,197 giving greater detection sensitivity and straightforward assembly without interference from blinking. Even though standard smart contact lens solutions lack biocompatibility, efficient sensor modalities, mechanical stability, and a specific fabrication method.
Contact lenses containing multifunctional sensor arrays with elastic linked nodes, PDMS lens substrate, PI passivation layers, and ultrathin TMDC semiconductors were developed to fulfill the aforementioned goals. Here, MoS2 transistors were used as core sensing material due to their layered dangling-bond-free surface, high surface area, large surface area, good biocompatibility, charge transfer properties and tunable bandgap.159,198–200 An Au-mediated exfoliation and assembly technique was used to create mono-to few-layered MoS2 sheets with a lateral dimension of 200 × 100 μm. A thin film of photo patternable PI with mechanically reinforced MoS2 transistors and the elastic parts were created using PI mesh structure and serpentine gold. The vision blocking is avoided by placing the sensor system close to the outer parts of the cornea. Mechanical analysis indicated that this design could effectively insulate devices from catastrophic mechanical deformations while maintaining high stretchability and the ability to readily transform functional components into diverse shapes of curvatures. Fig. 13a shows the structural architecture of a smart contact lens with a serpentine mesh sensor structure that is based on ultrathin MoS2 transistors. With UV illumination, these thin MoS2 transistors have a fast response of less than 1 s, high sensitivity (0.1 mM) and responsivity (4.8 A W−1) for photodetection, and a high current on/off ratio (>103).195 They also confirmed the contact lens system's light absorption to demonstrate transparency and performed in vitro cytotoxicity tests to ensure biocompatibility. Overall, the device features and material show that such contact lenses have addressed the needs for practical applications, providing a simple and new way for multipurpose contact lens sensor devices. This research creates a smart contact lens system that is very transparent, easy to use, and multipurpose. The serpentine mesh sensor device was installed directly on the lens substrate and maintained real interaction with the tears. This resulted in a more excellent performance without obstructing vision or blinking while remaining mechanically sturdy. Other functional components, such as power modules for future in vivo research, electrode arrangements for electroretinography, and antennae for wireless transmission, can be added more easily thanks to the fabrication technique and integrated contact lens sensor system.
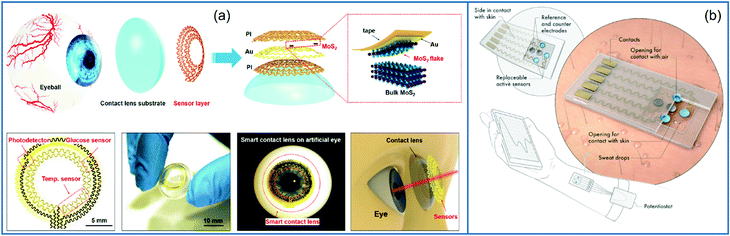 |
| Fig. 13 (a) Structure of a smart contact lens with a serpentine mesh sensor system based on ultrathin MoS2 transistors. Source reprinted from S. Guo, K. Wu, C. Li, H. Wang, Z. Sun, D. Xi, et al., Integrated contact lens sensor system based on multifunctional ultrathin MoS2 transistors, Matter., 2021, 4(3), 969–985. (b) Ti3C2Tx/PB based glucose/lactate and pH sensor developed by Lei et al. (Source: reprinted from Y. Lei, W. Zhao, Y. Zhang, Q. Jiang, J.-H. He, A. J. Baeumner, O. S. Wolfbeis, Z. L. Wang, K. N. Salama and H. N. Alshareef-, A MXene-Based Wearable Biosensor System for High-Performance In Vitro Perspiration Analysis, Small 2019, 1901190, with permission from Elsevier). | |
Electrochemical biosensors play a significant role in sensing technologies among many wearable biosensors. Sensing electrodes plays a substantial role in the construction of wearable sensors in this technology. Advanced 2D materials, such as MXene, have replaced traditional contact surface areas and introduced appropriate electrical and mechanical properties.201 Furthermore, the use of MXene in conjunction with biomarkers for microfluidic wearable electrochemical biosensors has received little attention in wearable microfluidic systems, but it is fast gaining traction In this regard, Lei et al. have introduced MXene-based microfluidic wearable electrochemical biosensors for sweat detection. Due to the excellent electrochemical activity and high conductivity, exfoliated MXene (Ti3C2Tx) with PB composites displayed higher electrochemical performance than graphene/PB and CNT/PB composites against H2O2 detection. In this case, the sensor device has a changeable sensor component that can be inserted and replaced with customized sensors designed to track various analytes such as lactate, glucose or pH value. The device substrate was composed of superhydrophobic carbon fiber to produce a tri-phase contact and protect the connector from sweat corrosion. The sensing performance of the manufactured device was evaluated using artificial sweat, and it demonstrated glucose sensitivity around 35.3 μA mm−1 cm−2 and lactate sensitivity around 11.4 μA mm−1 cm−2.202Fig. 13b depicts the schematic diagram of the wearable multipurpose biosensing patch based on Ti3C2Tx/PB composite. The sensor was also tested for human sweat analysis. The test findings showed simultaneous lactate and glucose readings with great sensitivity and reproducibility. A skin-conforming flexible biosensor with three interchangeable sensor units is integrated onto a single wearable platform in the constructed system. O2 access to the active enzymatic layer is infinite, promising intense enzyme activity and resulting inaccuracy, ultra-high sensitivity and a wider linear detection range.
Similarly, Noh et al. reported a new wearable impedimetric immunosensor comprising a chamber based on Ti3C2Tx-loaded LBG (laser-burned graphene) flakes 3D electrode and microfluidic channel for the non-invasive detection of cortisol in human perspiration. To create these elastic and flexible patch sensors, PDMS was used as a substrate, and LBG was placed onto the PDMS substrate by removing the PI film. When the LBG was transferred to the PDMS, a disconnection formed between the LBG flakes due to transferring and burning, which mainly influenced the electrochemical sensing response of the LBG electrode. As a result, a highly conductive MXene was introduced into the electrode, with good enzyme loading capacities and electrochemical characteristics. The microfluidic system was constructed using PDMS and a 3D-printed mold. Sweat was gathered via a patch sensor that was adhered to the skin with a double-sided adhesive film. Then a hole was created in the adhesive layer via which perspiration secreted by the skin is transmitted to the chamber under natural pressure via the channel. The PDMS/LBG MXene-based patch sensor had linearity and LOD around 0.01–100 Nm and 88 pM, respectively, under ideal conditions.203 Wearable microfluidic biosensors for sweat analytics offer a viable method for detecting biomarkers without invasive testing. Nonetheless, sweat-based sensing faces several challenges, including short sensor shelf lives when using these whole working electrodes patterned using traditional techniques, easy degradation of biomaterials and enzymes with repeated tests, and limited sensitivity and detection range of enzyme-based biosensors due to sweat oxygen deficiency. The performance of microfluidic wearable biosensors based on 2D materials is shown in Table 2.
