DOI:
10.1039/D1MA00541C
(Perspective)
Mater. Adv., 2022,
3, 2960-2970
Prospectus of advanced nanomaterials for antiviral properties
Received
21st June 2021
, Accepted 25th December 2021
First published on 24th January 2022
Abstract
Viral hazards have suddenly increased in the form of the century's biggest pandemic through COVID-19. However, viruses are also associated with other human diseases, the severity of which range from the mild common cold to deleterious cancers and HIV. Conventional anti-viral therapies that have been developed to mitigate deleterious viral effects have not stood the test of time owing to their numerous limitations. This has burdened the research community worldwide with the challenging task of discovering advanced anti-viral strategies to overcome the limitations being faced. In this regard, fortunately, metal and inorganic nanoparticles offer respite as they exhibit tremendous anti-viral potential and are considered a powerful weapon against viral intrusions. Metal nanoparticles of various metals such as silver, gold, and copper have not only successfully attenuated the infectivity of malignant viruses (HIV, HSV, HINI, etc.) in in vitro conditions and in vivo conditions (mainly silver and zinc oxide nanoparticles) but have also successfully overcome the limitations faced by conventional anti-viral therapies. Acting in a resistance insensitive, age and co-morbidity independent and low cytotoxic manner, metal nanoparticles can successfully inhibit viral entry and other viral development processes. In the light of the mechanisms and advantages offered by metal nanoparticles, it is suggested to consider their usage in actual clinical practice rather than as an alternate therapy. Further, considering the mechanisms exhibited by metal nanoparticles to deprive the viral load, we anticipate that the current pandemic (COVID-19) can be treated to some extent via the aid of metal nanoparticles. The successful implication of the hypothesized mechanisms can offer abating strategies to combat the current pandemic and open new avenues to cope with future pandemics. In this prospective, we provide the frontiers and current scenario of various classes of nanoparticles being explored for antiviral activities.
1. Introduction
Viruses are intracellular pathogens capable of capturing host cells to increase their number, thus converting them into factories for their mass production and re-infection.1 The phases of viral infection include attachment to the host cell,2,3 synthesis, assembly of mature virus particles,4 and finally their release.5,6 All these phases succeed through the orchestrated aid of viral proteins as well as the host-cell machinery.1,4,7 To evade viral destruction and confer protection to living beings, researchers have been devising anti-viral strategies for a long time. The conventional anti-viral strategies developed thus far include anti-viral drugs (therapeutics), vaccination and the recent si-RNA-based anti-viral approach. However, due to disadvantages such as resistance, sensitivity,8–10 narrow specificity,11 low therapeutic index of anti-viral drugs,12,13 vaccination failure due to host insensitivity,14 genetic predisposition,15 as well as age and co-morbidities,16,17 the development of more promising anti-viral strategies is necessary. With regards to this, the use of metal and inorganic nanoparticles for advanced anti-viral approaches is promising. The use of nanoparticles in the field of virology is not a novel idea. Nanomaterials have already proved themselves to be efficient nano-sensors, tracking viruses with great efficacy and sensitivity on account of their phenomenal conductivity and photo-electrochemical properties, making them an ideal choice for diagnostic purposes.18–20 Various novel approaches have been developed to investigate advanced nanomaterials for antiviral applications. Besides, nanoparticles, both organic and inorganic, owing to their characteristics properties such as quantum size effect, high surface-to-volume ratio,21 tunable surfaces and easy functionalization with desired chemical moieties have already proven their value as excellent conventional anti-viral therapy carriers.22 In addition, the biomimetic properties of metal and inorganic nanoparticles have enabled researchers to explore their intrinsic anti-viral activity.23 Besides anti-viral activity, metal nanoparticles also possess intrinsic anti-fungal, anti-bacterial and other anti-microbial properties.24,25 It is noteworthy to mention that with the growing research in the field of metal nano-biotechnology, scientists have developed various methods for their synthesis. Currently, the mode for the organic and bioinspired synthesis of metal nanoparticles is evolving, the application of which will solve problems such as their low biocompatibility and cost.26 Metal nanoparticles of either native metals (silver and gold) or metal oxides (copper, zinc, titanium and iron oxides) have successfully attenuated the infectivity of detrimental viruses such as HIV,27,28 HSV,29,30 influenza,31–33 adenovirus34 and hepatitis.35,36 To curb viral progression in hosts, metal nanoparticles execute various mechanisms. These mechanisms involve impediment of viral attachment to the host cells,27,28,34 annihilating viral genome replication or protein synthesis,27,29,35 evoking the immune response of the host to achieve enhanced viral clearance,37 and release of free ions (Cu2+ and Ti2+) in solution, leading to reactive oxygen species (ROS)-mediated viral protein aggregation and death (Table 1).32,38
Table 1 Table depicting the mechanism of the anti-viral activity of nanoparticles against viruses
Serial number |
Nanoparticle |
Target virus |
Mode of action/reference |
a. |
Silver (Ag-NPs) |
HIV |
Bind gp-120 required for binding to CD4 on host cell.27 |
|
HSV (1, 2 and 3) |
Block replication by binding to viral DNA or RNA molecules.28 |
|
Hepatitis B |
Block fusion between virus and host cell via sulfhydryl groups of glycoproteins.29 |
|
Influenza (HINI and H3N2) |
Curbs replication process.39 |
|
COVID-19 |
Impede the transcription of HCV RNA by binding to HCV ds DNA.35 |
|
|
Perturbation to cell fusion process by interacting with hemagglutinin.31 |
|
|
Block the interaction of RBD and ACE-2.40 |
|
|
Suppress synthesis of genomic RNA.41 |
|
|
Stimulate IFN in cells.42 |
|
b. |
Gold (Au-NPs) |
HIV |
Target gp-120 to curtail entry into host cell. |
|
Adenovirus |
Prevent viral adsorption on host cells.30 |
|
HSV |
Targets disulphide bonds of hemagglutinin, which is vital for entry.34 |
|
H1N1 |
Conjugated with MES to increase valency, resulting in polyvalent interaction.43 |
|
|
Block viral entry.44 |
|
c. |
Copper oxide (Cu2O, CuO, and CuI-NPs) |
HINI |
Release Cu2+ ions, leading to ROS production, which ultimately leads to loss of genomic integrity and deactivation of viral enzymes.32 |
|
Hepatitis C |
|
|
HSV |
Restricting viral entry possibly by deforming glycoproteins.36 |
|
COVID-19 |
Inhibiting viral replication by limiting one of the required transcription factors.29 |
|
|
ROS-mediated cell death.45 |
|
d. |
Titanium dioxide (TiO2 NPs) |
H3N2 |
Binds to viral envelope, causing protein aggregation.38 |
|
Norovirus |
Amplifies inflammatory response, leading to enhanced rate of viral clearance.37 |
|
e. |
Zinc oxide (ZnO NPs) |
HSV-1 |
Inhibition of replication processes.46 |
|
HINI |
Inhibition of replication process.33 |
|
f. |
Iron oxide (Fe2O3 and Fe3O4 NPs) |
HINI |
Inhibition of viral entry by interacting with sulphur-bearing protein groups.47 |
The exertion of the anti-viral activities of metal nanoparticles to deplete the viral load successfully overcomes the drawbacks faced by conventional anti-viral therapies.27,28,32 Thus, considering the mechanism executed by metal nanoparticles, the use of metal nanoparticles in actual clinical practice is encouraged. It is noteworthy to mention that the anti-viral properties of metal nanoparticles can also be beneficial for treating COVID-19.40,45,48,49 The successful implication of the hypothesized mechanisms (for COVID-19) can also open avenues to combat future pandemics. In this work, we summarize the recent efforts on advanced nanomaterials, focusing on their effective antiviral activities, possible mechanism of interaction and future prospective considering the inevitable pandemic due to the global spread of COVID-19.
