DOI:
10.1039/D1LC00801C
(Paper)
Lab Chip, 2022,
22, 47-56
Manipulation of cancer cells in a sessile droplet via travelling surface acoustic waves†
Received
7th September 2021
, Accepted 15th November 2021
First published on 25th November 2021
Abstract
The behaviours of microparticles inside a sessile droplet actuated by surface acoustic waves (SAWs) were investigated, where the SAWs produced an acoustic streaming flow and imparted an acoustic radiation force on the microparticles. The Rayleigh waves formed by a comb-like interdigital transducer were made to propagate along the surface of a LiNbO3 substrate in order to allow the manipulation of microparticles in a label-free and non-contact manner. Polystyrene microparticles were first employed to describe the behaviours inside a sessile droplet. The influence of the volume of the sessile droplet on the behaviours of the microparticles was examined by changing the contact angle of the droplet. Next, cancer cells were suspended in a sessile droplet, and the influence of contact angle on the behaviours of the cancer cells was investigated. A long gelation time was afforded by using a PEGylated fibrin gel. A primary tumour was mimicked by patterning the cancer cells to be concentrated in the middle of the sessile droplet. The non-contact manipulation property of acoustic waves was indicated to be biocompatible and enabled a structure-free platform configuration. Three-dimensional aggregated culture models were observed to make the cancer cells display an elevated expression of E-cadherin. The efficacy of the anticancer drug tirapazamine increased in the aggregated cancer cells, attributed to the low levels of oxygen in this formation of cancer cells.
Introduction
Experiments using microfluidics are recognized to be effective because they usually involve the use of small sample volumes and can be conducted at a higher throughput compared to conventional bulk experiments. Since the volumes of reagents or samples used in microfluidics are commonly on the order of microliters to nanoliters, it is necessary to handle objects (cells, droplets, and particles) within small volumes in microfluidics experiments. Compared to in vivo models, conventional two-dimensional (2D) cell culture systems have disadvantages such as their lack of the ability to embody discrete matrix fibrils, having extracellular matrices (ECMs) with low stiffness values, and being composed of cells not displaying any polarity with respect to the 3D microenvironment.1 This set of discrepancies results in disparities in a wide range of phenotypes including drug responses. In vitro microenvironment models by manipulating cells in a microfluidic system is crucial for simulating physiological conditions and obtaining similar drug responses.2 However, the need for a microchannel fabrication process and a syringe pump in most cases makes the system complex, poorly portable, and relatively difficult to operate.3 A simpler method to manipulate cells involves using surface acoustic waves (SAWs). Acoustofluidic manipulation in a sessile droplet minimizes the size of the external apparatus and the complexity of the microfluidic channels. Utilizing sessile droplet enables photolithography-/soft lithography-free microfluidic experiments and is easy to achieve in regular laboratory. The acoustofluidic manipulation in a sessile droplet can be applied to sample mixing,4 enrichment,5 separation,6,7 and detection,8,9 as well as to other biological assays.10,11
SAWs can be deployed in microfluidics over a wide range of frequencies (10 to 900 MHz) according to the design of the interdigital transducer (IDT) used.12 Unlike the case for other actively used methods (electronics13–15 and magnetics16), SAWs propagate through the medium deployed; hence, samples suspended in a sessile droplet are influenced, in a non-contact manner, by inhomogeneous wave scattering and streaming flow-induced drag forces that attenuate the wave intensity.17 SAWs take advantage of a higher degree of freedom with two forces: drag force caused by acoustic streaming flow (ASF) and acoustic radiation force (ARF).18 Several studies on sample mixing,4 separation,6,7 and other manipulations9,10,19 inside individual sessile droplets have been conducted using SAWs. Recently, Destgeer et al.6 investigated the motion of polystyrene (PS) microparticles suspended in a sessile droplet of water actuated by SAWs, which produced an ASF and imparted an ARF on the microparticles. They categorized four distinct regimes of particle patterning, specifically those that depend on the microparticle diameter, the SAW frequency, the acoustic wave attenuation length, and the droplet volume. The ASF-induced drag force, travelling wave-induced ARF, standing wave-induced ARF, and centrifugal force were used in various combinations to produce these distinct regimes. Furthermore, they used SAWs to investigate particle separation inside a sessile droplet of differing contact angles.7 Akther et al.20 increased the sample concentration by applying travelling SAWs to the side of the cylindrical closed chamber. Studies including microphysiological systems were conducted to form tumour spheroids using standing waves formed by overlapping two counter-propagating SAWs.21–23 In contrast to conventional ultra-low attachment surface culture systems, forming tumour spheroids by trapping cells in pressure nodes has been found to display the advantages of being rapid and allowing for a high throughput.24 Aggregating cancer cells by generating an ASF shows the advantages of being quick and simple.11 The cell aggregation formed using these methods showed phenotypes as a complementary in vitro system. Unlike ideal materials such as PS microparticles, cells are not standardized in size and the acoustic impedance contrast against water is small; these two features make it difficult to manipulate cells in water, so detailed investigations are required. In dealing with travelling SAWs, the behaviours of actual cells suspended in a sessile droplet are not yet fully understood and must be investigated in greater detail.
The objective of the present study is to investigate the behaviours of PS microparticles and cancer cells inside a sessile droplet of various contact angles by using travelling SAWs to generate ASF and ARF. First, PS microparticles were employed to describe the behaviours of particles inside a sessile droplet. The influence of the contact angle on the behaviours of objects inside the sessile droplet was examined by changing the voltage applied to the IDT and the contact angle of the sessile droplet on a surface-treated substrate. For a given contact angle, the combined effects of the ASF and ARF acting on the microparticles in the sessile droplet could be demonstrated. Next, cancer cells were suspended in a sessile droplet, and the dependence of the behaviours on the contact angle was investigated in detail. Even when travelling SAWs of the same frequency were applied, the behaviours of the ideal model with PS microparticles and the model with cancer cells were different depending on the contact angle. A long gelation time was afforded by using a PEGylated fibrin gel. The tumour primary site was mimicked by patterning the cancer cells concentrated in the middle of the sessile droplet. The comparative analysis was complemented by obtaining drug responses from tumour models of a conventional system and those of a rapidly formed in vitro system in a non-contact state using travelling SAWs. The application of the cancer cell model using travelling SAWs administered to a microfluidic system is expected to be of great help for cancer therapy as it can be applied to designing functional tissues, human disease models, or high-throughput drug screening models.