7.2. Conclusion and future directions
There are several obstacles to overcome to realize microfluidic wearable biosensors. Microstructure design and electronics functionality are two significant areas of concern. Fig. 14 depicts a schematic illustration of the general issues of wearable systems and the practical features of sensing entities. Biosensor wearability is primarily determined by materials that match human skin's biological, chemical, and physical attributes. However, difficulties arise because the human body contains uneven surfaces that are tough to remove. Human skin is also viscoelastic and very deformable. The outer epidermis layer permits fluid exchange to carry on homeostasis within the body. Finally, the adverse reaction of the epidermis towards foreign materials or chemicals, causing discomfort and skin irritation. So, the sensor material interface demands to consider all these requirements.
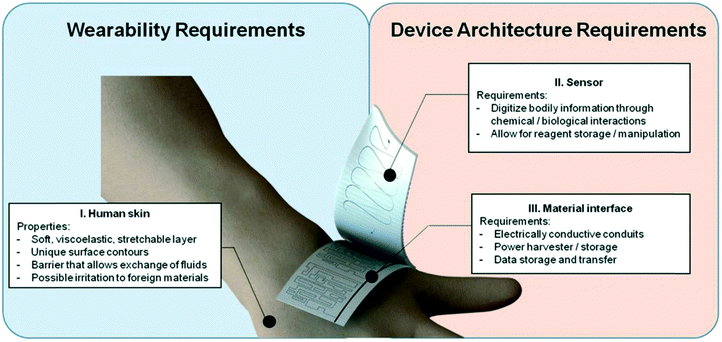 |
| Fig. 14 Schematic representation of the broad challenges of wearable systems and the practical aspects of the sensing entities. | |
To summarise, skin qualities should be compatible with biosensors while achieving digitalization of bodily information via specialized mechanical or chemical interactions, or both. Despite the fact that many of the appealing features of microfluidics/LOC devices have enabled the use of microchip-based platforms in life science and biology, microfluidic technologies often increase the performance of existing macroscale assays or give comparable alternatives.204 On the other hand, they have not reached their full capabilities due to a lack of new essential qualities. On the other hand, microfluidics technologies have lately been adopted to address difficulties that have not yet been addressed by the traditional laboratory tabletop method. Skin interfaced wearable biosensors used in sports analytics and wearable healthcare monitoring are one such example. Although this sector is still in its early stages, the foundations are extraordinarily strong; wearable LOC devices are gradually integrating with traditional techniques like flexible electronics, microfluidics, biocompatible materials, etc. Wearable biosensors are still in their developing stage with much ability for improvement in performance (accuracy, reliability, resolution, durability, limit of detection) and their sensing parameters (temperature, pressure, pH, force, hydration). Microfluidic wearable biosensors open up new avenues for improving life productivity and quality and enabling individual healthcare. Next-generation microfluidic wearables have the potential to provide unprecedented sensing, control, and monitoring capabilities. Considering these facts, we predict some potential and constraints for next-generation wearable systems for clinical applications.205 Despite many possibilities lying ahead, several challenges need to be conquered to implement wearable microfluidic sensors for clinical biosensing applications successfully. Many issues are critical and must be addressed right now. The extended stability and endurance of different parts of wearable microfluidics, for example, have yet to be thoroughly described and presented. At the moment, the majority of the individual components of wearable microfluidic-based devices are still in the early stages of research and are constrained to the lab setting. Additional work and robust characterization are required to get beyond this early level. These individual elements must go through a series of accelerated life tests to determine whether they can resist the wear and tear associated with the continuous use of wearable gadgets. Furthermore, by combining various components of wearable microfluidic technology, completely functionalized wearable devices can be created. Specifically, this means that the entire sequence of external stimuli collection, digitalization, evaluation, and data transmission must be completed without interruption. Complete integration of numerous functions in conformable, stretchable, and flexible tiny parts is required.193 These recent papers have highlighted a variety of prospects for microfluidic wearables along with challenges in the biosensing area. Finally, we anticipate that ongoing and progressive research in various areas, including material science, electronics, chemistry, and physics, will improve the applicability of microfluidic wearable biosensors.
Microfluidics and microfluidic wearables have enormous potential in biomedical applications such as continuous health diagnosis, monitoring, and therapy. In this paper, promising research works in sensing based on 2D materials for diverse health care and environmental applications are highlighted. We anticipate that these devices will eventually improve and prolong the quality of life of humans and, ultimately, society as a whole.
Conflicts of interest
The authors declare no conflict of interest.
Acknowledgements
This work was financially supported by the Department of Science and Technology (DST)-SERB Early Career Research project (Grant No. ECR/2017/001850), DST-Nanomission (DST/NM/NT/2019/205(G)), Karnataka Science and Technology Promotion Society (KSTePS/VGST-RGS-F/2018-19/GRD NO. 829/315). Authors would like to acknowledge the financial support from DST (Nanomission) Govt of India (SR/NM/NS-52/2016(G)).
References
-
D. Antuña-Jiménez, G. Díaz-Díaz, M. C. Blanco-López, M. J. Lobo-Castañón, A. J. Miranda-Ordieres and P. Tuñón-Blanco, in Molecularly Imprinted Sensors, ed. S. Li, Y. Ge, S. A. Piletsky and J. Lunec, Elsevier, Amsterdam, 2012, pp. 1–34 Search PubMed.
-
World Health Organization, Guidelines for drinking-water quality, World Health Organization, Geneva, 4th edn, 2011 Search PubMed.
- K. Yang, H. Peretz-Soroka, Y. Liu and F. Lin, Lab. Chip, 2016, 16, 943–958 RSC.
- R. Antony, M. S. Giri Nandagopal, N. Sreekumar and N. Selvaraju, Microsyst. Technol., 2014, 20, 1051–1061 CrossRef.
- X. Chen, S. Zhang, W. Han, Z. Wu, Y. Chen and S. Wang, J. Chem. Technol. Biotechnol., 2018, 93, 3353–3363 CrossRef CAS.
- S. A. Jaywant and K. M. Arif, Sensors, 2019, 19, 4781 CrossRef CAS.
- S. Gupta, K. Ramesh, S. Ahmed and V. Kakkar, Int. J. Bio-Sci. Bio-Technol., 2016, 8, 311–322 CrossRef.
- H. Andersson and A. van den Berg, Sens. Actuators, B., 2003, 92, 315–325 CrossRef CAS.