2. Virus: structure & function
Structurally viruses are comprised of a nucleocapsid (proteins and genome), with the addition of an envelope in enveloped viruses. Proteins are either structural, i.e., capsids and glycoproteins, or non-structural, i.e., polymerases, matrix proteins and proteases, in function.1 The viral genome is responsible for encoding proteins to subvert the host cell machinery, which is quite diversified in structure and composition. Its diverse structure ranges from ds DNA, ss DNA, ds RNA, ss positive (+) sense RNA, ss negative (−) sense RNA, and RNA genome with DNA intermediate to multipartite ds DNA form (Baltimore classification). Capsids, which strikingly differ with respect to their symmetry and biochemical properties among viruses (IUCN classification), besides protecting the viral genome, aid in the attachment of viruses to host cell receptors, receptor-mediated endocytosis and un-coating during the pre-infection phase.1,2 The accompanying viral envelope has viral glycoproteins attached to it, which also assists pre-infection phase processes such as receptor attachment and virus-host cell fusion.3 Pre-infection precedes the synthesis stage, which is characterized by multiplication of viral progeny. This stage is highly variable among viruses and varies with respect to the genomic composition, structure, forms and polymerases being utilized.1 Virus synthesis is succeeded by the assembly of mature virus particles. Viral assembly utilizes different cellular compartments such as the E. R, Golgi apparatus, and cytoplasm and requires the assistance of proteins such as capsids to ensure proper spatial arrangement and proteases to convert inactive enzymes into active form for re-infection.2,4,5 Proper assembly triggers the release of virus particles, which lyse the host cells (non-enveloped viruses) utilizing either the secretory/exocytosis or budding mechanism (Fig. 1).6,7
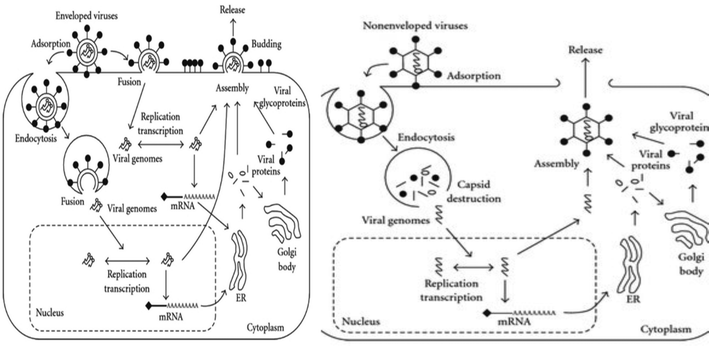 |
| Fig. 1 1.1. Stages of infection of enveloped viruses. Infectivity starts either with endocytosis or host cell-virus fusion. After successful viral entry, genome replication and viral protein synthesis take place. The synthesis stage is followed by the packaging of mature virus particles, which ultimately trigger the release of viruses via budding. 1.2. Infectivity of non-enveloped viruses follows a similar plan as that of enveloped viruses. However, the differences in viral entry (only endocytosis) and exit (lysis of host cells) are notable. (Figure Source: Function of Membrane Rafts in Viral Lifecycles and Host Cellular Response, Biochem. Res. Int. 2011, 2011, 245090 with permission.) | |
The devasting effects of viruses have been known throughout history. However, it is important to note that unravelling of the overshadowed beneficial side of viruses is a developing field of research. In the field of medicine, clinical trials involving the use of viruses (adenoviruses and lentiviruses) as cancer regressors owing to their cancer cell-specific tropism, resulting in oncolysis, and as virus-mediated gene therapy are yielding positive results.50–52 In plant systems, viral benefits include increased drought and cold resistance in plants infected with latent phase viruses such as brown mosaic virus (BMV) and cauliflower mosaic virus (CMV).53,54
2.1 Biology of SARS-CoV-2
SARS-CoV-2 (causative agent of COVID-19 pandemic), a member of the Coronaviridae family, is a large enveloped virus having a positive sense RNA genome.55 However, it lacks the enzyme hemagglutinin-esterase, which is associated with the closely linked A. Betacoronavirus.56 The genome of SARS-COV-2 is ∼30k base-pair long and has the following reading frame: 5′ UTR (cap), non-structural proteins (nsps), structural proteins, and accessory proteins, 3′ UTR (poly A tail). The non-structural proteins (nsp) are comprised of fifteen non-structural proteins, where among them, RNA-dependent RNA polymerase (nsp12), nsp7, nsp8, (co-factors of nsp7), helicase (nsp13), and exonuclease (nsp4)57,58 are vital for genomic replication and transcription.
The structural proteins (S, E, M, and N) are critical for host-virus cell receptor binding, virion assembly, morphogenesis and release of virus particles from the host cells.59,60 The S protein is crucial for binding and facilitates entry into the host cells. They are clove-shaped transmembrane in nature and can be distinguished into large ecto-domain, single pass transmembrane and intra-cellular tail. The ecto-domain is comprised of an S1 sub-unit, containing the receptor binding domain (RBD) and membrane fusion S2. Studies have found that the RBD of S1 binds to the ACE-2 (angiotensin converting enzyme-2) receptor and transmembrane serine protease 2 (TMPRSS2) in the case of SARS-Cov-2.61 The E protein is localised in the Golgi and ER in the host cell and is known to facilitate the formation of virus-like particles.60,62 The M glycoprotein also plays a major role in viral-assembly together with the E and N proteins. The N protein is responsible for packaging the viral genome into the helical nucleocapsid particles.63 Besides, there are some accessory proteins derived from the sub-genomic RNA and distributed among the structural genes.64,65
2.2 Failure of conventional anti-viral strategies
Mitigation of viral destruction necessitates the development of superior anti-viral strategies. The conventional anti-viral strategies designed thus far include anti-viral drugs, vaccines and RNA interference or RNAi-based anti-viral approach.66–69 However, despite their timely modifications, conventional anti-viral therapies have failed to keep up with highly evolving viruses, making it necessary to discover more promising anti-viral strategies.10,68,70
Anti-viral drugs are not as effective as anti-bacterial or anti-fungal drugs given that they perturb the viral developmental processes rather than targeting the pathogen directly. Anti-viral drugs such as Acyclovir, Favipiravir, and Remdesivir impede replication processes by participating as nucleotide precursors in the replication process, while drugs such as Saquinavir and Oseltamivir stop the maturation and assembly process due to the blockade of the active sites of proteases or other enzymes vital for this process.66,67 Despite the targeted action of anti-viral drugs, their use faces several set-backs, stressing the need to develop more advanced methods for the eradication of viruses.