Experimental
Interdigital transducer (IDT)
For IDT fabrication, comb-like IDT fingers were deposited on a 128°-rotated Y-cut X-propagating LiNbO3 wafer (MTI Korea, Korea) with a 300 Å-thick Cr layer and a 1000 Å-thick Au layer by carrying out photolithography, electron-beam evaporation, and lift-off processes. The IDT, which was made to generate bidirectionally propagating Rayleigh waves, consisted of 20-finger pairs. The width of each electrode, at 49.9 μm, was designed to correspond to a quarter of the wavelength, and the length of the aperture was 1.2 mm. A vector network analyzer (TTR503A, Tektronix, USA) was used to measure the scattering parameter (S11) for finding the actual resonance frequency of the fabricated IDT (Fig. S1†). The SAWs were generated by connecting the IDT to a radio frequency analog signal generator (N5181A-503, Keysight Technologies, USA), a power amplifier (LZY-22+, Mini-Circuits, USA), and a DC power supply (E3634A, Keysight Technologies, USA).
Contact angle measurement
Contact angles were measured by using a contact angle analyzer (Phoenix 300 Plus, SEO Co., Korea). First, to measure the contact angles for different sample volumes, a polydimethylsiloxane (PDMS; Sylgard 184 A/B, Dow Corning, USA) block having a hole with a diameter of 3 mm was used as a mask, and plasma treatment (CUTE, Femto Science, Korea) was performed for a minute at a 100 W intensity on the PDMS film. For each of various volumes of distilled water (DW; Daejung Chemicals & Metals Co., Korea) from 1 μl to 8 μl on the treated PDMS film, a photograph of the sessile droplet was obtained.
Manipulation of particles inside a sessile droplet
PS microparticles with diameters of 6 μm (Fluoro-Max Red, Thermo Fisher Scientific, USA), 10 μm (Polybead® Polystyrene Yellow Dyed Microspheres, Polysciences, USA), and 15 μm (Fluoro-Max Green, Thermo Fisher Scientific, USA) were diluted in DW to 0.2% (v/v), 0.6% (v/v), and 1% (v/v), respectively, and then used to observe the effect of the contact angle of the sessile droplet on the drag force and ARF. SAWs were applied asymmetrically relative to the center of the droplet after adjusting the volume of the droplet so that the desired contact angle was formed on the local surface-treated PDMS film. In the cases of the PS microparticles with diameters of 6 μm and 15 μm, fluorescence images were obtained using an epifluorescence microscope (Axio Observer Z1, Carl Zeiss, Germany), and in the case of the PS microparticles with a diameter of 10 μm, images were obtained using an upright microscope (BX53, Olympus, Japan) equipped with a digital camera (DP72, Olympus, Japan). For color micrographs, to suppress the refraction of light formed along the edge of the sessile droplet at a high contact angle, a thin plastic sheet was added under the substrate, and an external light source was attached to the side instead of using the built-in light source of the microscope.
Cell culture
MCF7 cells (Korean Cell Line Bank, Korea) were cultured in Dulbecco's Modified Eagle's Medium (Lonza, Switzerland) supplemented with 10% (v/v) fetal bovine serum (Gibco, USA) and 1% (v/v) penicillin/streptomycin (Gibco, USA). The cells were cultured in a humidified incubator at 37 °C and 5% CO2. The cells were detached by applying 0.25% trypsin–EDTA (Gibco, USA) and sub-cultured when the cells reached 70–80% confluency.
Manipulation of cells inside the sessile droplet
Surface-treated PDMS films were used as substrates for cell-containing sessile droplets. To visualize the cancer cells, CellTracker™ Red CMTPX (Invitrogen, USA, 5 μM) was used according to the manufacturer's protocol. The stained cancer cells were placed on the surface-treated PDMS film while changing the droplet volume, and the behaviours of the cells subjected to SAWs in droplets with different contact angles were analyzed. Fluorescence images of the cells were obtained using an epifluorescence microscope (Axio Observer Z1, Carl Zeiss, Germany).
PEGylated fibrin gel
The PEGylated fibrin gel with increased density to prevent collapsing when pouring a medium was prepared using a previously described protocol.25 First, filtered fibrinogen from a bovine plasma (Sigma-Aldrich, USA) stock solution, a 3.4 kDa bifunctional poly(ethylene glycol) succinimidyl glutarate (SG-PEG-SG; Nof America Corporation) stock solution, and thrombin from a bovine plasma (Sigma-Aldrich, USA) stock solution were each prepared in phosphate-buffered saline (PBS; Lonza, Switzerland). After that, the samples from the fibrinogen stock solution and SG-PEG-SG stock solution were mixed so that the molar ratio of fibrinogen to SG-PEG-SG was 1
:
5, and then incubated at 37 °C for 10 minutes. In the meantime, the thrombin stock solution was mixed with a cell suspension or PBS. The prepared PEGylated fibrinogen solution and thrombin solution were mixed and then added dropwise onto a surface-treated PDMS substrate. For gelation time measurement, a PS bead solution was mixed with a sample of the thrombin stock solution instead of PBS, and SAWs were applied until the droplet edge started to be polymerized. In other cases, i.e., except for the gelation time measurement, the N-hydroxysuccinimide (NHS) esters remaining after the reaction and the unreacted residues were washed away by replacing the polymerized hydrogel with a fresh medium or PBS at 30 minutes and 2.5 hours after the start of the experiment.
