DOI:
10.1039/D2GC00664B
(Communication)
Green Chem., 2022,
24, 3629-3633
Sustainable and fast synthesis of functionalized quinoxalines promoted by natural deep eutectic solvents (NADESs)†
Received
18th February 2022
, Accepted 30th March 2022
First published on 31st March 2022
Abstract
Functionalized quinoxalines, embedding acid sensitive protecting groups, can be prepared with a fast (5 min) and high yielding (>90%) protocol using a choline chloride/water NADES at room temperature without any additional activation. The products obtained after solvent extraction do not require any further purification. The NADES used can be recycled up to six times maintaining the same efficiency level.
Introduction
The quinoxaline unit is embedded in a notable number of compounds showing manifold practical applications. In the field of materials chemistry, quinoxaline derivatives show liquid crystal properties,1 are known as corrosion inhibitors,2 and find wide use in photovoltaic devices.3 On the other hand, these heterocyclic systems are also currently employed as anticancer agents,4 for their recognized antiviral and antitubercular activities,5 as well as for their many other important biomedical applications (Fig. 1).6 Over the years, remarkable interest in these quinoxaline-containing compounds has resulted in the development of a high number of protocols, which often feature a limited level of sustainability, such as those using transition metal catalyzed reactions.7 A survey of the existing procedures available for the synthesis of quinoxalines reveals that the condensation of 1,2-diamino compounds with 1,2-dicarbonyl derivatives represents the most straightforward approach to obtaining these target products.8 This simple reaction can occur even under uncatalyzed conditions in water,9 or ethanol,10 but is limited to the utilization of poorly functionalized substrates or requires harsh conditions (Scheme 1). Better results can be generally obtained by catalyzed or promoted processes working under acidic homogeneous or heterogeneous conditions. Evaluating the nature of various acids which can be possibly used for this reaction, acetic acid appears as the most advantageous one mainly considering its sustainability features.11 However, several processes entailing the use of acetic acid often require relatively high temperatures or prolonged reaction times, which considerably curtail the sustainability features of the corresponding synthetic protocol. A protic ionic liquid obtained mixing DABCO, acetic acid and water (1
:
1
:
3) has been recently used for the synthesis of quinoxalines showing some advantages over traditional procedures but a relatively high temperature (80 °C, 20–40 min) is still required for a proper reaction.12 In an attempt to minimize these drawbacks, we turned our attention to the use of deep eutectic solvents (DESs), which have been recognized as formidable green media in several processes because of their low toxicity and high biodegradability.13
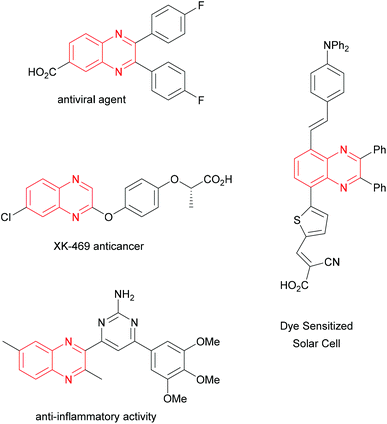 |
| Fig. 1 Bioactive compounds featuring the quinoxaline ring. | |
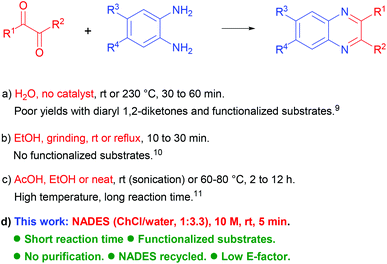 |
| Scheme 1 Synthetic protocols for the preparation of quinoxalines. | |
A DES is defined as a mixture of compounds showing a significant depression of the melting point compared to those of the single pure components. The eutectic point represents the composition ratio at which the minimum value of the melting point is recorded. This DES composition is normally employed for synthetic and practical purposes. Additionally, DESs feature a low viscosity, a high thermal stability and a low vapor pressure, which make them ideal candidates to replace volatile organic solvents (VOCs) in several processes. Particularly, the peculiar activating properties of DESs have been demonstrated when used as selective extracting solvents from lignin and other biomass matrixes.14 Well documented synthetic applications of DESs can be highlighted in multicomponent and asymmetric catalyzed processes,15 metal and biocatalyzed reactions,16 and polymer and materials science.17 According to their composition, the chemical features of DESs can be suitably tuned to provide basic, neutral or acidic environments. From a sustainable standpoint, natural deep eutectic solvents (NADESs) are of particular interest since their components are polar compounds featuring high biodegradability and biocompatibility.18 These NADESs are usually binary mixtures of hydrogen-bond acceptors (HBA) and hydrogen-bond donors (HBD) and the more popular HBA is certainly choline chloride (ChCl), which also finds widespread use as a nutritional additive.19 Acetic acid, urea, lactic acid and water are employed as HBD together with ChCl in the preparation of various NADESs that feature different properties. Water represents a special case of HBD components in NADESs since it has been recently demonstrated that ChCl/water mixtures in the range of 1
:
3–4 ratios behave as a real NADES rather than a concentrated water solution of the ammonium salt.20 As a matter of fact ChCl/water in a ratio of 1
:
3.33 remains liquid even at −80 °C.21 In this paper we report for the first time a fast, clean and efficient synthesis of functionalized quinoxalines by condensation of 1,2-dicarbonyl derivatives with 1,2-diamino compounds promoted just by a ChCl/water NADES in 5 min at room temperature.