- S. Mashaghi, A. Abbaspourrad, D. A. Weitz and A. M. van Oijen, Trends Anal. Chem., 2016, 82, 118–125 CrossRef CAS.
- L. Reverté, B. Prieto-Simón and M. Campàs, Anal. Chim. Acta, 2016, 908, 8–21 CrossRef.
- F. Ghorbani Zamani, H. Moulahoum, M. Ak, D. Odaci Demirkol and S. Timur, TrAC, Trends Anal. Chem., 2019, 118, 264–276 CrossRef CAS.
- S. Wu, X. Wang, Z. Li, S. Zhang and F. Xing, Micromachines, 2020, 11, 1059 CrossRef.
- J. Kudr, O. Zitka, M. Klimanek, R. Vrba and V. Adam, Sens. Actuators, B, 2017, 246, 578–590 CrossRef CAS.
-
R. J. Toh, C. C. Mayorga-Martinez, J. Han, Z. Sofer and M. Pumera, Group 6 Layered Transition-Metal Dichalcogenides in Lab-on-a-Chip Devices, https://pubs.acs.org/doi/pdf/10.1021/acs.analchem.7b00302, accessed 9 September 2021.
- J. Sengupta and C. M. Hussain, TrAC, Trends Anal. Chem., 2019, 114, 326–337 CrossRef CAS.
- Y. Huang, Y. Shi, H. Y. Yang and Y. Ai, Nanoscale, 2015, 7, 2245–2249 RSC.
- J. Liu, X. Jiang, R. Zhang, Y. Zhang, L. Wu, W. Lu, J. Li, Y. Li and H. Zhang, Adv. Funct. Mater., 2019, 29, 1807326 CrossRef.
- Scottish Graphene Firm Launches Flagship Product Under New Face, https://www.printedelectronicsworld.com/articles/22141/scottish-graphene-firm-launches-flagship-product-under-new-face, accessed 11 September 2021.
- V. V. Pokropivny and V. V. Skorokhod, Mater. Sci. Eng. C, 2007, 27, 990–993 CrossRef CAS.
- D. Tyagi, H. Wang, W. Huang, L. Hu, Y. Tang, Z. Guo, Z. Ouyang and H. Zhang, Nanoscale, 2020, 12, 3535–3559 RSC.
- C. N. R. Rao, A. K. Sood, K. S. Subrahmanyam and A. Govindaraj, Angew. Chem., Int. Ed., 2009, 48, 7752–7777 CrossRef CAS.
- C. Anichini, W. Czepa, D. Pakulski, A. Aliprandi, A. Ciesielski and P. Samorì, Chem. Soc. Rev., 2018, 47, 4860–4908 RSC.
- D. J. Late, Y. K. Huang, B. Liu, J. Acharya, S. N. Shirodkar, J. Luo, A. Yan, D. Charles, U. V. Waghmare, V. P. Dravid and C. N. R. Rao, ACS Nano, 2013, 7, 4879–4891 CrossRef CAS.
- X. Liu, T. Ma, N. Pinna and J. Zhang, Adv. Funct. Mater., 2017, 27, 1–30 Search PubMed.
- S. Xu, Z. Yan, K. I. Jang, W. Huang, H. Fu, J. Kim, Z. Wei, M. Flavin, J. McCracken, R. Wang, A. Badea, Y. Liu, D. Xiao, G. Zhou, J. Lee, H. U. Chung, H. Cheng, W. Ren, A. Banks, X. Li, U. Paik, R. G. Nuzzo, Y. Huang, Y. Zhang and J. A. Rogers, Science, 2015, 347, 154–159 CrossRef CAS PubMed.
- K. S. Kumar, N. Choudhary, Y. Jung and J. Thomas, ACS Energy Lett., 2018, 3, 482–495 CrossRef CAS.
- N. Convery and N. Gadegaard, Micro Nano Eng., 2019, 2, 76–91 CrossRef.
- H. A. Stone, A. D. Stroock and A. Ajdari, Annu. Rev. Fluid Mech., 2004, 36, 381–411 CrossRef.
- L. Baharudin, Instrum. Sci. Technol., 2008, 36, 222–230 CrossRef CAS.
- J. Wu and M. Gu, J. Biomed. Opt., 2011, 16, 080901 CrossRef.
- G. A. Schultz, T. N. Corso, S. J. Prosser and S. Zhang, Anal. Chem., 2000, 72, 4058–4063 CrossRef CAS PubMed.
- L. Licklider, X.-Q. Wang, A. Desai, Y.-C. Tai and T. D. Lee, Anal. Chem., 2000, 72, 367–375 CrossRef CAS.
- J. Kameoka, R. Orth, B. Ilic, D. Czaplewski, T. Wachs and H. G. Craighead, Anal. Chem., 2002, 74, 5897–5901 CrossRef CAS PubMed.
- B. M. D. C. Costa, S. Griveau, F. d’Orlye, F. Bedioui, J. A. F. da Silva and A. Varenne, Electrochim. Acta, 2021, 391, 138928 CrossRef CAS.
- W. Cha, Y.-C. Tung, M. E. Meyerhoff and S. Takayama, Anal. Chem., 2010, 82, 3300–3305 CrossRef CAS PubMed.
- M. Bauer, L. Wunderlich, F. Weinzierl, Y. Lei, A. Duerkop, H. N. Alshareef and A. J. Baeumner, Anal. Bioanal. Chem., 2021, 413, 763–777 CrossRef CAS.
- D. Ross, M. Gaitan and L. E. Locascio, Anal. Chem., 2001, 73, 4117–4123 CrossRef CAS.
- A. Q. Liu, H. J. Huang, L. K. Chin, Y. F. Yu and X. C. Li, Anal. Bioanal. Chem., 2008, 391, 2443–2452 CrossRef CAS.
- O. Tamarin, S. Comeau, C. Déjous, D. Moynet, D. Rebière, J. Bezian and J. Pistré, Biosens. Bioelectron., 2003, 18, 755–763 CrossRef CAS.
- B. Godber, K. S. J. Thompson, M. Rehak, Y. Uludag, S. Kelling, A. Sleptsov, M. Frogley, K. Wiehler, C. Whalen and M. A. Cooper, Clin. Chem., 2005, 51, 1962–1972 CrossRef CAS.
- L.-S. Bouchard, S. R. Burt, M. S. Anwar, K. V. Kovtunov, I. V. Koptyug and A. Pines, Science, 2008, 319, 442–445 CrossRef CAS PubMed.
- V.-V. Telkki, C. Hilty, S. Garcia, E. Harel and A. Pines, J. Phys. Chem. B, 2007, 111, 13929–13936 CrossRef CAS.