Scanning electron microscopy
Hydrogel samples for scanning electron microscopy (SEM) studies were prepared as previously described.26,27 Briefly, the hydrogels were rinsed 3 times each for 15 minutes with 50 mM sodium cacodylate buffer solution (Sigma-Aldrich, USA). The samples were then each fixed overnight with a 2% glutaraldehyde solution (Sigma-Aldrich, USA). The fixed samples were dehydrated with 30, 50, 70, 90, and 95% ethanol for 15 minutes each and 3 times with 100% ethanol for 15 minutes. The dehydrated samples were air-dried using hexamethyldisilazane (Sigma-Aldrich, USA) and then coated with osmium and observed using a field emission scanning electron microscope (SU5000, Hitachi, Japan).
Viability
Hoechst 33342 (Thermo Fisher Scientific, USA, 1 μg ml−1) and ethidium homodimer-1 (Thermo Fisher Scientific, USA, 4 μM), compounds with high affinity for nucleic acids, were used to confirm the cell viability and hence to examine the biocompatibility of the SAWs. The cells were stained as described in the manufacturer's protocol. The stained cells were imaged using a high-resolution confocal microscope (LSM 880, Carl Zeiss, Germany). Since Hoechst 33342 is cell-permeable and ethidium homodimer-1 is cell-impermeable, viability (%) was calculated as: | 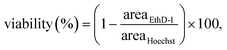 | (1) |
where areaEthD-1 is the nuclear area stained by ethidium homodimer-1 and areaHoechst is the nuclear area stained by Hoechst 33342.
Immunofluorescence staining
The cells were first treated in 4% paraformaldehyde (Biosesang, Korea) for 20 minutes at room temperature. Then, they were treated with 0.5% Triton™ X-100 solution (Sigma-Aldrich, USA) for 30 minutes, followed by washing twice with PBS. These cells were then treated for one hour with a CAS-Block™ histochemical reagent (Thermo Fisher Scientific, USA), followed by treating overnight with a 1
:
100 dilution of recombinant anti-E-cadherin antibody (Abcam, UK), an intercellular junction marker. After removing this primary antibody solution and washing the cells 5 times with PBS, the cells were treated overnight with a solution consisting of anti-rabbit Alexa Fluor 488 secondary antibody (Invitrogen, USA) diluted in 1
:
200, 4′,6-diamidino-2-phenylindole (Sigma-Aldrich, USA) diluted in 1
:
1000, and rhodamine phalloidin (Invitrogen, USA) diluted in 1
:
400 in PBS with 0.1% bovine serum albumin (BSA; Sigma-Aldrich, USA). Lastly, the cells were washed 3 times with PBS together with 0.1% BSA, and then imaged using a confocal microscope (LSM 880, Carl Zeiss, Germany).
Drug screening
The anticancer drug tirapazamine (TPZ; Toronto Research Chemicals, Canada) was purchased and dissolved in DMSO for producing a stock solution. The samples of the stock solution were diluted to 50 μM, 100 μM, and 500 μM in a culture medium. The samples of the cells were treated for 24 hours with each medium diluted with the drug, and then stained with Hoechst 33342 and ethidium homodimer-1. The viability under each condition was calculated using eqn (1).
Data analysis
All the fluorescence micrographs were analyzed using Fiji (https://imagej.net/Fiji). For statistical comparisons, MATLAB (MathWorks, USA) was used. Two different groups were compared with an unpaired two-sample t-test, and a p-value of less than 0.05 was considered to be statistically significant.
Results and discussion
A SAW platform, shown in Fig. 1(a), was used to manipulate microparticles inside a sessile droplet, and this manipulation was accomplished by adjusting the contact angle. When a PDMS film is surface-treated, the contact angle of the droplet can be increased up to the typical contact angle on PDMS (∼110°)28 while maintaining the same surface area (Fig. 2(b)). The SAWs generated by the IDT propagated along the LiNbO3 substrate, penetrated the PDMS film, and were asymmetrically introduced into the sessile droplet (Fig. 1(a)). The aperture of the IDT (1.2 mm) is smaller than the radius of the sessile droplet (1.5 mm) where the sessile droplet can be located asymmetrically with respect to the IDT. Due to the viscoelastic properties of PDMS, a wave intensity attenuation of ∼0.933 dB occurred as a result of the waves penetrating the PDMS film29 (mean thickness of 69 ± 2 μm from three independent films). By Snell's law, the refraction angle (θR2) of the acoustic waves transmitted to the sessile droplet through the PDMS film was calculated as: | 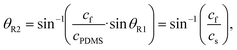 | (2) |
where cf, cPDMS, and cs are the speeds of sound in water (1485 m s−1), PDMS (1119.1 m s−1),30 and LiNbO3 (3965 m s−1), respectively12 and θR1 is the refraction angle from LiNbO3 to PDMS. The Rayleigh waves were transformed into longitudinal waves in the PDMS film and further propagated to the sessile droplet.31 These propagated waves then reflected within the sessile droplet,32 and the attenuation length (xf) can be written as:6 |  | (3) |
where ρf is the density of the fluid, μ is the dynamic viscosity of the fluid, and f is the wave frequency. The long xf (∼165 mm) due to the relatively low frequency used made the transmitted waves reflective and form longitudinal standing waves.6 The acoustic force field depends greatly on the actuation frequency, droplet volume and droplet exposure to SAWs. The attenuation length (xf) as it interacts with a droplet plays an important role in driving the acoustic forces. The standing wave-induced ARF along the edge of the droplet acted more dominantly than did the ASF-induced drag force. At the same time, the offset between the center of the sessile droplet and the center of the Gaussian distribution of the acoustic waves due to the above-described asymmetric arrangement resulted in a decrease in the azimuthal flow velocity at the center of the droplet (Fig. 1(c)). The κ-factor (κ) to consider the ARF by travelling waves may be derived as: |  | (4) |
where dp is the particle diameter. Due to the frequency used (19.32 MHz) and the diameters of the PS microparticles (6, 10, 15 μm), the travelling wave-induced ARF was considered to be negligible compared to the other two forces (ASF-induced drag force and standing wave-induced ARF) for κ < 1.6 The influence of the volume of the sessile droplet on the behaviours of the microparticles was examined by changing the contact angle of the droplet. Due to the influence of the unbalanced force distribution, most of the microparticles congregated in the center of the sessile droplet as a result of the standing wave-induced ARF and ASF-induced drag force at higher contact angles and were trapped in the pressure nodes along the edge of the droplet and congregated in the center of the droplet as a result of the ASF-induced drag force and enhanced standing wave-induced ARF along the edge at lower contact angles (Fig. 1(b)). At higher contact angles, more microparticles, as well as cells, can be collected in the center of the sessile droplet as a result of the ASF-induced drag force, as shown in Fig. 1(d).