Results and discussion
The stability of ChCl-based DESs appears to be due to the extended network of hydrogen bonding and van der Waals interactions involving ionic atoms and also ammonium methyl groups. In most NADESs, the activation of reactants toward the condensation is therefore supposed to conveniently occur by their embedding in this network and lowering the energy of the transition state.22 The first trial using ChCl/AcOH on the model reactants 1a and 2a gave the expected quinoxaline 3a after 5 min stirring in high yield (Table 1, entry 1). Comparable results were obtained using the combination of ChCl/lactic acid but the time required for a complete conversion was notably longer (Table 1, entry 2). A related behavior was observed employing the very popular DES ChCl/urea, which gave a complete conversion of the reactants after 1 h but required heating at 45 °C (Table 1, entry 3). Interestingly, the outstanding results achieved with ChCl/AcOH were maintained using ChCl/water, which introduced a notable improvement in the sustainability features of the protocol (Table 1, entry 4). In this context, it should be observed that ammonium halides in methanol or acetonitrile solutions have been used in the synthesis of quinoxalines but under heating or prolonged reaction times.23 The crucial activating effect exerted by a DES was highlighted by running the reaction under neat conditions without any further activation. In this instance, compound 3a was obtained in only 50% yield together with a large amount of unreacted materials (Table 1, entry 5). In order to reduce the amount of NADES used in this process, the concentration was progressively increased by tenfold (10 M) without any significant reduction in the chemical yield (Table 1, entries 6 and 7). A further increase in the concentration up to 15 M caused a notable increase in the reaction time, although the yield of the obtained product was still excellent (Table 1, entry 8).
Table 1 Optimization studiesa
The optimized reaction conditions were then employed to evaluate the substrate scope for the preparation of various functionalized quinoxalines 3 (Table 2). Unsymmetrical 1,2-diketones bearing an aryl framework and an alkyl framework were initially chosen for the condensation with symmetrical 1,2-diaminobenzenes leading to quinoxalines 3a-f in excellent yields.
Table 2 Substrate scope for the synthesis of quinoxalines 3
a
Reaction conditions: diketone 1 (0.5 mmol) and diamine 2 (0.5 mmol) stirred in ChCl/water (1 : 3.3), 10 M for 5 min.
Reaction carried out on 5 mmol of reactants.
|
|
The same pattern has been obviously observed using symmetrical 1,2-dicarbonyls with unsymmetrical 1,2-diaminobenzenes for the preparation of compounds 3g and m. As expected, the condensation of a couple of unsymmetrical reactants was devoid of any regioselectivity leading to an equimolar mixture of products 3k and 3k′ although in excellent overall yield. The reaction of benzil with 1,2-diaminobenzene also conveniently affords product 3l, showing that our procedure offers a better performance over that employing just water for the coupling reaction.9a At this point our goal was to demonstrate the peculiar mildness of this protocol especially when compared to procedures based on the utilization of acid-based catalysts or promoters. For this reason, a series of dicarbonyl reactants embedding acid sensitive groups, such as acetals, which are popular protecting groups of alcohols and ketones, were tested with our method. Products 3n and o containing a tetrahydropyranyl group and a 1,3-dioxolanyl group are obtained in very high yields without any trace of compounds arising from cleavage of these protecting groups. A comparison with the same reaction carried out in water shows that in spite of the neutral conditions, compound 3n is obtained in only 65% yield together with a notable amount of various byproducts (Scheme 2). In all the examples reported, the quinoxaline is obtained in pure form after extraction from the NADES with EtOAc, drying and solvent evaporation. Repeating the reaction on a 5 mmol scale for the synthesis of compounds 3a and 3e has revealed an increase in the recorded chemical yield making this procedure rather appealing for large scale applications. For the preparation of these two compounds, green metrics such as the PMI (process mass intensity) and E-factor (environmental factor)24 have been calculated in scaled up reactions, giving interesting values as reported in Scheme 3.