- M. Mujika, S. Arana, E. Castaño, M. Tijero, R. Vilares, J. M. Ruano-López, A. Cruz, L. Sainz and J. Berganza, Biosens. Bioelectron., 2009, 24, 1253–1258 CrossRef CAS.
- G. Minas, J. S. Martins, J. C. Ribeiro, R. F. Wolffenbuttel and J. H. Correia, Sens. Actuators Phys., 2004, 110, 33–38 CrossRef CAS.
- B. Filanoski, S. K. Rastogi, E. Cameron, N. N. Mishra, W. Maki and G. Maki, Luminescence, 2008, 23, 22–27 CrossRef CAS.
- O. Hofmann, P. Miller, P. Sullivan, T. S. Jones, J. C. deMello, D. D. C. Bradley and A. J. deMello, Sens. Actuators, B, 2005, 106, 878–884 CrossRef CAS.
- A. M. Jorgensen, K. B. Mogensen, J. P. Kutter and O. Geschke, Sens. Actuators, B, 2003, 90, 15–21 CrossRef CAS.
- W. K. Ridgeway, E. Seitaridou, R. Phillips and J. R. Williamson, Nucleic Acids Res., 2009, 37, e142 CrossRef.
- J. G. Santiago, S. T. Wereley, C. D. Meinhart, D. J. Beebe and R. J. Adrian, Exp. Fluids, 1998, 25, 316–319 CrossRef CAS.
- M. G. Olsen, J. M. Bauer and D. J. Beebe, Appl. Phys. Lett., 2000, 76, 3310–3312 CrossRef CAS.
- D. Sinton, R. Gordon and A. G. Brolo, Microfluid. Nanofluidics, 2008, 4, 107–116 CrossRef CAS.
- A. De Leebeeck, L. K. S. Kumar, V. de Lange, D. Sinton, R. Gordon and A. G. Brolo, Anal. Chem., 2007, 79, 4094–4100 CrossRef CAS.
- I. T. Kim and K. D. Kihm, Exp. Fluids, 2006, 41, 905–916 CrossRef CAS.
- D. J. Sirbuly, A. Tao, M. Law, R. Fan and P. Yang, Adv. Mater., 2007, 19, 61–66 CrossRef CAS.
- P. Dumais, C. L. Callender, J. P. Noad and C. J. Ledderhof, Opt. Express, 2008, 16, 18164–18172 CrossRef CAS PubMed.
- R. G. Heideman and P. V. Lambeck, Sens. Actuators, B, 1999, 61, 100–127 CrossRef CAS.
- J. A. Chediak, Z. Luo, J. Seo, N. Cheung, L. P. Lee and T. D. Sands, Sens. Actuators Phys., 2004, 111, 1–7 CrossRef CAS.
- K. R. Strehle, D. Cialla, P. Rösch, T. Henkel, M. Köhler and J. Popp, Anal. Chem., 2007, 79, 1542–1547 CrossRef CAS.
- B. D. Piorek, S. J. Lee, J. G. Santiago, M. Moskovits, S. Banerjee and C. D. Meinhart, Proc. Natl. Acad. Sci. U. S. A., 2007, 104, 18898–18901 CrossRef CAS PubMed.
- J. C. Fanguy and C. S. Henry, Electrophoresis, 2002, 23, 767–773 CrossRef CAS PubMed.
- W. Cha, Y.-C. Tung, M. E. Meyerhoff and S. Takayama, Anal. Chem., 2010, 82, 3300–3305 CrossRef CAS.
- M. Bauer, L. Wunderlich, F. Weinzierl, Y. Lei, A. Duerkop, H. N. Alshareef and A. J. Baeumner, Anal. Bioanal. Chem., 2021, 413, 763–777 CrossRef CAS PubMed.
- M. Galloway, W. Stryjewski, A. Henry, S. M. Ford, S. Llopis, R. L. McCarley and S. A. Soper, Anal. Chem., 2002, 74, 2407–2415 CrossRef CAS.
- M. Žúborová, Z. Demianová, D. Kaniansky, M. Masár and B. Stanislawski, J. Chromatogr. A, 2003, 990, 179–188 CrossRef.
- R. H. Liu, J. Yang, R. Lenigk, J. Bonanno and P. Grodzinski, Anal. Chem., 2004, 76, 1824–1831 CrossRef CAS PubMed.
- Mass Spectrometry, https://www.wiley.com/en-gb/Mass+Spectrometry%3A+Principles+and+Applications%2C+3rd+Edition-p-9780470033104, accessed 27 December 2021.
- D. F. Hunt, J. R. Yates, J. Shabanowitz, S. Winston and C. R. Hauer, Proc. Natl. Acad. Sci. U. S. A., 1986, 83, 6233–6237 CrossRef CAS PubMed.
- G. A. Schultz, T. N. Corso, S. J. Prosser and S. Zhang, Anal. Chem., 2000, 72, 4058–4063 CrossRef CAS PubMed.
- L. Licklider, X.-Q. Wang, A. Desai, Y.-C. Tai and T. D. Lee, Anal. Chem., 2000, 72, 367–375 CrossRef CAS.
- H. Gao, C. Yan, W. Wu and J. Li, Sensors, 2020, 20, 1792 CrossRef CAS PubMed.
- P. Pattanayak, S. K. Singh, M. Gulati, S. Vishwas, B. Kapoor, D. K. Chellappan, K. Anand, G. Gupta, N. K. Jha, P. K. Gupta, P. Prasher, K. Dua, H. Dureja, D. Kumar and V. Kumar, Microfluid. Nanofluidics, 2021, 25, 99 CrossRef.
- Y. Zhang, J. Qi, F. Liu, N. Wang, X. Sun, R. Cui, J. Yu, J. Ye, P. Liu, B. Li and L. Chen, Se Pu Chin. J. Chromatogr., 2021, 39, 802–815 Search PubMed.
- J. Mairhofer, K. Roppert and P. Ertl, Sensors, 2009, 9, 4804–4823 CrossRef CAS PubMed.
- C. N. R. Rao, K. Gopalakrishnan and U. Maitra, ACS Appl. Mater. Interfaces, 2015, 7, 7809–7832 CrossRef CAS PubMed.
- J. Sengupta and C. M. Hussain, TrAC, Trends Anal. Chem., 2019, 114, 326–337 CrossRef CAS.
- E. W. Hill, A. Vijayaragahvan and K. Novoselov, IEEE Sens. J., 2011, 11, 3161–3170 CAS.
- O. Moldovan, B. Iñiguez, M. J. Deen and L. F. Marsal, IET Circuits Dev. Syst., 2015, 9, 446–453 CrossRef.
-
E. M. Materón, R. S. Lima, N. Joshi, F. M. Shimizu and O. N. Oliveira, in Graphene-Based Electrochemical Sensors for Biomolecules, ed. A. Pandikumar and P. Rameshkumar, Elsevier, 2019, pp. 321–336 Search PubMed.