Due to the narrow spectrum of anti-viral drugs, they fail to elicit a proper response for viruses belonging to the same family due to the complexity in genome and development processes. Owing to this narrow specificity, the severity of the current pandemic (COVID-19) was further aggravated given that Remdesivir, which is used to treat Middle East Respiratory Syndrome caused by SARS-CoV-1 and SARS-CoV-2 (in animals), failed to treat SARS-CoV-2 (causative agent) of COVID-19 in humans.11 Thus, the narrow specificity of anti-viral drugs mandates the use of anti-viral drugs in combination or together with immunotherapy (TNFα and IFNβ) to deplete the viral load. However, combination therapy further reduces the already low therapeutic index of anti-viral drugs and toxicity manifests in the form of various side effects such as nausea, intestinal nephritis and kidney damage.12,13,71 In addition, the anti-viral drugs currently used for the treatment of viral diseases can become ineffective at any time due to the development of resistant strains. Resistant mutations have been reported in all determinant viruses such as HIV, hepatitis, and influenza, thus nullifying the efficacy of the applied anti-viral therapy.8,9,72 Recently, the resistance mechanism conferring tolerance to Favipiravir (used currently for the treatment of Nipah virus) was found in influenza (H1N1), suggesting the possible re-emergence of the Nipah pandemic.10
Vaccination is carried out to evoke an immune response once the same pathogen is encountered, which has proven to be quite successful in counteracting viral diseases such as small pox, polio and chicken pox but still has a long way to go to confer protection against every existing viral disease encountered by an individual.14–16 Vaccination failure with respect to preparation, attenuation, dosage and route of administration is well documented. In addition, vaccine failure with respect to hosts is also a crucial factor, given that vaccines designed for infections such as measles, influenza, and hepatitis have failed to confer protection in certain individuals. Although the exact reasons for this remain unknown, factors such as genetic predisposition, obesity, and high proportion of T-reg cell subset in certain cases seem to be probable.14,15,17,73 Furthermore, due to the fact that vaccines are tested on young healthy individuals under optimal conditions, they also fail to confer protection in old-aged individuals, where the pool of humoral and cellular immunity is already decreasing, rendering them a higher risk for novel or resistant viral infections.16,74 In addition, vaccine development for novel viruses proceeds with a time lag to gain in depth knowledge of the viral biology and the designed vaccine may not evoke a sufficient immune response. It is noteworthy to mention with respect to this that the potential vaccine candidate ChAdOx1 developed against COVID-19 failed to induce a sufficient humoral immune response in animal models, resulting in the need of additional booster doses or another reliable anti-viral strategy.68
The RNAi or RNA interference method involves the synthesis of viral m-RNA complementary short-interference (si-RNA) from long dsRNA, where the resultant si-RNA then base pairs with viral m-RNA in viral-infected cells, leading to its degradation.69 si-RNA complementary to small stretches of m-RNA sequences of HIV, influenza, hepatitis B, etc. have been developed and used successfully.75,76 However, although RNAi technology is relatively advanced and successful, it also faces certain disadvantages, where among them, the inability of RNAi to cross the plasma membrane due to its high negative charge, its rapid renal clearance, enhanced phagocytosis and accelerated antibody-mediated immune response are prominent.77,78 Thus, based on the above-mentioned strategies, it can be concluded that there is still a gap for new approaches to treat virus infections. Among them, the use of nanomaterials is one emerging approach, which needs important consideration due to their inherent antiviral properties.
3. Anti-viral activities of nanomaterials
To overcome the limitations of conventional anti-viral therapies, the development of advanced anti-viral strategies is urgent. The designed anti-viral strategy must be reliable, with low cytotoxicity, resistance insensitive and must act in an age and co-morbidity independent manner. Accordingly, the exploration of the anti-viral potential of nanomaterials offers an exciting platform.40,45,79–81
3.1 Nanomaterials
Nanomaterials can be categorized as organic and inorganic, ranging in size from 1–100 nm. The characteristics of nanomaterials such as their quantum size effect, high surface area, high surface to volume ratio, and tunable surface charge have validated their role as excellent carriers of conventional anti-viral therapies.21,22 Additionally, the biomimetic properties of inorganic and metal nanoparticles have inspired researchers to investigate their intrinsic anti-viral activities.23
3.2 Organic nanoparticles
Organic nanomaterials are composed of polymers of PGA (polyglycolides), PLA (polylactides) or nanocapsules, liposomes, micelles and dendrites.79,82 Organic nanoparticles are either treated chemically (usually with PEG) or surface modified to encapsulate anti-viral drugs/vaccines, leading to their increased concentration at the target site, enhanced stability, and better retention time, thus forming an efficient drug delivery system.80,81 The quantum size of organic nanoparticles allow them to traverse the BBB (blood–brain barrier) and blood-testis barrier, which would otherwise be unable to cross and exhaust the viral titer.83 Organic nanoparticles also play a pivotal role in transporting si-RNA across the cell membrane by slowing down phagocytosis and impeding renal clearance.70
3.3 Inorganic nanoparticles
Metal nanoparticles or inorganic nanoparticles include nanoparticles of either native metals such as silver (Ag), gold (Au), and Tin (Sn) or metal oxides such as copper (Cu2O and CuO), zinc (ZnO), titanium (TiO2) and iron (Fe2O3 and Fe3O4).27–29,47 Besides acting as excellent carriers of anti-viral drugs or vaccines, inorganic nanoparticles execute their intrinsic anti-viral activity and curtail the pathogenicity of determinant viruses such as HIV, HSV, HINI, H3N2, and hepatitis A–E.27,31,36,46 The use of metal nanoparticles offers broad spectrum usage, resistance insensitivity, low cytotoxicity, and independence of comorbidities, and hence can be considered as an advanced replacement for conventional anti-viral strategies.30,32,84
4. Conventional (organic) anti-viral developments
The use of organic nanoparticles such as polymeric nanoparticles (PNP), micelles, liposomes, dendrites, and nano-vaccines has been around for quite a long time, succeeding in qualitatively and quantitatively enabling the direct and sustained release of conventional anti-viral strategies, i.e., drugs and vaccines.85 A brief account of their application is given below.
4.1 Polymeric nanoparticles (PNP)
Polymeric nanoparticles or PNPs are the most widely used nanoparticles in vivo, which are comprised of nanoparticles of natural proteins (collagen, gelatin, and albumin) or artificial polymers such as polylactic acid (PLA), polylactide-glycolic acid (PLGA), polystyrene, and polycaprolactone (PECL).86 Several modifications have been carried out to reduce the interactions of drugs with serum proteins and evade phagocytosis to enhance their pharmacokinetics.87 Two forms of PNPs exist, nanocapsules, in which the drug is localized in their cavity, and nanospheres, in which the drug is localized on their surface. A widely used nanocapsule is Solutol, which is capable of traversing the BBB and aiding the significant localization of indinavir.88
4.2 Micelles
Micelles are polymeric nanoparticles that are amphipathic in nature, i.e., one of their components (core) is hydrophobic and the other component (surface) is hydrophilic. The designed structure allows a high loading of hydrophobic drug by conjugating it with the core and the hydrophilic portion decreases the non-specific interactions with enzymes, etc.89,90 An in vivo example of this is the conjugation of acyclovir to the hydrophobic ε-capro-lactone and sequential addition of hydrophilic hydroxy polyethylene glycol, resulting in an enhanced drug-loading capacity.91
4.3 Liposomes
Liposomes are derived from phospholipids and range in size from 20–30 nm. They offer the advantage of conjugating both hydrophobic and hydrophilic drugs. Their other advantages include their bio-degradable nature, non-immunogenicity, easy availability, low cost and ability to restrict gastro-intestinal digestion.92
4.4 Solid lipid nanoparticles
Solid lipid nanoparticles (SLNs) with a size in the range of 10–100 nm are comprised of a solid lipid matrix, differing from liposomes in their aggregating status. They have been designed to achieve controlled drug release, improved stability, and targeted drug delivery.93 SLNs have been used for the delivery of ritonavir, maraviroc, darunavir, etc.94 Second-generation solid liquid nanoparticles are liquid lipid carriers having enhanced drug delivery and retention capacities.95
4.5 Dendrimers
Dendrimers are composed of a central core, inner shell made up of repeating units of building blocks, and outer shell composed of numerous functionalities.82 The structural complexity of dendrimers allows the encapsulation of several chemical moieties, displaying multiple surface groups.96,97 The commonly available dendrimers include poly-lysine (PLI), poly-amidoamine (PAMAM), and poly-propylene-imine (PPI) dendrimers.98 Dendrimers are functionalized mainly with three types of molecules, namely, carbohydrates, peptides, and anionic groups. Interestingly, these dendrimers possess inherent antimicrobial or antiviral activities. Dendrimers decorated with anionic groups interact with the V3 loop of the gp 120 protein and interfere with the early stages of viral replication. PPI dendrimers with randomly sulfated galactose residues and PLL dendrimers with sulfated galactose and cellobiose residues have demonstrated reasonable antiviral activity.99
5. Metal and metal oxide nanoparticles as anti-viral agents
Inorganic nanoparticles, unlike polymeric nanoparticles (PNPs), are not solely dependent on the loaded conventional anti-viral drug and vaccine to execute their anti-viral activity, but rather execute their intrinsic anti-viral potential to deplete the viral load.23,100 Inorganic nanoparticles, which are one-scale smaller than organic nanoparticles (1–100 nm), are partially or solely independent of the limiting uptake processes such as clathrin-mediated endocytosis, pinocytosis, and caveolin-mediated endocytosis, thereby gaining entry into host cells via van der Waals and electrostatic interactions, and thus are superior to PNPs as carriers.101 In the case of the intrinsic anti-viral activity of inorganic nanoparticles, they target vital steps such as viral entry and viral replication, evoke host responses, and cause agglutination of viral proteins, rendering them incapable of entry, and thus offering broad range, wide spectrum application, and resistance insensitivity unlike PNPs, which simultaneously become ineffective once the drug is transported and lose their efficacy.10,27,32,84
It is noteworthy to mention here that the in vivo application of inorganic nanoparticles is lower than that of the widely used PNPs. This is attributed to the associated toxicity/side-effects of metal nanoparticles, i.e., hepatotoxicity, nephrotoxicity, cardiotoxicity, genotoxicity, and cyanidation.102,103 With recent advancements in the field of nano-biotechnology, victorious attempts to decrease their associated cytotoxicity by balancing the concentration of nanoparticles and applying various coatings in the form of SiO2 (gold) and PEG (zinc) were successfully performed.29,34 Cases where metal nanoparticles have been administered in vivo to curtail viral pathogenicity include the administration of silver nanoparticles to attenuate the infectivity of the H3N2 virus in mice, decrease the capacity of HSV-2 to infect female Balb/c-mice vaginal tissue following prior incubation with ZnO nanoparticles and inhalation therapy of Ag-NPs for home quarantine patients to attenuate the pathogenesis of COVID-19.40,104,105
Detailed information on the anti-viral activity of various metal nanoparticles is described below.