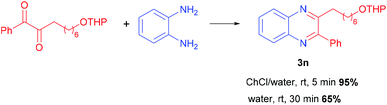 |
| Scheme 2 Comparative results using a NADES and water for the synthesis of 3n. | |
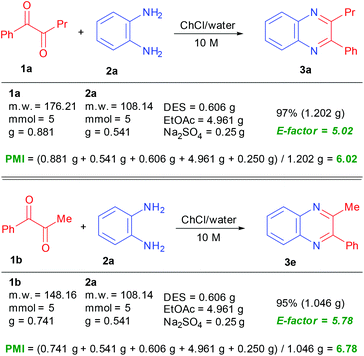 |
| Scheme 3 Green metric calculations (PMI and E-factor) for selected reactions. | |
Although ChCl is a rather inexpensive material, recycling of NADES has been pursued with the aim to reduce the waste produced in our process (Fig. 2). The NADES recovered after solvent extraction by vacuum evaporation is freed of residual ethyl acetate, and then reused for a further six reactions always leading to the corresponding product 3a in good yield.
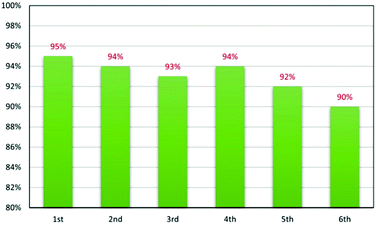 |
| Fig. 2 Recycling of the NADES used in the synthesis of quinoxalines. | |
Conclusions
Synthetic approaches to quinoxalines, although simple, are often unsuitable for the preparation of compounds embedding labile protecting groups or related functional groups. Even protocols carried out under neutral conditions show a limited substrate scope when tested with functionalized substrates. The utilization of the NADES, ChCl/water (1
:
3.3), provides a formidable green medium enabling the fast activation of reactants, and easy recovery of products, which are pure enough to avoid any further purification for practical use. This procedure has been used for the preparation of simple as well as functionalized quinoxalines embedding acetal protecting groups, which are totally unaffected by the reaction conditions. Thus, the devised protocol offers a cheap and competitive method from a sustainable standpoint for the preparation of quinoxalines.
Author contributions
G. L. carried out the experimental work. A. P. and M. P. conceptualized and designed the experiments.
Conflicts of interest
There are no conflicts to declare.
Acknowledgements
We acknowledge financial support from the University of Camerino (FAR program) and MIUR (PRIN 2017, NATURECHEM project, grant no. 2017A5HXFC).
Notes and references
- V. K. Vishwakarma and A. A. Sudhakar, Soft Matter, 2021, 17, 8221–8257 RSC.
- D. S. Chauhan, P. Singh and M. A. Quraishi, J. Mol. Liq., 2020, 320, 114387 CrossRef CAS.
-
(a) C. Sun, C. Zhu, L. Meng and Y. Li, Adv. Mater., 2021, 2104161, DOI:10.1002/adma.202104161;
(b) D. Gedefaw, M. Prosa, M. Bolognesi, M. Seri and M. R. Andersson, Adv. Energy Mater., 2017, 7, 1700575 CrossRef.
-
(a) O. O. Ajani, M. T. Nlebemuo, J. A. Adekoya, K. O. Ogunniran, T. O. Siyanbola and C. O. Ajanaku, Acta Pharm., 2019, 69, 177–196 CrossRef CAS PubMed;
(b) T. Kaushal, G. Srivastava, A. Sharma and A. S. Negi, Bioorg. Med. Chem., 2019, 27, 16–35 CrossRef CAS;
(c) M. Montana, F. Mathias, T. Terme and P. Vanelle, Eur. J. Med. Chem., 2019, 163, 137–147 CrossRef.
-
(a) M. Montana, V. Montero, O. Khoumeri and P. Vanelle, Molecules, 2020, 25, 2784 CrossRef CAS PubMed;
(b) M. Montana, V. Montero, O. Khoumeri and P. Vanelle, Molecules, 2021, 26, 4742 CrossRef CAS PubMed.