- X. Chen, S. Zhang, W. Han, Z. Wu, Y. Chen and S. Wang, J. Chem. Technol. Biotechnol., 2018, 93, 3353–3363 CrossRef CAS.
- S. Wu, X. Wang, Z. Li, S. Zhang and F. Xing, Micromachines, 2020, 11, 1–25 Search PubMed.
- Green Synthesis of Graphene Based Biomaterial Using Fenugreek Seeds for Lipid Detection|ACS Sustainable Chemistry & Engineering DOI:10.1021/acssuschemeng.5b00923, accessed 17 May 2021.
- A. C. Q. Silva, C. Vilela, H. A. Santos, A. J. D. Silvestre and C. S. R. Freire, Appl. Mater. Today, 2020, 18, 100450 CrossRef.
- J. L. Garcia-Cordero and S. J. Maerkl, Curr. Opin. Biotechnol., 2020, 65, 37–44 CrossRef CAS PubMed.
- C. Chircov, A. C. Bîrcă, A. M. Grumezescu and E. Andronescu, Molecules, 2020, 25, 6013 CrossRef CAS PubMed.
- Y.-E. Choi, J.-W. Kwak and J. W. Park, Sensors, 2010, 10, 428–455 CrossRef CAS PubMed.
- Visual and high-throughput detection of cancer cells using a graphene oxide-based FRET aptasensing microfluidic chip - Lab on a Chip (RSC Publishing), https://pubs.rsc.org/en/content/articlelanding/2012/lc/c2lc40564d#!divAbstract, accessed 22 May 2021.
- Paper-Based Microfluidic Electrochemical Immunodevice Integrated with Nanobioprobes onto Graphene Film for Ultrasensitive Multiplexed Detection of Cancer Biomarkers|Analytical Chemistry DOI:10.1021/ac401445a, accessed 24 May 2021.
- Y. Wu, C. Wang, P. Wang, C. Wang, Y. Zhang and L. Han, RSC Adv., 2021, 11, 8124–8133 RSC.
-
Z. Niu, M. A. Kozminsky, K. C. Day, P. L. Palmbos, M. L. Day and S. Nagrath, Poster Presentations - Proffered Abstracts, American Association for Cancer Research, 2020, p. B35 Search PubMed.
- Y. An, T. Jin, Y. Zhu, F. Zhang and P. He, Biosens. Bioelectron., 2019, 142, 111503 CrossRef CAS PubMed.
- Y. Wang, S. Sun, J. Luo, Y. Xiong, T. Ming, J. Liu, Y. Ma, S. Yan, Y. Yang, Z. Yang, J. Reboud, H. Yin, J. M. Cooper and X. Cai, Microsyst. Nanoeng., 2020, 6, 1–9 CrossRef PubMed.
- K. S. Prasad, X. Cao, N. Gao, Q. Jin, S. T. Sanjay, G. Henao-Pabon and X. Li, Sens. Actuators, B, 2020, 305, 127516 CrossRef CAS PubMed.
- H. Torul, E. Yarali, E. Eksin, A. Ganguly, J. Benson, U. Tamer, P. Papakonstantinou and A. Erdem, Biosensors, 2021, 11, 236 CrossRef CAS PubMed.
- A. N. Bhatt, R. Mathur, A. Farooque, A. Verma and B. S. Dwarakanath, Ind. J. Med. Res., 2010, 132, 129–149 CAS.
- S. Hammarström, Semin. Cancer Biol., 1999, 9, 67–81 CrossRef PubMed.
- Y.-K. Yen, C.-H. Chao and Y.-S. Yeh, Sensors, 2020, 20, 1372 CrossRef CAS PubMed.
- B. Guo, L. Fang, B. Zhang and J. R. Gong, Insci. J., 2011, 80–89 CrossRef CAS.
- V. V. Chaban and O. V. Prezhdo, Nanoscale, 2016, 8, 15521–15528 RSC.
- S. Agnoli and M. Favaro, J. Mater. Chem. A, 2016, 4, 5002–5025 RSC.
- H. Li, J. Qin, M. Li, C. Li, S. Xu, L. Qian and B. Yang, Sens. Actuators, B, 2020, 302, 127209 CrossRef CAS.
- C.-W. Lin and C.-C. Chang, Biosens. Cancer, 2011, 17 Search PubMed.
- Y. Li, A. W. Wark, H. J. Lee and R. M. Corn, Anal. Chem., 2006, 78, 3158–3164 CrossRef CAS PubMed.
- D. T. Nurrohman and N.-F. Chiu, Nanomaterials, 2021, 11, 216 CrossRef CAS PubMed.
- M. B. Hossain, M. M. Islam, L. F. Abdulrazak, M. M. Rana, T. B. A. Akib and M. Hassan, Photonic Sens., 2020, 10, 67–79 CrossRef CAS.
- D. Moschou and A. Tserepi, Lab. Chip, 2017, 17, 1388–1405 RSC.
- Y.-J. Chang and H. You, Chin. J. Anal. Chem., 2019, 47, 965–975 CAS.
- N. C. S. Vieira, J. Borme, G. Machado Jr., F. Cerqueira, P. P. Freitas, V. Zucolotto, N. M. R. Peres and P. Alpuim, J. Phys.: Condens. Matter, 2016, 28, 085302 CrossRef CAS PubMed.
- F. Chen, Q. Qing, J. Xia and N. Tao, Chem. – Asian J., 2010, 5, 2144–2153 CrossRef CAS PubMed.
- Measurement of the quantum capacitance of graphene|Nature Nanotechnology, https://www.nature.com/articles/nnano.2009.177, accessed 20 July 2021.
- S. Papamatthaiou, P. Estrela and D. Moschou, Sci. Rep., 2021, 11, 9815 CrossRef CAS PubMed.
- G. Saltzgaber, P. Wojcik, T. Sharf, M. R. Leyden, J. L. Wardini, C. A. Heist, A. A. Adenuga, V. T. Remcho and E. D. Minot, Nanotechnology, 2013, 24, 355502 CrossRef PubMed.
- Y. Wang, Y. Xiao, G. Gao, J. Chen, R. Hou, Q. Wang, L. Liu and J. Fu, J. Mater. Chem. B, 2017, 5, 511–516 RSC.
- N. Singh, M. A. Ali, P. Rai, I. Ghori, A. Sharma, B. D. Malhotra and R. John, Lab. Chip, 2020, 20, 760–777 RSC.
- M. Asif, A. Aziz, M. Azeem, Z. Wang, G. Ashraf, F. Xiao, X. Chen and H. Liu, Adv. Colloid Interface Sci., 2018, 262, 21–38 CrossRef CAS PubMed.