5.1 Silver nanoparticles (Ag-NPs)
Ag-NPs possess superior anti-microbial properties such as parent silver (Ag) metal. Ag-NPs employ virucidal as well as virustatic mechanisms to halt the progression of HIV-1, HSV 1, 2 and 3, and Hepatitis-B, HIN1 and H3N2.27,28,31,35 Ag-NPs circumvent the infectivity of HIV-1 by blocking the binding between viral gp-120 and the host cell CD4 receptor. This interaction targets the least mutational negative loop of gp-120 unlike retroviral drugs, which target the highly mutational V3 loop of gp120 to prevent host cell binding, thus losing efficiency as resistant strains develop over time. A further decline in the HIV-1 pathogenesis occurs as Ag-NPs halt the reverse the transcription are translation processes by binding directly to viral DNA or RNA molecules.27 To annihilate HSV (1, 2 and 3) infectivity, Ag-NPs inhibit both viral entry by masking the sulfhydryl group of glycoproteins, which is necessary for host cell–viral envelope fusion and replication, by directly establishing contact with viral DNA.28,39 In this case, the coating of Ag-NPs with a tannic case increases their anti-viral activity against HSV.106
In the case of hepatitis B, Ag-NPs only exhibit virucidal activity by directly binding to HBV ds DNA, impeding the production of intracellular HBV RNA. This ultimately reduces the production of viral proteins, leading to declined infectivity.35
The mechanism by which Ag-NPs attenuate HINI pathogenicity is not clearly well understood. Research suggests that blockade of viral entry via binding to hemagglutinin could be the most probable mechanism.31 In addition to HINI, Ag-NPs diminish the infectivity of another influenza strain, H3N2, in in vitro and in vivo conditions by enacting the same mechanism.105 Recently, it was reported that the inhalation of silver nanoparticles at the initial stage of COVID-19 infection could manage symptoms in patients. Although the exact explanation for this could not be framed, inhibition of viral entry seemed to be the operational mechanism.40
5.2 Gold nanoparticles (Au-NPs)
Gold nanoparticles (Au-NPs), although not as popular as Ag-NPs owing to their relatively higher cellular toxicity, have shown promising results in circumventing infections of HIV-1, HSV-1, H1N1, H3N2 and adenoviruses.43,44,84 Various modifications to Au-NPs such as PEG coating, creation of porous gold particles and embedding Ag-NPs in a shell of inert molecules such as SiO2 have been routinely performed to reduce their associated cytotoxic effects.34,84 Ag-NPs also act as an efficient carrier of conventional anti-viral drugs, vaccines and interferons presently.84,107
Ag-NPs have been found to be successful in abrogating HIV-1 entry into host cells. Unmodified Ag-NPs and PEG-coated Ag-NPs mitigate viral entry by targeting the viral gp-120, rendering it incompatible to attach the host cell cluster of differentiation (CD4). To achieve this, Ag-NPs target the sulfhydryl groups of gp-120, causing protein denaturation and making them incompetent to attach CD4, in addition to being resistance insensitive.30,108 Ag-NPs uniformly distributed within a shell of silica dioxide (SiO2) attenuated the adsorption of adenoviruses (causative agent of gastrointestinal infections) to host cells.34 Porous gold particles (Po-Ag NPs) successfully tapered the infectivity of influenza virus strains HINI, H3N2, and H9N2. Perturbation of viral entry via tampering of the structure of disulphide bonds of hemagglutinin, which is crucial for viral fusion, is the proposed mechanism (Fig. 2).43,44
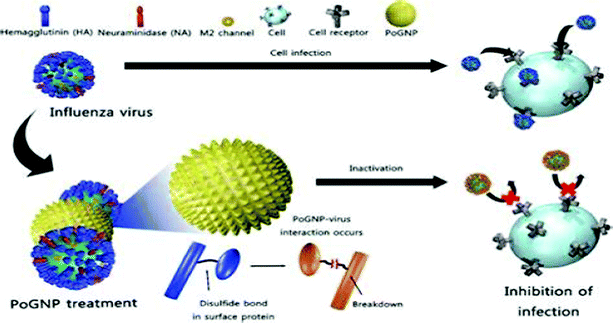 |
| Fig. 2 Porous gold nanoparticles (PoGNPs) have been used to attenuate infectivity of the HINI virus. PoGNPs prevent viral infectivity by interacting with the disulphide bonds of the enzyme hemagglutinin, the status of which plays a crucial role in establishing successful viral entry in cells. (Image source: Porous gold nanoparticles for attenuating infectivity of influenza A virus, J. Nano Biotechnol., 2020, 18, 54 with permission.). | |
The anti-viral approach of Ag-NPs towards HSV-1 is indirect. Ag-NPs are conjugated with mercaptoethanesulphonate (Au-MESNPs), which mimics the heparin sulphate binding site of HSV-1. Conjugating mercaptoethanesulphonate with Ag-NPs increases the binding valency between MES and HSV, resulting in polyvalent interaction. This indicates that Ag-NPs may also have viral binding sites and possibly possess intrinsic anti-viral activity against HSV.43
5.3 Copper oxide (CuO-NPs) nanoparticles
Copper was the first metal to be declared as an effective metallic anti-microbial agent in 2008 by the U.S. Environmental Protection Agency (EPA). The widely discovered anti-viral activity of copper compounds such as CuI (copper iodide), Cu2O (cuprous oxide), and CuO (cupric oxide) depends on the release of Cu2+ in solution. Cu2+ released in solution generates ROS, leading to the loss of genome integrity, lipid peroxidation and deactivation of viral enzymes, as indicated in Fig. 3. This mechanism was reported to attenuate the pathogenicity of HINI (pandemic-2009) with released Cu2+ ions deactivating the hemagglutinin and neuraminidase enzymes, which are vital for infectivity.32
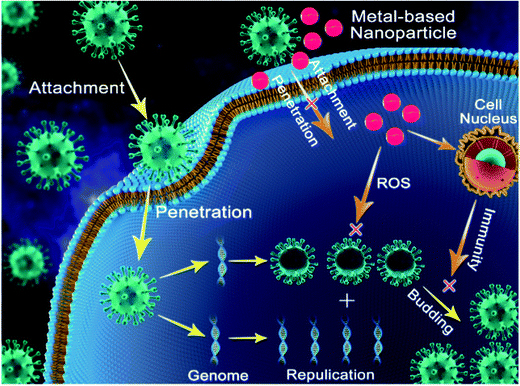 |
| Fig. 3 Pictorial representation of the general mechanisms adopted by metal nanoparticles to reduce the infectivity of viruses. Metal nanoparticles exhibit their activity by impeding viral entry into the cells, by inhibiting viral replication or transcription, inducing ROS-mediated cell death, and evoking host cell immune response. Image Source: Progress and Perspective of Antiviral Protective Material, Adv. Fiber Mater., 2, 123–139 (2020) with permission. | |
The intrinsic anti-viral activity of copper oxide (Cu2O and CuO) nanoparticles independent of the release of Cu2+ ions and ROS generation has been barely deciphered. CuO nanoparticles were first reported to be effective against HCV attachment and entry into host cells. Although their exact mechanism of entry inhibition is not well elucidated, the interaction of CuO nanoparticles with HCV glycoproteins is the hypothesized mode of action.36 However, no effect of CuO nanoparticles on HCV replication in this case was reported. Additionally, CuO nanoparticles have been found to suppress the infectivity of HSV in the replication stage by inhibiting the activity of one of the required transcription factors.29
At the beginning of the COVID-19 pandemic, various metallic surfaces were investigated for surface interaction with COVID-19. It was reported that the activity of COVID-19 on copper or on copper alloy was just 4 h. The mechanisms behind this phenomenon was the release of Cu2+ ions, leading to the loss of membrane integrity and genome fragmentation.45 This indicates that the application of a copper coating on various surfaces or use of copper nanoparticles of various sizes can be vital in curbing COVID and slowing down the pandemic.