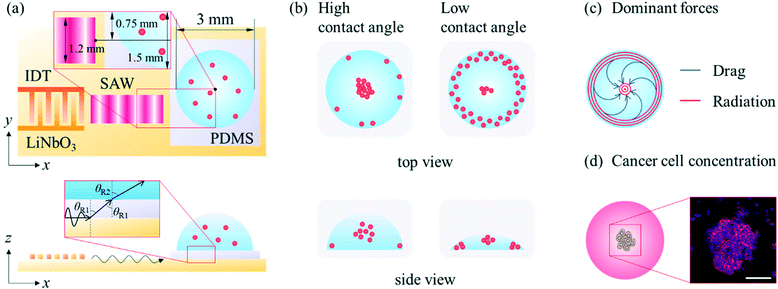 |
| Fig. 1 (a) Schematic of the experimental setup. (b) Particle behaviours for high and low contact angles. (c) Dominant forces within the sessile droplet. (d) Concentration of the cancer cells to mimic the primary tumour site (maximum intensity projection of the fluorescence image of nuclei (blue) and F-actin (red), scale bar: 100 μm). | |
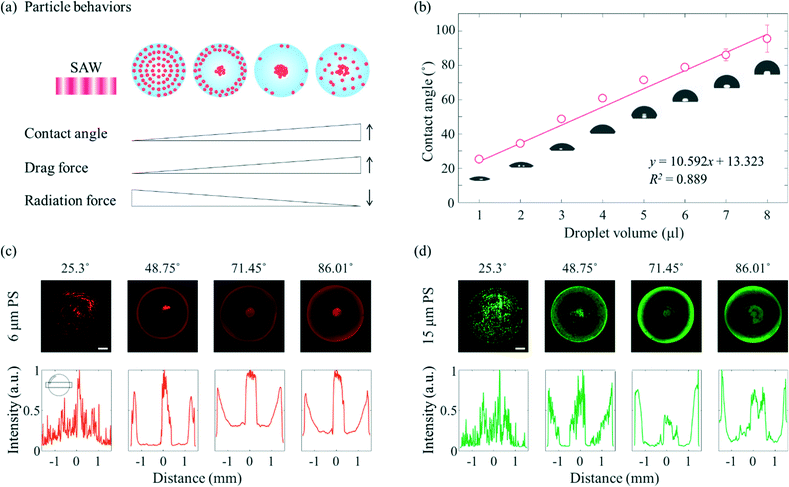 |
| Fig. 2 Particle behaviours for different contact angles. (a) Schematic of the behaviours. (b) Contact angle vs. droplet volume (red circle: mean, error bar: standard deviation, n = 3, inset: representative images, d = 3 mm). Fluorescence images of the (c) 6 μm-diameter and (d) 15 μm-diameter PS microparticles at the same frequency, voltage, and droplet diameter (top row) and normalized mean column fluorescence intensities across the sessile droplet (bottom row) (scale bar: 500 μm, the inset in (c) shows the region of interest). | |
A schematic diagram showing in more detail the dependence of the particle behaviours on the contact angle is shown in Fig. 2(a). Due to the enhanced liquid–air and liquid–substrate reflections at low contact angles, the standing wave-induced ARF here was predominated. A relatively strong ARF compared to the ASF-induced drag force enabled particles to be trapped to form a concentric pattern. With an increasing contact angle, the microparticles started to escape from the pressure nodes located between the center and edge of the droplet due to the relatively weakened reflection at the edge of the sessile droplet and enhanced ASF-induced drag force, and then became concentrated at the center of the droplet. Due to the presence of distinct drag force-dominated and ARF-dominated regions in the sessile droplet, the microparticles became concentrated or formed an annular ring at the same time in this contact angle range. Subsequently, with further increases in the contact angle, most of the microparticles escaped from the pressure nodes as a result of the ASF-induced drag force and became concentrated at the center of the sessile droplet. Finally, the 3D flow due to the high contact angle made the microparticles mixed throughout the droplet rather than more concentrated at the center. In Fig. 2(b), the contact angle increased almost linearly (R2 = 0.889) as the volume of the DW was increased from 1 μl to 8 μl on the surface-treated PDMS film with a constant diameter of 3 mm. For the volumes of the sessile droplet between 1 μl to 8 μl in steps of 1 μl, the average contact angles were 25.3°, 34.53°, 48.75°, 60.87°, 71.45°, 78.8°, 86.01°, and 95.53°, respectively. It would be difficult to measure the contact angle over the intrinsic contact angle on PDMS.