- S. Dong, A. Q. Dao, B. Zheng, Z. Tan, C. Fu, H. Liu and F. Xiao, Electrochim. Acta, 2015, C, 195–201 CrossRef.
- Z. Wang, M. Gui, M. Asif, Y. Yu, S. Dong, H. Wang, W. Wang, F. Wang, F. Xiao and H. Liu, Nanoscale, 2018, 10, 6629–6638 RSC.
- Sensors|Free Full-Text|Electrochemical Detection of Glucose Molecules Using Laser-Induced Graphene Sensors: A Review, https://www.mdpi.com/1424-8220/21/8/2818, accessed 24 July 2021.
- P. Rewatkar, A. Kothuru and S. Goel, IEEE Trans. Electron. Dev., 2020, 67, 1832–1838 CAS.
- M. Bhaiyya, P. Rewatkar, M. Salve, P. K. Pattnaik and S. Goel, IEEE Trans. NanoBiosci., 2021, 20, 79–85 Search PubMed.
- M. Bhaiyya, P. K. Pattnaik and S. Goel, Sens. Actuators, Phys., 2021, 331, 112831 CrossRef CAS.
- M. L. Bhaiyya, P. K. Pattnaik and S. Goel, IEEE Trans. Instrum. Meas., 2021, 70, 1–8 Search PubMed.
- M. Bhaiyya, P. K. Pattnaik and S. Goel, IEEE Trans. Electron. Dev., 2021, 68, 2447–2454 CAS.
- Roekmono, H. Hadi, H. N. Imtihani and R. A. Wahyuono, Int. J. Drug Delivery Technology, 2020, 10, 295–300 CrossRef.
- L. Cao, G.-C. Han, H. Xiao, Z. Chen and C. Fang, Anal. Chim. Acta, 2020, 1096, 34–43 CrossRef CAS.
- Graphene Field Effect Transistors for Biomedical Applications: Current Status and Future Prospects - PubMed, https://pubmed.ncbi.nlm.nih.gov/28933752/, accessed 1 August 2021.
-
Y. Zhi, J. Jian, Y. Qiao, Y. Tian, Y. Yang and T.-L. Ren, 2020 21st International Conference on Electronic Packaging Technology (ICEPT), 2020, pp. 1–6.
- A. Wiorek, M. Parrilla, M. Cuartero and G. A. Crespo, Anal. Chem., 2020, 92, 10153–10161 CrossRef CAS PubMed.
- S. J. Fishlock, G. Bhattacharya and J. McLaughlin, ECS Meet. Abstr., 2020, MA2020-01, 2347 CrossRef.
- A. Schuck, H. E. Kim, J. K. Moreira, P. S. Lora and Y.-S. Kim, Sensors, 2021, 21, 1852 CrossRef CAS PubMed.
- J. S. C. Chew, M. Saleem, C. M. Florkowski and P. M. George, Clin. Biochem. Rev., 2008, 29, 47–62 Search PubMed.
- G. Kaur and E. Levy, Front. Mol. Neurosci., 2012, 5, 79 CrossRef CAS PubMed.
- M. H. Gozashti, A. Gholamhosseinian, F. Musavi and M. Mashrouteh, Iran. J. Reprod. Med., 2013, 11, 71–76 CAS.
- K. S. S. Devi and U. M. Krishnan, Microchim. Acta, 2020, 187, 585 CrossRef CAS PubMed.
- [PDF] A laser-engraved wearable sensor for sensitive detection of uric acid and tyrosine in sweat|Semantic Scholar, https://www.semanticscholar.org/paper/A-laser-engraved-wearable-sensor-for-sensitive-of-Yang-Song/fbdbc1416c9f3cbcadbfad7131c5a1c5acc69a60#citing-papers, accessed 11 August 2021.
- G. M. Stojanović, T. Kojić, M. Simić, A. Jovanović-Galović, B. Pavlović, A. Zurutuza, L. Anzi and R. Sordan, IEEE Sens. J., 2021, 21, 16744–16753 Search PubMed.
- A. E. Moutaouakil, S. Poovathy, M. Belmoubarik and W. K. Peng, Phys., 2020 Search PubMed , arXiv:2006.11881.
- J. Sengupta, A. Adhikari and C. M. Hussain, Carbon Trends, 2021, 4, 100072 CrossRef.
- M. A. Ali, C. Hu, S. Jahan, B. Yuan, M. S. Saleh, E. Ju, S.-J. Gao and R. Panat, Adv. Mater. Deerfield Beach Fla, 2021, 33, e2006647 CrossRef PubMed.
- S. Damiati, S. Søpstad, M. Peacock, A. S. Akhtar, I. Pinto, R. R. G. Soares and A. Russom, IEEE Sens. J., 2021, 21, 13060–13067 CAS.
- M. A. Ehsan, S. A. Khan and A. Rehman, Diagnostics, 2021, 11, 1030 CrossRef CAS PubMed.
- P. Teengam, W. Siangproh, S. Tontisirin, A. Jiraseree-amornkun, N. Chuaypen, P. Tangkijvanich, C. S. Henry, N. Ngamrojanavanich and O. Chailapakul, Sens. Actuators, B, 2021, 326, 128825 CrossRef CAS.
- S. Boonkaew, A. Yakoh, N. Chuaypen, P. Tangkijvanich, S. Rengpipat, W. Siangproh and O. Chailapakul, Biosens. Bioelectron., 2021, 193, 113543 CrossRef CAS PubMed.
- M. Pumera, A. Ambrosi, A. Bonanni, E. L. K. Chng and H. L. Poh, TrAC, Trends Anal. Chem., 2010, 29, 954–965 CrossRef CAS.
- Chemically Modified Graphenes as Detectors in Lab-on-Chip Device - Chua - 2013 - Electroanalysis - Wiley Online Library, https://analyticalsciencejournals.onlinelibrary.wiley.com/doi/10.1002/elan.201200583, accessed 22 August 2021.
- C. K. Chua and M. Pumera, Electrophoresis, 2013, 34, 2007–2010 CrossRef CAS PubMed.
- K. Pungjunun, S. Nantaphol, N. Praphairaksit, W. Siangproh, S. Chaiyo and O. Chailapakul, Sens. Actuators, B, 2020, 318, 128241 CrossRef CAS.
- J. Wang, X. Zhang, Z. Wei, H. Qiu, Y. Chen, Y. Geng, Y. Du, Z. Cheng and X. Li, IEEE Access, 2021, 9, 56479–56485 Search PubMed.
- R. A. Soomro, S. Jawaid, Q. Zhu, Z. Abbas and B. Xu, Chin. Chem. Lett., 2020, 31, 922–930 CrossRef CAS.