5.4 Titanium dioxide (TiO2 NPs)
The virucidal activity of TiO2 NPs is initially dependent on photocatalysis, leading to ROS production under UV irradiation, which has become increasingly important with the discovery that TiO2 nanoparticles tethered the H3N2 envelope caused hemagglutinin and neuraminidase agglutination independent of visible light/UV irradiation.38 TiO2 nanoparticles also aid in viral clearance by stimulating immune cells to secrete pro-inflammatory cytokines (IL-6 and TNFα), leading to increased vascular permeability, chemotaxis, and increased production of antibodies, which contribute to the depletion of viral stocks. This strategy employing TiO2 for attenuating the virulence of gastrointestinal murine noroviruses also has immense potential to cure hepatitis B and influenza infections.37
5.5 Zinc oxide (ZnO NPs)
ZnO nanoparticles, despite their bactericidal activity, are still being explored for their anti-viral properties. Initially utilized just to increase the antibody titer for enhanced viral clearance in HSV, ZnO nanoparticles have recently been shown to exhibit intrinsic virucidal activity against HSV-1.46,109 In addition to HSV, ZnO nanoparticles have also successfully inhibited the replication of HINI in vitro.33 In both cases, PEG coating was done to reduce their cytotoxicity and elevate their efficiency.
5.6 Iron oxide (Fe2O3 and Fe3O4) nanoparticles
Super-magnetic maghemite (y-Fe2O3) and haematite (Fe3O4) iron oxide nanoparticles were conjugated to synthetic DNA aptamer, targeting the HCV E1E2 envelope glycoprotein of hepatitis C. Upon subjecting the plasma of infected patients to these particles, the virus load decreased drastically compared to that with the use of the DNA aptamer individually, owing to the strong magnetic field created by the iron nanoparticles.110 Iron nanoparticles also formed a very efficient delivery system for DNAzyme targeting the NS3 gene, where knockdown of NS3 is lethal to viruses owing to the termination of their replication process.111 However, the intrinsic anti-viral activity of iron oxide nanoparticles has been rarely reported. To date, only virustatic activity of iron nanoparticles (Fe3O4) against the influenza virus HINI has been reported. The proposed mode of activity involves the interaction between the sulfur-bearing groups of hemagglutinin and iron oxide particles.47
6. Summary and future perspectives
Thorough the introspection of the anti-viral activities of metal nanoparticles leads to the conclusion that the exploitation of the intrinsic anti-viral potential of metal nanoparticles is a promising strategy to prevent the havoc of future viral pandemics to a great extent. Strategies adopted by metal nanoparticles to subvert viral infectivity include inhibiting host cell–virus fusion, impeding genomic replication, evoking sufficient host immune response and bringing about a change in the virus structure, rendering it incapable of binding to host cells (Fig. 3). All these mechanisms act vividly and can be well-applied to diverse viruses without the need of in-depth knowledge of structural viral biology, and thus act as a ray of hope in the case of future pandemics.27,32,37 However, despite their numerous advantages, the use of metal nanoparticles suffers some limitations. The major limitation faced by metal nanoparticles is their associated cytotoxicity and genotoxicity (at higher concentrations).112 Upon reaching the human body, metal nanoparticles cross various barriers and are accumulated in vital organs such as the kidneys, brain, and lungs and trigger the production of ROS, which is a hallmark of oxidative stress and inflammation. Accumulation of ROS in cells leads to cell death, damage to proteins and lipids, etc.113,114 However, it is important to know that the cytotoxicity of metal nanoparticles can be averted greatly by adopting simple measures such as decreasing the size and dose of metal nanoparticles, favoring simple shapes such as spheres over complex ones such as star shape to minimize their surface area, and coating metal nanoparticles with molecules such as citrate, tannic acid, and mercapto-succinic acid.115–119 With proper implementation of the above-mentioned measures to reduce the cytotoxicity of metal nanoparticles, the field of metal nanobiotechnology can be extended to the frontline to reduce the wrath of viral diseases and potent future pandemics. It is also necessary to properly implement in vitro anti-viral findings of metal nanoparticles on in vivo subjects (mice) first before implementation on human volunteers. With the proper implementation of the above-mentioned strategy, not only viral diseases but also cases of cytotoxicity can be handled successfully.102,105
Based on the thorough study of the structural biology of COVID-19 and its pathogenesis and the anti-viral potential of metal nanoparticles, we believe that the current global pandemic (COVID-19) can be treated to a great extent via the application of nanoparticles through different approaches. This can be summarized as follows.