To verify the dependence of the behaviours of the particles on the contact angle when applying SAWs of the same frequency, fluorescent PS microparticles of two different diameters (6 μm, red fluorescence and 15 μm, green fluorescence) were used, as shown in Fig. 2(c) and (d). For both microparticles, when the average contact angle was 25.3°, the standing wave-induced ARF predominated, whereas when the average contact angle was 86.01°, the ASF-induced drag force was the predominant force, and the microparticles became concentrated at the center of the droplet. When the contact angle of the sessile droplet with the 6 μm microparticles was 25.3°, the microparticles were rotated by the ASF weakly generated in the middle of the droplet, and some microparticles became trapped in the SAW-applied side (the upper side of the sessile droplet in the fluorescence images). On the other hand, i.e., unlike for the 6 μm microparticles, most of the 15 μm microparticles became trapped in the pressure nodes, especially the SAW-applied side of the sessile droplet (Fig. S2†). When the contact angle was 48.75°, more microparticles began to be affected by the drag force than when the contact angle was 25.3°. As a result, the particles concentrated at the center of the droplet. Along the edge of the sessile droplet, the 15 μm microparticles became trapped by the standing waves. The amount of trapped microparticles is greater than that of 6 μm in diameter, and the intervals resulting from the standing waves was clearly visible. As the contact angle was increased further, to 71.45° and 86.01°, both the 6 μm and 15 μm microparticles became concentrated at the center of the sessile droplet. For each of these two contact angles, a signal from the fluorescent microparticles was detected along the edge of the droplet. The fluorescence signals were not discrete, but hazy and continuous, which may be attributed to the refraction of light at the edge of the sessile droplet with a higher contact angle. Furthermore, an external light source was located on the side of the sessile droplet (Fig. S3†). In particular, when the contact angle was 86.01° (fourth column), the region between the center and edge of the droplet appeared slightly blurred compared to the appearance of this region when the contact angle was 71.45° (third column). The increase in the contact angle may have enhanced the 3D flow and interfered with the azimuthal movement of the microparticles toward the center of the drop. Note that the evaporation effect during the experiment (60 s) is insignificant under the present conditions (20 MHz and 18 mV).
As approximately 70% of cell mass is composed of water,33 the acoustic impedance of cells is relatively low compared to that of PS microparticles.34 While the effect of the travelling wave-induced ARF on the PS microparticles is quite large due to the difference between the acoustic impedance, the effect of the ARF on cells is reduced due to similar acoustic impedances of cells and water.35 To determine the effect of SAWs on cells in various sessile droplets with different contact angles, MCF7 breast cancer cells were suspended in the medium instead of PS particles (Fig. 3). When the contact angle was 25.3°, most of the cancer cells became trapped in the pressure nodes formed by overlapping standing waves. The cancer cells tended to escape more easily than did the PS microparticles as the contact angle was increased. Most of the cells were found to be concentrated in the center of the droplet having a contact angle of 60.87° compared to 51.17°, where most cells in the center were trapped at the edge of the droplet. That the cancer cells were apparently more affected by drag force than PS microparticles. This was presumed to be the reduced effect of ARF by the low acoustic impedance difference. The effective diameter of the cells (Fig. S4†) being larger than that of the PS microparticles used also increased the effect of the drag force. At a contact angle of 75.13°, most of the cells were distributed randomly throughout the sessile droplet, due to the ASF-induced drag force that formed in three dimensions inside the sessile droplet.
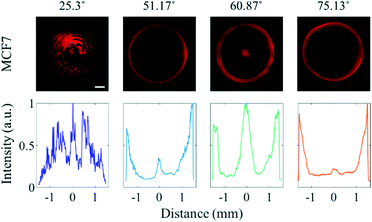 |
| Fig. 3 Fluorescence images of the MCF7 cells in various sessile droplets with four different contact angles at the same frequency, voltage, and droplet diameter (top row), and normalized mean column fluorescence intensity across each sessile droplet (bottom row) (scale bar: 500 μm). | |
Various hydrogels have been used for applications in in vitro microphysiological systems.36 Naturally derived fibrin gel, which is a component of blood clot formed as a result of the enzymatic reaction of thrombin on fibrinogen, is one of the hydrogels widely used to mimic the ECM of the cellular microenvironment.37,38 Combining fibrinogen and thrombin solutions has been shown to result in the gelation of fibrin through knob–hole interactions using knobs ‘A’ of the fibrin monomer. The high affinity of holes ‘a’ for these knobs leads to quick gelation of fibrin, specifically within seconds, which is not a long enough time to generate ASF.39 In order to afford a sufficiently long gelation time for ASF-induced effects to be realized, SG-PEG-SG, a homobifunctional crosslinking PEG reagent, was employed.25 Attachment of an NHS ester reagent to both ends of SG-PEG-SG has been shown to slow down the polymerization of the fibrin monomer by covalently bonding with the amine in fibrinogen. Consequently, the PEGylated fibrin gel formed a fiber thicker than the fibrin gel formed by covalent bonds (Fig. 4(a)), and displayed a long enough gelation time (increased from 19.2 ± 7.1 s to 39.8 ± 11 s, Fig. 4(b)) to enable the use of the ASF-induced drag force. SAWs can be used to manipulate target materials (PS particles, cancer cells). Since the negative effects of the radiation force and drag force on the cells cannot be excluded, the biocompatibility of SAWs was tested by conducting a viability comparison (Fig. 4(c) and (d)). Here, the MCF7 cells were found to have similar viabilities regardless of the SAW status, specifically 95.5 ± 3.2% for the SAWs off, and 96.4 ± 1.2% for the SAWs on. The somewhat less than perfect viabilities under both of these conditions may have been due to the effect of manipulating cells during the experiment. We found that the cell viability is negligible at the present low 18 mV.