- Y. Lei, W. Zhao, Y. Zhang, Q. Jiang, J.-H. He, A. J. Baeumner, O. S. Wolfbeis, Z. L. Wang, K. N. Salama and H. N. Alshareef, Small, 2019, 15, 1901190 CrossRef PubMed.
- M. Li, L. Wang, R. Liu, J. Li, Q. Zhang, G. Shi, Y. Li, C. Hou and H. Wang, Biosens. Bioelectron., 2021, 174, 112828 CrossRef CAS PubMed.
- S. Zhang, M. A. Zahed, M. Sharifuzzaman, S. Yoon, X. Hui, S. Chandra Barman, S. Sharma, H. S. Yoon, C. Park and J. Y. Park, Biosens. Bioelectron., 2021, 175, 112844 CrossRef CAS PubMed.
- M. M. Tunesi, R. A. Soomro, X. Han, Q. Zhu, Y. Wei and B. Xu, Nano Converg., 2021, 8, 5 CrossRef CAS PubMed.
- K. Yang, K. Zhu, Y. Wang, Z. Qian, Y. Zhang, Z. Yang, Z. Wang, L. Wu, S. Zong and Y. Cui, ACS Nano, 2021, 15, 12996–13006 CrossRef CAS PubMed.
- P. Yager, T. Edwards, E. Fu, K. Helton, K. Nelson, M. R. Tam and B. H. Weigl, Nature, 2006, 442, 412–418 CrossRef CAS PubMed.
- R. J. Toh, C. C. Mayorga-Martinez, J. Han, Z. Sofer and M. Pumera, Anal. Chem., 2017, 89, 4978–4985 CrossRef CAS PubMed.
- S. He, B. Song, D. Li, C. Zhu, W. Qi, Y. Wen, L. Wang, S. Song, H. Fang and C. Fan, Adv. Funct. Mater., 2010, 20, 453–459 CrossRef CAS.
- S. Song, Z. Liang, J. Zhang, L. Wang, G. Li and C. Fan, Angew. Chem., Int. Ed., 2009, 48, 8670–8674 CrossRef CAS PubMed.
- R. Yang, J. Jin, Y. Chen, N. Shao, H. Kang, Z. Xiao, Z. Tang, Y. Wu, Z. Zhu and W. Tan, J. Am. Chem. Soc., 2008, 130, 8351–8358 CrossRef CAS PubMed.
- C. Zhu, Z. Zeng, H. Li, F. Li, C. Fan and H. Zhang, J. Am. Chem. Soc., 2013, 135, 5998–6001 CrossRef CAS PubMed.
- P. T. K. Loan, W. Zhang, C.-T. Lin, K.-H. Wei, L.-J. Li and C.-H. Chen, Adv. Mater., 2014, 26, 4838–4844 CrossRef CAS PubMed.
- D. Ren, H. Jiang, Y. Hu, L. Zhang and C. Li, RSC Adv., 2014, 4, 40368–40372 RSC.
- D. Yang, M. Tayebi, Y. Huang, H. Y. Yang and Y. Ai, Sensors, 2016, 16, 1911 CrossRef PubMed.
- S.-K. Eng, P. Pusparajah, N.-S. Ab Mutalib, H.-L. Ser, K.-G. Chan and L.-H. Lee, Front. Life Sci., 2015, 8, 284–293 CrossRef CAS.
- F. Adzitey, N. Huda and G. R. R. Ali, 3 Biotechnol., 2013, 3, 97–107 Search PubMed.
- X. Ni, M. Castanares, A. Mukherjee and S. E. Lupold, Curr. Med. Chem., 2011, 18, 4206–4214 CrossRef CAS PubMed.
- D. Chimene, D. L. Alge and A. K. Gaharwar, Adv. Mater., 2015, 27, 7261–7284 CrossRef CAS PubMed.
- H.-L. Shuai, X. Wu and K.-J. Huang, J. Mater. Chem. B, 2017, 5, 5362–5372 RSC.
- D. Sarkar, W. Liu, X. Xie, A. C. Anselmo, S. Mitragotri and K. Banerjee, ACS Nano, 2014, 8, 3992–4003 CrossRef CAS PubMed.
- S. Z. Butler, S. M. Hollen, L. Cao, Y. Cui, J. A. Gupta, H. R. Gutiérrez, T. F. Heinz, S. S. Hong, J. Huang, A. F. Ismach, E. Johnston-Halperin, M. Kuno, V. V. Plashnitsa, R. D. Robinson, R. S. Ruoff, S. Salahuddin, J. Shan, L. Shi, M. G. Spencer, M. Terrones, W. Windl and J. E. Goldberger, ACS Nano, 2013, 7, 2898–2926 CrossRef CAS PubMed.
- Y.-C. Lin, D. O. Dumcenco, Y.-S. Huang and K. Suenaga, Nat. Nanotechnol., 2014, 9, 391–396 CrossRef CAS PubMed.
- C. N. R. Rao, H. S. S. R. Matte and U. Maitra, Angew. Chem., Int. Ed., 2013, 52, 13162–13185 CrossRef CAS PubMed.
- A. H. Loo, A. Bonanni, A. Ambrosi and M. Pumera, Nanoscale, 2014, 6, 11971–11975 RSC.
- K.-J. Huang, Y.-J. Liu, H.-B. Wang, Y.-Y. Wang and Y.-M. Liu, Biosens. Bioelectron., 2014, 55, 195–202 CrossRef CAS PubMed.
-
C. Singh, M. A. Ali, V. Kumar, R. Ahmad and G. Sumana, 2017, 259 DOI:10.1016/J.SNB.2017.12.094.
- S. Nagabooshanam, S. Roy, A. Mathur, I. Mukherjee, S. Krishnamurthy and L. M. Bharadwaj, Sci. Rep., 2019, 9, 19862 CrossRef CAS PubMed.
- M. Mohammad, A. Razmjou, K. Liang, M. Asadnia and V. Chen, ACS Appl. Mater. Interfaces, 2019, 11, 1807–1820 CrossRef CAS PubMed.
- A. W. Martinez, S. T. Phillips, E. Carrilho, S. W. Thomas, H. Sindi and G. M. Whitesides, Anal. Chem., 2008, 80, 3699–3707 CrossRef CAS PubMed.
- D. A. Bruzewicz, M. Reches and G. M. Whitesides, Anal. Chem., 2008, 80, 3387–3392 CrossRef CAS PubMed.
- A. W. Martinez, S. T. Phillips, B. J. Wiley, M. Gupta and G. M. Whitesides, Lab. Chip, 2008, 8, 2146–2150 RSC.