6.1 Blocking transmission/rendering COVID incapable of binding to host cell
Considering that to date, no conventional anti-viral drug seems to completely mitigate the progression of COVID-19, people are left with no option other than minimizing human to human contact and mandatory use of masks for normal people and protective gear or personal protective equipment (PPE) kits for health professionals given that the route of transmission is via droplets of infected persons.11,68,71 PPE kits or face masks prevent bacteria and virus particles from droplets of infected persons from contacting healthy persons. However, reports suggest that during/after the discard of these materials, some infectious particles find their way to other hosts, leading to widespread transmission. It has been suggested that nanocoating of PPE kits or masks with disinfectant and with positively charged materials can immediately kill the virus and increase their filtering power.48,120 Recent advances in nanotechnology allow both problems to be solved simultaneaously. In the past, copper oxide-based filters were used to block the transmission of HIV in media.121 Thus, we extended this concept and suggest the incorporation of a film of positively charged metal nanoparticles such as Ag-NPs, Au-NPs and CuO-NPs on protective gear. The work carried out by Sarah Warners et al. supports our hypothesis, suggesting the ROS-mediated cell death of coronavirus on copper-coated surfaces.45
6.2 Inhibiting viral-host cell fusion
Entry of SARS-CoV-2 (COVID-19) into host cells is attributed to the binding of the RBD domain of the S1 protein to ACE-2. Analysis of amino-acids forming the binding region suggests that it is composed of hydrophobic and negatively charged amino-acids and binding is mediated by van der Waals and electrostatic interactions.122,123 Here, we hypothesize that silver nanoparticles (Ag-NP's) can electrostatically interact with the RBD of S1 to block this interaction. Supporting our hypothesis, Oron Zachar found that the inhalation of silver nanoparticles during the initial stage of infection can block viral entry by perturbing the entry step.40 Further, it has been demonstrated that SiO2 NPs can scavenge free virus particles and prevent them from binding to host cells. This binding is universal for enveloped viruses, making them potential targets for SARS-CoV-2.49
6.3 Inhibiting genomic replication
The genetic material of COVID-19 is single-stranded RNA. Much evidence in the literature suggests that the synthesis of single-stranded RNA can be impeded via nanoparticles such as gold (Ag-NPs) and silver (Au-NP's). With regard to this, it was demonstrated that treating PEDV (commonly studied model of coronavirus) with glutathione-capped Ag2S nanoclusters suppressed the synthesis of genomic RNA.41
6.4 Evoking sufficient host response
Reports suggest that the inhibition of COVID-19 infection can be treated exceptionally well if the host immune system is aptly stimulated. Ag-NP-modified GO (graphene oxide) was reported to exhibit 59.2% inhibitory efficiency of COVID-19 via proper stimulation of IFN in cells and inhibition of viral infection-induced ROS production.42
6.5 Aiding conventional anti-viral drugs for targeted action
After gaining insights into the COVID-19 biology, various drugs that perturb different developmental stages of the virus life cycle were approved by the FDA. The approved drugs include chloroquine, which targets fusion of the S2 protein, the corticosteroid Ciclesonide, blocking RNA replication by targeting nsp15, Ivermectin, targeting RNA replication, and Remdesivir, targeting RNA-dependent RNA polymerase.124–127 However, the approved drugs are eventually discontinued owing to the number of side effects manifested by patients.71 This problem could be overcome by specifically targeting anti-viral drugs to a certain location. A case in which the conventional drug-carrying property of metal/organic nanoparticles was utilized to aid COVID-19 drug delivery is the transport of chloroquine by encapsulating it in poly-lactic acid (PLA) polymers.128 Further, the field of conventional nanoparticles is still open to enable the transport of conventional designed anti-viral drugs in the case that severe symptoms arise in COVID-19 subjects.
6.6 Nano biosensors for antiviral detection
Polymerase chain reaction (PCR) is the major technique used for the detection of COVID-19. However, it is a time-consuming technique, which also exhibits 70–80% accuracy. Moreover, sample collection (nasal swab) is highly painful in PCR. Accordingly, various efforts have been focused on the development of nanobiosensor devices for selective and specific detection with a lower limit of detection.129,130 However, it is still necessary to develop highly promising saliva-based compatible and ultrafast techniques to detect COVID-19.
Conflicts of interest
There are no conflicts to declare.
References
- M. Marsh and A. Helenius, J. Cell, 2006, 124, 729–740 CrossRef CAS PubMed.
- M. Novikova, Y. Zhang, E. O. Freed and K. Peng, Virol. Sin., 2019, 34, 119–134 CrossRef CAS PubMed.
-
J. J. Skehel and D. C. Wiley, Annu. Rev. Biochem., 2000, 69, 531–569 Search PubMed.
- J. Konvalinka, H.-G. Kräusslich and B. Müller, Virology, 2015, 479–80, 403–417 CrossRef PubMed.
- D. M. Jones and J. Mc Lauchlan, J. Biol. Chem., 2005, 285(30), 22733–22739 CrossRef PubMed.
- I. B. Hogue, J. Scherer and L. W. Enquist, mBio, 2016, 7(3), e00820-16 CrossRef PubMed.
- J. M. White, Science, 1992, 258, 917–924 CrossRef CAS PubMed.
- T. L. Kieffer and S. George, Curr. Opin. Virol., 2014, 8, 16–21 CrossRef CAS PubMed.
- M. Samson and A. Pizzorno, Antiviral Res., 2013, 98(2), 174–185 CrossRef CAS PubMed.
- D. H. Goldhill and A. J. W. Te, Proc. Natl. Acad. Sci. U. S. A., 2018, 115(45), 11613–11618 CrossRef CAS PubMed.
- Y. Wang, D. Zhang, G. Du, R. Du, J. Zhao and Y. Jin,
et al., Lancet, 2020, 395, 1569–1578 CrossRef CAS.
- J. Y. Feng, E. Murakami and S. M. Zorca, Antimicrob. Agents Chemother., 2004, 48(4), 1300–1306 CrossRef CAS PubMed.
- J. W. Hanes, Y. Zhu, D. S. Parris and K. A. Johnson, J. Biol. Chem., 2007, 282(34), 25159–25167 CrossRef CAS PubMed.
- A. K. Kubba, P. Taylor, B. Graneek and S. Strobel, Commun. Dis. Public Health, 2003, 6(2), 106–112 CAS.
- C. M. Gelder, R. Lambkin, K. W. Hart and D. Fleming, J. Infect. Dis., 2002, 185, 114–117 CrossRef CAS PubMed.
- D. Boraschi and P. Italiani, Immunol. Lett., 2014, 162(1), 346–353 CrossRef CAS PubMed.
- K. M. Young and C. M. Gray, PLoS One, 2013, 8, e82779 CrossRef PubMed.
- G. A. Naikoo, G. A. Naikoo and A. Qurashi, IEEE Sens. J., 2021, 21(16), 17643–17656 CAS.
- S. S. Bhat, A. Qurashi and F. A. Khanday, TrAC, 2017, 86, 1–13 CAS.
- M. I. Haque Ansari, S. Hassan, A. Qurashi and F. A. Khanday, Biosens. Bioelectron., 2016, 85, 247–260 CrossRef CAS PubMed.
- S. E. Mc Neil, Mol. Biol., 2011, 697, 3–8 CAS.
- R. A. Petros and J. M. Desimone, Nat. Rev. Drug Discovery, 2010, 9, 615–627 CrossRef CAS PubMed.
- M. Gagliardi, Ther. Delivery, 2017, 8, 289–299 CrossRef CAS PubMed.
- S. Rehman, S. M. Asiri, F. A. Khan and A. Qureshi, Sci. Rep., 2020, 10, 3228 CrossRef CAS PubMed.
- S. Rehman, S. Asiri, F. A. Khan, R. Jermy, H. Khan, S. Akhtar, R. Jindan, K. Khan and A. Qurashi, ChemistrySelect, 2019, 4, 4013–4017 CrossRef CAS.
- G. A. Naikoo, M. Mustaqeem, I. U. Hassan, T. Awan, F. Arshad, H. Salim and A. Qurashi, J. Saudi Chem. Soc., 2021, 25(9), 1319–6103 Search PubMed.
- H.
H. Lara, N. V. Ayala-Núnez, L. D. C. Ixtepan Turrent and C. Rodríguez Padilla, J. Nanobiotechnol., 2010, 8, 1 CrossRef PubMed.
- S. Gaikwad and A. Ingle, Int. J. Nanomed., 2013, 8, 1 CrossRef PubMed.
- A. Tavakoli and M. S. Hashemzadeh, J. Virol. Methods, 2020, 275, 113688 CrossRef CAS PubMed.
- S. Vijayakumar and S. Ganesan, Curr. HIV Res., 2012, 10(8), 643–646 CrossRef CAS PubMed.
- D. Xiang, Q. Chen, L. Pang and C.-L. Zheng, J. Virol. Methods, 2011, 178(1-2), 137–142 CrossRef CAS PubMed.
- Y. Fujimori and T. Sato, Appl. Environ. Microbiol., 2012, 78(4), 951–955 CrossRef CAS PubMed.
- H. Ghaffari, A. Tavakoli, A. Moradi and A. Tabarraei, J. Biomed. Sci., 2019, 26(1), 70 CrossRef PubMed.