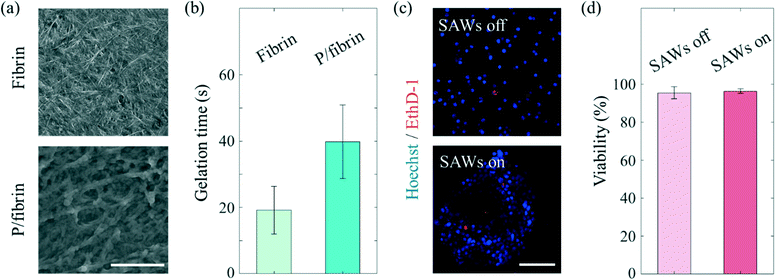 |
| Fig. 4 (a) SEM images of the fibrin gel and PEGylated fibrin gel (scale bar: 10 μm, P/fibrin: PEGylated fibrin). (b) Gelation times for 8 mg ml−1 fibrin and PEGylated fibrin gel (error bars represent standard deviation, n = 5). (c) Representative maximum intensity projection fluorescence images of the MCF7 cells (scale bar: 100 μm). (d) Viabilities of the MCF7 cells with and without SAWs (error bars represent standard deviation, n = 4). | |
To mimic the tumour microenvironment, the contact angle was set to 60.87° for increasing the concentration of the cancer cells as shown in Fig. 3. In addition, the PEGylated fibrin gel was used as a 3D scaffold to maintain the aggregation of the cancer cells even after SAWs were applied. As shown in Fig. 5(a), the effective diameter
increased in proportion to the increase in the cell density (172.4 ± 29.7 μm, 254.6 ± 16.6 μm and 305.3 ± 44.5 μm for 1 × 106 cells per ml, 3 × 106 cells per ml, and 5 × 106 cells per ml, respectively). Similarly, as shown in Fig. 5(b), the circularity
of the cell aggregation increased as the cell density increased, with values of 0.65 ± 0.02, 0.75 ± 0.06 and 0.89 ± 0.09 for 1 × 106 cells per ml, 3 × 106 cells per ml, and 5 × 106 cells per ml, respectively. Although the cancer cells were concentrated even at a low cell density, the circularity was low, due to the small number of cancer cells. As shown in Fig. 5(c) and S5,† fluorescence staining of cell aggregates was carried out to evaluate the 3D tumour microenvironment. Here, E-cadherin, which is one of the epithelial cell adhesion proteins, was confirmed to be expressed at high levels in the aggregated cancer cells.40 Since 3D aggregated culture models make cancer cells express E-cadherin at higher levels compared to 2D monolayer culture models, it was expected that the cancer cells patterned by SAWs provided more information about the characteristics of the tumour microenvironment than those from dispersed culture models.41,42
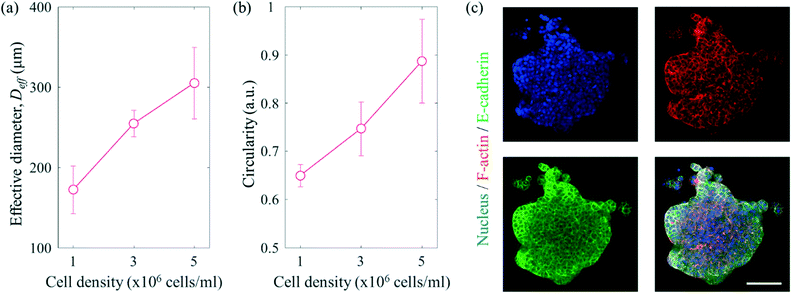 |
| Fig. 5 (a) Effective diameters of the cancer cell aggregations with different cell densities (error bars represent standard deviation, n = 3). (b) Circularity values of the cell aggregations with different cell densities (error bars represent standard deviation, n = 3). (c) Maximum intensity projection fluorescence images of the MCF7 cells (scale bar: 100 μm). | |
In contrast to conventional 2D in vitro models, aggregated cancer cells that form a 3D microenvironment permit complex interactions to form between the surrounding cells and the ECM. Such aggregation of cells has a variety of consequences including enhanced or reduced levels of gene expression, elongated or cuboidal morphological changes, and sensitization.43 The tumour microenvironment shows a relatively low partial pressure of oxygen.44 Hypoxic conditions mainly appear in tumour microenvironments; especially in the core of the primary tumour in which many cancer cells are concentrated. There are various anticancer drugs that efficiently target the cancer cells in the core.45 TPZ is one of the anticancer drugs found to target hypoxic environments. Since TPZ is activated under hypoxic conditions, its anticancer efficacy increases in hypoxic environments.46 Here, different samples of cancer cells were treated with TPZ at, respectively, three different concentrations (50 μM, 100 μM and, 500 μM) for 24 hours (Fig. 6). At 50 μM, the viability levels of the cells with and without the application of SAWs were 84.8 ± 15.2% and 90.8 ± 6.2%, respectively (Fig. 6(b)); that is the viability only slightly decreased when SAWs were applied. As the concentration of TPZ was increased to 100 μM, this difference in viability gradually increased (92.3 ± 3.6% without SAWs and 83.6 ± 22.8% with SAWs). At a concentration of 500 μM, the difference in viability due to SAWs was apparently even greater (76.3 ± 14.6% without SAWs and 60.1 ± 14.4% with SAWs). As mentioned above, the aggregated cancer cells presumably did not obtain sufficient oxygen, i.e., they were under relatively hypoxic conditions compared to the conditions for the dispersed cells. The degree of activation of TPZ was thus, as expected, relatively high under our presumably hypoxic conditions. Our current study moreover was able to take advantage of the ability, using SAWs, to more rapidly manipulate cancer cells than can be done using conventional culture methods. In addition, since the non-contact manipulation property of acoustic waves is biocompatible and enables a structure-free platform configuration, it has the potential to be used for various biological applications. Although we manipulated a single cancer cell line, the different drug response from conventional system was expected to establish drug screening platform for in vitro to in vivo extrapolation.