- G. C. Ilacas, A. Basa, K. J. Nelms, J. D. Sosa, Y. Liu and F. A. Gomez, Anal. Chim. Acta, 2019, 1055, 74–80 CrossRef CAS PubMed.
- W. Qi, L. Zheng, S. Wang, F. Huang, Y. Liu, H. Jiang and J. Lin, Biosens. Bioelectron., 2021, 178, 113020 CrossRef CAS PubMed.
- L. Chen, D. W. McBranch, H. L. Wang, R. Helgeson, F. Wudl and D. G. Whitten, Proc. Natl. Acad. Sci. U. S. A., 1999, 96, 12287–12292 CrossRef CAS PubMed.
- S. Chatterjee, M. S. Fujimoto, Y. H. Cheng, R. Kargupta, J. A. Soltis, R. K. Motkuri and S. Basuray, Sens. Actuators, B, 2019, 284, 663–674 CrossRef CAS.
- Y. H. Cheng, D. Barpaga, J. A. Soltis, V. Shutthanandan, R. Kargupta, K. S. Han, B. P. McGrail, R. K. Motkuri, S. Basuray and S. Chatterjee, ACS Appl. Mater. Interfaces, 2020, 12, 10503–10514 CrossRef CAS PubMed.
- A. Jayakumar, A. Surendranath and M. Pv, Int. J. Pharm., 2018, 551, 309–321 CrossRef CAS PubMed.
- S. Ramalingam, R. Chand, C. B. Singh and A. Singh, Biosens. Bioelectron., 2019, 135, 14–21 CrossRef CAS PubMed.
- R. G. Amorim and R. H. Scheicher, Nanotechnology, 2015, 26, 154002 CrossRef PubMed.
- E. Hu, J. Ning, B. He, Z. Li, C. Zheng, Y. Zhong, Z. Zhang and Y. Hu, J. Mater. Chem. A, 2017, 5, 2271–2279 RSC.
- Simple and Large Scale Construction of MoS2-g-C3N4 Heterostructures Using Mechanochemistry for High Performance Electrochemical Supercapacitor and Visible Light Photocatalytic Applications|Scientific Reports, https://www.nature.com/articles/srep43055#Abs1, accessed 31 December 2021.
- A. Rana, M. Killa, N. Yadav, A. Mishra, A. Mathur, A. Kumar, M. Khanuja, J. Narang and R. Pilloton, Sensors, 2020, 20, 2070 CrossRef CAS PubMed.
- W. Gao, S. Emaminejad, H. Y. Y. Nyein, S. Challa, K. Chen, A. Peck, H. M. Fahad, H. Ota, H. Shiraki, D. Kiriya, D.-H. Lien, G. A. Brooks, R. W. Davis and A. Javey, Nature, 2016, 529, 509–514 CrossRef CAS PubMed.
- J. Guo, Y. Yu, L. Cai, Y. Wang, K. Shi, L. Shang, J. Pan and Y. Zhao, Mater. Today, 2021, 44, 105–135 CrossRef CAS.
- Y. Yu, X. Wang, C. Yang and L. Shang, Lab. Chip, 2016, 16, 4082–4090 RSC.
- A. Bolotsky, D. Butler, C. Dong, K. Gerace, N. R. Glavin, C. Muratore, J. A. Robinson and A. Ebrahimi, ACS Nano, 2019, 13, 9781–9810 CrossRef CAS PubMed.
- S. Guo, K. Wu, C. Li, H. Wang, Z. Sun, D. Xi, S. Zhang, W. Ding, M. E. Zaghloul, C. Wang, F. A. Castro, D. Yang and Y. Zhao, Matter, 2021, 4, 969–985 CrossRef CAS PubMed.
- N. Jiang, Y. Montelongo, H. Butt and A. K. Yetisen, Small, 2018, 14, 1704363 CrossRef PubMed.
- T. Kamei and T. Wada, Appl. Phys. Lett., 2006, 89, 114101 CrossRef.
- S. Luo, S. Wu, J. Xu, X. Zhang, L. Zou, R. Yao, L. Jin and Y. Li, Appl. Nanosci., 2020, 10, 3703–3716 CrossRef CAS.
- O. Lopez-Sanchez, D. Lembke, M. Kayci, A. Radenovic and A. Kis, Nat. Nanotechnol., 2013, 8, 497–501 CrossRef CAS PubMed.
- A. Shokri and N. Salami, Sens. Actuators, B, 2016, 236, 378–385 CrossRef CAS.
- J. S. Nah, S. C. Barman, M. A. Zahed, M. Sharifuzzaman, H. Yoon, C. Park, S. Yoon, S. Zhang and J. Y. Park, Sens. Actuators, B, 2021, 329, 129206 CrossRef CAS.
- Y. Lei, W. Zhao, Y. Zhang, Q. Jiang, J.-H. He, A. J. Baeumner, O. S. Wolfbeis, Z. L. Wang, K. N. Salama and H. N. Alshareef, Small, 2019, 15, 1901190 CrossRef PubMed.
- J. S. Nah, S. C. Barman, M. A. Zahed, M. Sharifuzzaman, H. Yoon, C. Park, S. Yoon, S. Zhang and J. Y. Park, Sens. Actuators, B, 2021, 329, 129206 CrossRef CAS.
- J. C. Yeo, Kenry and C. T. Lim, Lab. Chip, 2016, 16, 4082–4090 RSC.
- A. Sharma, M. Badea, S. Tiwari and J. L. Marty, Molecules, 2021, 26, 748 CrossRef CAS PubMed.
- T. W. Odom, J. C. Love, D. B. Wolfe, K. E. Paul and G. M. Whitesides, Langmuir, 2002, 18, 5314–5320 CrossRef CAS.
- H. Cao, J. O. Tegenfeldt, R. H. Austin and S. Y. Chou, Appl. Phys. Lett., 2002, 81, 3058–3060 CrossRef CAS.
- U. M. Attia, S. Marson and J. R. Alcock, Microfluid. Nanofluidics, 2009, 7, 1 CrossRef CAS.
- G.-L. Roth, C. Esen and R. Hellmann, Opt. Express, 2017, 25, 18442–18450 CrossRef CAS PubMed.
- J. S. Rossier, M. A. Roberts, R. Ferrigno and H. H. Girault, Anal. Chem., 1999, 71, 4294–4299 CrossRef CAS PubMed.
- Y. H. Cheng, D. Barpaga, J. A. Soltis, V. Shutthanandan, R. Kargupta, K. S. Han, B. P. McGrail, R. K. Motkuri, S. Basuray and S. Chatterjee, ACS Appl. Mater. Interfaces, 2020, 12, 10503–10514 CrossRef CAS PubMed.
Footnote |
† S. R. and M. M. contributed equally. |
|
This journal is © The Royal Society of Chemistry 2022 |