- V. Lysenko and V. Lozovski, J. Nanosci. Nanotechnol., 2018, 9(2), 025021 Search PubMed.
- L. Lu, R. Wai, Y. Sun, R. Chen and C.-K. Hui, Antiviral Ther., 2008, 13(2), 253–262 CAS.
- X. Hang and H. Peng, J. Virol. Methods, 2015, 222, 150–157 CrossRef CAS PubMed.
- S. Agnihothram and L. Mullis, Int. J. Nanotechnol. Eng. Med., 2016, 1(3), 63–73 CrossRef PubMed.
- N. A. Mazurkovaa and Y. E. Spitsyna, Nanotechnol. Russ., 2010, 5, 417–420 CrossRef.
- R. L. Hu and S. R. Li, Genetics Mol. Res., 2014, 13(3), 7022–7028 CrossRef CAS PubMed.
-
O. Zachar, Formulations for COVID-19 Treatment via Silver Nanoparticles Inhalation Delivery, 2020 Search PubMed.
- T. Du, J. Liang and N. Dong, ACS Appl. Mater. Interfaces, 2018, 10, 4369–4378 CrossRef CAS PubMed.
- T. Du, J. Liang and N. Dong, Carbon, 2016, 110, 278–285 CrossRef CAS.
- D. B. Pinto, S. Shukla and A. Gedanka, Wiley Interdiscip. Rev.: Comput. Mol. Sci., 2010, 6(9), 1044–1050 Search PubMed.
- J. Kim, M. Yeom and T. Lee, J. Nano Biotechnol., 2020, 18, 54 CrossRef CAS PubMed.
- S. L. Warnes, Z. R. Little, S. L. Warnes, Z. R. Little and C. W. Keevil, mBio, 2015, 6, 1697 CrossRef PubMed.
- T. E. Antoine, S. Hadigal and A. Yakoub, J. Immunol., 2016, 196(11), 4566–4575 CrossRef CAS PubMed.
- R. Kumar and M. Nayar, J. Infect. Chemotherapy, 2019, 25(5), 325–329 CrossRef CAS PubMed.
- Y. Li, P. Leung, L. Yao, Q. Song and E. Newton, J. Hosp. Infect., 2006, 62, 58 CrossRef CAS PubMed.
- L. A. Osminkina, V. Y. Timoshenko, I. P. Shilovsky, G. V. Kornilaeva, S. N. Shevchenko, M. B. Gongalsky, K. P. Tamarov, S. S. Abramchuk, V. N. Nikiforov, M. R. Khaitov and E. V. Karamov, J. Nanopart. Res., 2014, 16, 2430 CrossRef.
- J. Bell and G. McFadden, Cell Host Microbe, 2014, 15, 260–265 CrossRef CAS PubMed.
- J. Pol, G. Kroemer and L. Galluzzi, Oncoimmunology, 2016, 5(1), e1115641 CrossRef PubMed.
- M. A. Kotterman, T. W. Chalberg and D. V. Schaffer, Annu. Rev. Biomed., 2016, 17(1), 63–89 CrossRef PubMed.
- M. J. Roossinck, Nat. Rev. Microbiol., 2011, 9, 99–108 CrossRef CAS PubMed.
- M. J. Roossinck, PLoS Pathog., 2013, 9(5), e1003304 CrossRef CAS PubMed.
- F. Li, Annu. Rev. Virol., 2016, 3(1), 237–261 CrossRef CAS PubMed.
- J. F. Chan, K. H. Kok, Z. Zhu, H. Chu and K. K. To, Emerging Microbes Infect., 2020, 9, 221–236 CrossRef CAS PubMed.
- A. Wu, Y. Peng, B. Huang, X. Ding and X. Wang, Cell Host Microbe, 2020, 27, 325–328 CrossRef CAS PubMed.
- E. J. Snijder, E. Decroly and J. Ziebuhr, Adv. Virus Res., 2016, 96, 59–126 CAS.
- H. Hofmann and S. Pohlmann, Trends Microbiol., 2004, 12, 466–472 CrossRef CAS PubMed.
- Y. L. Siu, K. T. Teoh, J. Lo, C. M. Chan and F. J. Kien, Virol., 2008, 82, 11318–11330 CrossRef CAS PubMed.
- M. Hoffmann, H. Kleine-Weber, S. Schroeder, N. Kruger and T. Herrler, Cell, 2020, 181, 271–280 CrossRef CAS PubMed , e278.
- J. L. Nieto-Torres, M. L. Dediego, E. Alvarez, J. M. Jimenez-Guardeno and J. A. Regla-Nava, Virology, 2011, 415, 69–82 CrossRef CAS PubMed.
- Y. R. Guo, Q. D. Cao, Z. S. Hong, Y. Y. Tan and S. D. Chen, Mil. Med. Res., 2020, 7, 11 CAS.
- C. Wang, Z. Liu, Z. Chen, X. Huang and M. Xu, J. Med. Virol., 2020, 92(6), 667–674 CrossRef CAS PubMed.
- D. X. Liu and T. S. Fung, Antiviral Res., 2014, 109, 97–109 CrossRef CAS PubMed.
- J. W. Gnann Jr, N. H. Barton and R. J. Whitley, Pharmacotherapy, 1983, 5, 275–283 CrossRef PubMed.
- B. E. Davies, J. Antimicrob. Chemother., 2010, 65(2), 5–10 Search PubMed.
- S. P. Graham, R. K. McLean, A. J. Spencer, S. Belij-Rammerstorfer, D. Wright, M. Ulaszewska, J. C. Edwards, J. W. Hayes, V. Martini, N. Thakur and C. Conceicao, npj Vaccines, 2020, 395(1569), 1578 Search PubMed.
- W. Filipowicz, Cell, 2005, 122, 17–20 CrossRef CAS PubMed.
- N. Toub and C. Malvy, Biomed. Pharmacother., 2006, 60(9), 607–620 CrossRef CAS PubMed.
- I. F.-N. Hung, K.-C. Lung and E. Y.-K. Tso, Lancet, 2020, 395, 1695–1704 CrossRef CAS.
- R. Shafer, S.-Y. Rhee and D. Pillay, AIDS, 2007, 21, 215–223 CrossRef CAS PubMed.
- H. L. Bock, J. Kruppenbacher, R. Sanger, W. Hobel, R. Clemens and W. Jilg, Arch. Intern. Med., 1996, 156, 2226–2231 CrossRef CAS PubMed.
- B. Grubeck-Loebenstein, J. Comput. Pathol., 2010, 142, 116–119 CrossRef PubMed.
- J. J. Rossi, Biotechniques, 2006, 25–29 CrossRef PubMed.
- M. Nakazawa, S. E. Kadowaki, I. Watanabe, Y. Kadowaki, M. Takei and H. Fukuda, Antiviral Res., 2008, 78, 10524–10538 CrossRef PubMed.
- S. K. Adesina and E. O. Akala, Mol. Pharm., 2015, 12, 4175–4187 CrossRef CAS PubMed.
- B. M. Chen, Y. C. Su and C. J. Chang, Anal. Chem., 2016, 88(22), 10661–10666 CrossRef CAS PubMed.
- M. Stevanovic and D. Uskokovic, Curr. Nanosci., 2009, 5, 1–14 CrossRef CAS.
- M. J. Santos-Martinez and K. Rahme, J. Biomed. Nanotechnol., 2014, 10, 1004–1015 CrossRef CAS PubMed.
- A. Gabizon, R. Catane and B. Uziely, Cancer Res., 1994, 54, 987–992 CAS.
- A.-M. Caminade, R. Laurent and J.-P. Majoral, Adv. Drug Delivery Rev., 2005, 57, 2130–2146 CrossRef CAS PubMed.
- S. D. Mahajan, R. Aalinkeel and W.-C. Law, Int. J. Nanomed., 2012, 7, 5301–5314 CrossRef CAS PubMed.