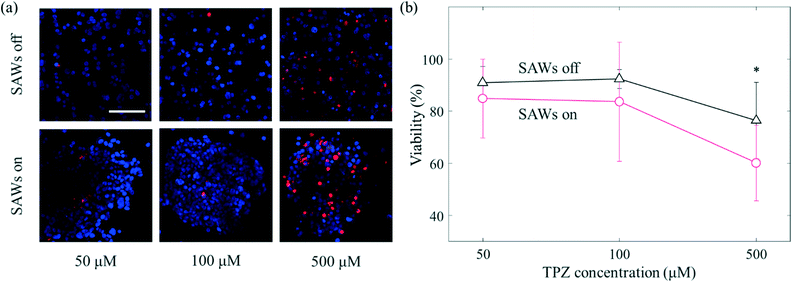 |
| Fig. 6 Concentrated cancer cells influence the efficacy of TPZ, which targets hypoxic tumours. (a) Maximum intensity projection fluorescence images of MCF7 (blue: Hoechst 33342, red: ethidium homodimer-1, scale bar: 100 μm). (b) Viability versus TPZ concentration (13 devices under each condition from 10 independent experiments, error bars: standard deviation, asterisk: p < 0.05). | |
Conclusions
We examined the behaviours of polystyrene microparticles and cancer cells by applying travelling SAWs asymmetrically in a sessile droplet. The Rayleigh waves formed using an interdigital transducer were made to propagate along the surface of a LiNbO3 substrate. ASF-induced drag force and standing wave-induced ARF were utilized in various combinations by changing the contact angle. The manipulation of microparticles and cancer cells in sessile droplets was performed without the use of microchannels. By patterning cancer cells under an optimal contact angle condition and exploiting a modified hydrogel as a scaffold, we deployed the present platform as an in vitro drug screening model. The local cellular distribution was manipulated, and done so rapidly, by using travelling SAWs. SG-PEG-SG covalently bonds with the amines in fibrinogen and hence slows down the otherwise rapid polymerization of fibrin monomers. The PEGylated fibrin gel was observed to form a fiber thicker than the fibrin gel. MCF7 cells were found to have a negligible effect on the viability levels of cells regardless of the SAW conditions. The effective diameter of the aggregated cancer cells was observed to increase in proportion to the increase in the cell density. The aggregated cancer cells patterned by SAWs exhibited more characteristics in the tumour microenvironment than those from dispersed culture models. We found E-cadherin being expressed in the aggregated cancer cells, and attributed this to the aggregated form of the cancer cells. The efficacy of an anticancer drug against the corresponding cancer cell aggregation was found to differ from its efficacy when using a conventional dispersed model. This discrepancy between in vitro models indicates that testing parameters including particle dimensions and cellular distribution are important for mimicking in vivo experimental models. Further applications can be implemented by aligning cells to form functional tissues, patterning various cell types for achieving human disease modeling, and stimulating cells for enhancing the secretion of specific cytokines.
Author contributions
H. J. S., J. S. J., and J. P. supervised the research. H. N. conceived the research and designed and performed the experiments. H. N. analyzed the experimental results. H. N., H. J. S., and J. S. J. wrote the manuscript. All the authors reviewed the manuscript.
Conflicts of interest
There are no conflicts to declare.
Acknowledgements
This work was supported by the National Research Foundation of Korea (NRF) grant funded by the Korea government (MSIT) (No. 2020R1A2C2008106, 2020R1A2C110047111 and 2020R1A5A8018367) and the Korea Basic Science Institute (DC202102).
References
- K. Duval, H. Grover, L.-H. Han, Y. Mou, A. F. Pegoraro, J. Fredberg and Z. Chen, Physiology, 2017, 32, 266–277 CrossRef CAS PubMed.
- H. Yun, K. Kim and W. G. Lee, Biofabrication, 2013, 5, 022001 CrossRef.
- G. Luka, A. Ahmadi, H. Najjaran, E. Alocilja, M. DeRosa, K. Wolthers, A. Malki, H. Aziz, A. Althani and M. Hoorfar, Sensors, 2015, 15, 30011–30031 CrossRef CAS PubMed.
- R. J. Shilton, M. Travagliati, F. Beltram and M. Cecchini, Adv. Mater., 2014, 26, 4941–4946 CrossRef CAS.
-
(a) H. Li, J. R. Friend and L. Y. Yeo, Biomed. Microdevices, 2007, 9, 647–656 CrossRef.
- G. Destgeer, H. Cho, B. H. Ha, J. H. Jung, J. Park and H. J. Sung, Lab Chip, 2016, 16, 660–667 RSC.
- G. Destgeer, J. H. Jung, J. Park, H. Ahmed and H. J. Sung, Anal. Chem., 2017, 89, 736–744 CrossRef CAS.
- J. Reboud, Y. Bourquin, R. Wilson, G. S. Pall, M. Jiwaji, A. R. Pitt, A. Graham, A. P. Waters and J. M. Cooper, Proc. Natl. Acad. Sci. U. S. A., 2012, 109, 15162–15167 CrossRef CAS.
- J. Nam, W. S. Jang, J. Kim, H. Lee and C. S. Lim, Biosens. Bioelectron., 2019, 142, 111496 CrossRef CAS PubMed.
- A. Salehi-Reyhani, F. Gesellchen, D. Mampallil, R. Wilson, J. Reboud, O. Ces, K. R. Willison, J. M. Cooper and D. R. Klug, Anal. Chem., 2015, 87, 2161–2169 CrossRef CAS PubMed.
- L. Alhasan, A. Qi, A. Al-Abboodi, A. Rezk, P. P. Chan, C. Iliescu and L. Y. Yeo, ACS Biomater. Sci. Eng., 2016, 2, 1013–1022 CrossRef CAS PubMed.
- W. Connacher, N. Zhang, A. Huang, J. Mei, S. Zhang, T. Gopesh and J. Friend, Lab Chip, 2018, 18, 1952–1996 RSC.
- S. J. Kim, K. H. Kang, J.-G. Lee, I. S. Kang and B. J. Yoon, Anal. Chem., 2006, 78, 5192–5197 CrossRef CAS PubMed.
- J.-Y. Jung and H.-Y. Kwak, Anal. Chem., 2007, 79, 5087–5092 CrossRef CAS PubMed.
- D. Mampallil, D. Tiwari, D. van den Ende and F. Mugele, Biomicrofluidics, 2013, 7, 044102 CrossRef.
- J. R. Trantum, D. W. Wright and F. R. Haselton, Langmuir, 2012, 28, 2187–2193 CrossRef CAS PubMed.
- J. Park, G. Destgeer, H. Kim, Y. Cho and H. J. Sung, Lab Chip, 2018, 18, 2936–2945 RSC.
- M. Wiklund, R. Green and M. Ohlin, Lab Chip, 2012, 12, 2438–2451 RSC.
- D. Mampallil, J. Reboud, R. Wilson, D. Wylie, D. R. Klug and J. M. Cooper, Soft Matter, 2015, 11, 7207–7213 RSC.
- A. Akther, S. Marqus, A. R. Rezk and L. Y. Yeo, Anal. Chem., 2020, 92, 10024–10032 CrossRef CAS PubMed.
- J. P. Lata, F. Guo, J. Guo, P. H. Huang, J. Yang and T. J. Huang, Adv. Mater., 2016, 28, 8632–8638 CrossRef CAS PubMed.
- B. Chen, Y. Wu, Z. Ao, H. Cai, A. Nunez, Y. Liu, J. Foley, K. Nephew, X. Lu and F. Guo, Lab Chip, 2019, 19, 1755–1763 RSC.
- X. Hu, S. Zhao, Z. Luo, Y. F. Zuo, F. Wang, J. Zhu, L. Chen, D. Yang, Y. Zheng and Y. Zheng, Lab Chip, 2020, 20, 2228–2236 RSC.
- M. Vinci, S. Gowan, F. Boxall, L. Patterson, M. Zimmermann, C. Lomas, M. Mendiola, D. Hardisson and S. A. Eccles, BMC Biol., 2012, 10, 1–21 CrossRef.
- O. M. Benavides, J. P. Quinn, S. Pok, J. Petsche Connell, R. Ruano and J. G. Jacot, Tissue Eng., Part A, 2015, 21, 1185–1194 CrossRef CAS.
- N. Badiei, A. Sowedan, D. Curtis, M. Brown, M. Lawrence, A. Campbell, A. Sabra, P. Evans, J. Weisel and I. Chernysh, Clin. Hemorheol. Microcirc., 2015, 60, 451–464 CAS.
- M. Tomaiuolo, C. N. Matzko, I. Poventud-Fuentes, J. W. Weisel, L. F. Brass and T. J. Stalker, Proc. Natl. Acad. Sci. U. S. A., 2019, 116, 2243–2252 CrossRef CAS PubMed.
- B. Ruben, M. Elisa, L. Leandro, M. Victor, G. Gloria, S. Marina, S. K. Mian, R. Pandiyan and L. Nadhira, Micro Nano Lett., 2017, 12, 754–757 CrossRef CAS.
- D. Rabaud, P. Thibault, J.-P. Raven, O. Hugon, E. Lacot and P. Marmottant, Phys. Fluids, 2011, 23, 042003 CrossRef.
- J. K. Tsou, J. Liu, A. I. Barakat and M. F. Insana, Ultrasound Med. Biol., 2008, 34, 963–972 CrossRef PubMed.
- J. Park, B. H. Ha, G. Destgeer, J. H. Jung and H. J. Sung, RSC Adv., 2016, 6, 33937–33944 RSC.
- P. Brunet, M. Baudoin, O. B. Matar and F. Zoueshtiagh, Phys. Rev. E: Stat., Nonlinear, Soft Matter Phys., 2010, 81, 036315 CrossRef CAS PubMed.
-
K. Roberts, B. Alberts, A. Johnson, P. Walter and T. Hunt, Molecular Biology of the Cell, New York: Garland Science, 2002, vol. 32 Search PubMed.
- G. Destgeer, J. H. Jung, J. Park, H. Ahmed, K. Park, R. Ahmad and H. J. Sung, RSC Adv., 2017, 7, 22524–22530 RSC.
- Z. Ma, Y. Zhou, D. J. Collins and Y. Ai, Lab Chip, 2017, 17, 3176–3185 RSC.
- S. R. Caliari and J. A. Burdick, Nat. Methods, 2016, 13, 405–414 CrossRef CAS.
- J. S. Jeon, S. Bersini, M. Gilardi, G. Dubini, J. L. Charest, M. Moretti and R. D. Kamm, Proc. Natl. Acad. Sci. U. S. A., 2015, 112, 214–219 CrossRef CAS.
- S. Kim, J. Park, J. Kim and J. S. Jeon, ACS Biomater. Sci. Eng., 2021, 7, 1230–1241 CrossRef CAS PubMed.
- J. W. Weisel and R. I. Litvinov, Blood, 2013, 121, 1712–1719 CrossRef CAS PubMed.
- D. Wirtz, K. Konstantopoulos and P. C. Searson, Nat. Rev. Cancer, 2011, 11, 512–522 CrossRef CAS PubMed.
- T.-Y. Na, L. Schecterson, A. M. Mendonsa and B. M. Gumbiner, Proc. Natl. Acad. Sci. U. S. A., 2020, 117, 5931–5937 CrossRef CAS.
- L. Zhao, J. Xiu, Y. Liu, T. Zhang, W. Pan, X. Zheng and X. Zhang, Sci. Rep., 2019, 9, 1–14 Search PubMed.
- M. Sacchi, R. Bansal and J. Rouwkema, Trends Biotechnol., 2020, 38, 623–636 CrossRef CAS PubMed.
- E. B. Rankin and A. J. Giaccia, Science, 2016, 352, 175–180 CrossRef CAS.
- X. Lu and Y. Kang, Clin. Cancer Res., 2010, 16, 5928–5935 CrossRef CAS PubMed.
- H. Nam, K. Funamoto and J. S. Jeon, Biomicrofluidics, 2020, 14, 044107 CrossRef CAS PubMed.
Footnote |
† Electronic supplementary information (ESI) available. See DOI: 10.1039/d1lc00801c |
|
This journal is © The Royal Society of Chemistry 2022 |