- R. Kesarkar and G. Oza, J. Microbiol. Biotechnol., 2012, 2(2), 276–283 CAS.
- H. Zazo, C. I. Colino and J. M. Lanao, J. Controlled Release, 2016, 224, 86–102 CrossRef CAS PubMed.
-
S. Kaushik, Handb Polym Ceram Nanotechnol. Cham, Springer International Publishing, 2020, pp. 1–17 Search PubMed.
-
E. Ratemi, Stimuli Responsive Polymeric. Nanocarriers Drug Delivery Applications., 2018, 1st edn, pp. 121–141 Search PubMed.
- M. P. De Oliveira, E. Garcion and N. Venisse, Pharm. Res., 2005, 22, 1898–1905 CrossRef PubMed.
- H. Cabral, K. Miyata, K. Osada and K. Kataoka, Chem. Rev., 2018, 118, 6844–6892 CrossRef CAS PubMed.
- A. Mandal, R. Bisht, I. D. Rupenthal and A. K. Mitra, J. Controlled Release, 2017, 248, 96–116 CrossRef CAS PubMed.
- A. J. Sawdon and C.-A. Peng, Colloids Surf., B, 2014, 122, 738–745 CrossRef CAS PubMed.
- T. Li, D. Cipolla, T. Rades and B. J. Boyd, J. Controlled Release, 2018, 288, 96–110 CrossRef CAS PubMed.
- V. Mishra, K. Bansal, A. Verma, N. Yadav, S. Thakur and K. Sudhakar, Pharmaceutics, 2018, 10, 191 CrossRef CAS PubMed.
- V. Makwana, R. Jain, K. Patel, M. Nivsarkar and A. Joshi, Int. J. Pharm., 2015, 495, 439–446 CrossRef CAS PubMed.
-
R. Müller and A. Ulrike, Nanostructured Lipid Carriers (NLC): The Second Generation of Solid Lipid Nanoparticles, in Percutaneous Penetration Enhancers Chemical Methods in Penetration Enhancement, ed. N. Dragicevic and H. Maibach, Springer, Berlin, Heidelberg, 2016, pp. 161–185 Search PubMed.
- D. A. Tomalia, Mater. Today, 2005, 8, 34–46 CrossRef CAS.
- S. Tripathy and M. K. J. Das, Appl. Pharm. Sci., 2013, 3, 142–149 Search PubMed.
- T. Garg, O. Singh, S. Arora and R. S. R. Murthy, Int. J. Pharm. Sci. Rev. Res., 2013, 7, 211–220 Search PubMed.
- R. D. Kensinger, B. J. Catalone, F. C. Krebs, B. Wigdahl and C.-L. Schengrund, Antimicrob. Agents Chemother., 2004, 48, 1614–1623 CrossRef CAS PubMed.
- N. Sanvicens and M. P. Marco, Trends Biotechnol., 2008, 26, 425–433 CrossRef CAS PubMed.
- D. A. Kuhn, D. Vanhecke and B. Michen, Beilstein J. Nanotechnol., 2014, 5, 1625–1636 CrossRef PubMed.
- S. Ahadian and M. Radisic, Woodhead Publishing, 2017, 12, 233–248 Search PubMed.
- A. Sereemaspun, R. Rojanathanes and V. Wiwanitkit, Ren. Fail., 2008, 30, 323–325 CrossRef CAS PubMed.
- A. Agelidis, L. Koujah and R. Suryawanshi, Front. Immunol., 2019, 10, 500 CrossRef CAS PubMed.
- D. Xiang and Y. Zheng, Int. J. Nanomed., 2013, 8(1), 4103–4114 CrossRef PubMed.
- P. Orlowski, E. Tomaszewska and M. Gniadek, PLoS One, 2014, 9(8), e104113 CrossRef PubMed.
- S. Yasri and V. Wiwanitkit, J. Coast. Life Med, 2014, 2754–2761 Search PubMed.
- R. Kesarkar, S. Shroff, M. Yeole and A. J. Chowdhary, Immunol. Virol, 2015, 1, 1 Search PubMed.
- A. Tavakoli and A. Ataei-Pirkooh, Nanomedicine, 2018, 13(21), 2675–2690 CrossRef CAS PubMed.
- N. Delaviz, P. Gill, A. Ajami and M. Aarabi, RSC Adv., 2015, 5, 79433–79439 RSC.
- S. R. Ryoo and H. Jang, Biomaterials, 2012, 33(9), 2754–2761 CrossRef CAS PubMed.
- X. He, W. G. Aker, P. P. Fu and H.-M. Hwang, Environ. Sci.: Nano, 2015, 2, 564–582 RSC.
- J. Fard, S. Jafari and M. Eghbal, Adv. Pharm. Bull., 2015, 5, 447–454 CrossRef CAS PubMed.
- P. Fu, Q. Xia, H. Hwang, P. Ray and H. Yu, J. Food Drug Anal., 2014, 22, 64–75 CrossRef CAS PubMed.
- A. Gliga, S. Skoglund, I. Wallinder, B. Fadeel and H. Karlsson, Part. Fibre Toxicol., 2014, 11, 11 CrossRef PubMed.
- M. Z. Rizk, S. A. Ali, M. A. Hamed, N. S. El-Rigal, H. F. Aly and H. H. Salah, Biomed. Pharmacother., 2017, 90, 466–472 CrossRef CAS PubMed.
- K. P. Steckiewicz, E. Barcinska, A. Malankowska, A. Zauszkiewicz-Pawlak, G. Nowaczyk, A. Zaleska-Medynska and I. Inkielewicz-Stepniak, J. Mater. Sci.: Mater. Med., 2019, 30, 22 CrossRef PubMed.
- B. C. Nelson, E. J. Petersen, B. J. Marquis, D. H. Atha, J. T. Elliott, D. Cleveland, S. S. Watson and I.-H. Tseng, Nanotoxicology, 2013, 7, 21–29 CrossRef CAS PubMed.
- M. C. Senut, Y. Zhang, F. Liu, A. Sen, D. M. Ruden and G. Mao, Small, 2016, 12, 631–646 CrossRef CAS PubMed.
- M. Iwata, H. Tanizaki, H. Fujii, Y. Endo and A. Fujisawa, Acta Derm. Venereol., 2015, 95, 628 CrossRef PubMed.
- G. Borkow, H. Humberto and L. Chandice, Antimicrob. Agents Chemother., 2008, 518–525 CrossRef CAS PubMed.
- J. Lan, J. Ge and J. Yu, Nature, 2020, 581, 215–220 CrossRef CAS PubMed.
- F. Li, W. Li, M. Farzan and S. C. Harrison, Science, 2009, 309, 1864–1868 CrossRef PubMed.
- T. Hu, M. Frieman and J. Wolfram, Nat. Nanotechnol., 2020, 15, 247–249 CrossRef CAS PubMed.
- S. Matsuyama, M. Kawase and N. Nao, bioRxiv, 2015, 95, 1 Search PubMed.
- L. Caly, J. D. Druce and M. G. Catton, Antivir. Res., 2020, 178, 104787 CrossRef CAS PubMed.
- M. L. Agostini, E. L. Andres and A. C. Sims, mBio, 2018, 9 Search PubMed.
- F. R. Lima, Pharmaceutics, 2018, 10, 255 CrossRef PubMed.
- G. A. Naikoo, T. Awan, I. U. Hassan, H. Salim, F. Arshad, W. Ahmed, A. M. Asiri and A. Qurashi, IEEE Sens. J., 2021, 21, 17643–17656 CAS.
- G. Seo, G. Lee, M. J. Kim, S. H. Baek, M. Choi, K. B. Ku, C. Lee, S. Jun, D. Park, H. Kim, S. Kim, J. Lee, B. T. Kim, E. C. Park and S. Kim, ACS Nano, 2020, 14, 5135–5142 CrossRef CAS PubMed.
|
This journal is © The Royal Society of Chemistry 2022 |