-
(a) A. Irfan, S. Ahmad, S. Hussain, F. Batool, H. Riaz, R. Zafar, K. Kotwica-Mojzych and M. Mojzych, Appl. Sci., 2021, 11, 5702 CrossRef CAS;
(b) H. Khatoon and E. Abdulmalek, Molecules, 2021, 26, 1055 CrossRef CAS PubMed;
(c) S. Tariq, K. Somakala and M. Amir, Eur. J. Med. Chem., 2018, 143, 542–557 CrossRef CAS PubMed;
(d) J. A. Pereira, A. M. Pessoa, M. N. D. S. Cordeiro, R. Fernandes, C. Prudêncio, J. P. Noronha and M. Vieira, Eur. J. Med. Chem., 2015, 97, 664–672 CrossRef CAS.
- V. K. Maikhuri, A. K. Prasad, A. Jhab and S. Srivastava, New J. Chem., 2021, 45, 13214–13246 RSC.
-
(a) L. Biesen and T. J. J. Müller, Adv. Synth. Catal., 2021, 363, 980–1006 CrossRef CAS;
(b) G. Yashwantrao and S. Saha, Org. Chem. Front., 2021, 8, 2820–2862 RSC;
(c) R. Singh, D. Bhardwaj and M. R. Saini, RSC Adv., 2021, 11, 4760–4804 RSC;
(d) B. Borah and L. R. Chowhan, RSC Adv., 2021, 11, 37325–37353 RSC;
(e) T. Mathew, A. Á. Papp, F. Paknia, S. Fustero and G. K. S. Prakash, Chem. Soc. Rev., 2017, 46, 3060–3094 RSC.
-
(a) C. Delpivo, G. Micheletti and C. Boga, Synthesis, 2013, 45, 1546–1552 CrossRef CAS for a more recent paper involving heating at 230 °C see:
(b) F. Amaya-García, M. Caldera, A. Koren, S. Kubicek, J. Menche and M. M. Unterlass, ChemSusChem, 2021, 14, 1853–1863 CrossRef PubMed.
-
(a) P. Alam, N. L. C. Leung, H. Su, Z. Qiu, R. T. K. Kwok, J. W. Y. Lam and B. Z. Tang, Chem. – Eur. J., 2017, 22, 14911–14917 CrossRef PubMed;
(b) Z. T. Bhutia, G. Prasannakumar, A. Das, M. Biswas, A. Chatterjee and M. Banerjee, ChemistrySelect, 2017, 2, 1183–1187 CrossRef CAS.
-
(a) S. S. Mahadik, D. R. Garud, R. V. Pinjari and R. M. Kamble, J. Mol. Struct., 2022, 1248, 131541 CrossRef CAS;
(b) H. You, H. Kang, D. Kim, J. S. Park, J.-W. Lee, S. Lee, F. S. Kim and B. J. Kim, ChemSusChem, 2021, 14, 3520–3527 CrossRef CAS;
(c) F. Zhao, T. Sun, Y. Wang, Y. Zhan and W. Yang, Dyes Pigm., 2021, 196, 109763 CrossRef CAS;
(d) B. Pashaei, H. Shahroosvand, M. Ameri, E. Mohajerani and M. K. Nazeeruddin, J. Mater. Chem. A, 2019, 7, 21867–21873 RSC;
(e) T. Huang, D. Liu, J. Jiang and W. Jiang, Chem. – Eur. J., 2019, 25, 10926–10937 CrossRef CAS PubMed;
(f) L. Yu, Z. Wu, G. Xie, C. Zhong, Z. Zhu, H. Cong, D. Ma and C. Yang, Chem. Commun., 2016, 52, 11012–11015 RSC;
(g) X. Xu, Y. Wu, J. Fang, Z. Li, Z. Wang, Y. Li and Q. Peng, Chem. – Eur. J., 2014, 20, 13259–13251 CrossRef CAS.
- A. G. Mulik, D. R. Chandam, D. R. Patil, P. P. Patil, G. N. Mulik, S. T. Salunkhe and M. B. Deshmukh, Res. Chem. Intermed., 2015, 41, 10085–10096 CrossRef CAS.
-
(a) T. R. Sekharan, R. M. Chandira, S. Tamilvanan, S. C. Rajesh and B. S. Venkateswarlu, Biointerface Res. Appl. Chem., 2022, 12, 847–860 Search PubMed;
(b) B. B. Hansen, S. Spittle, B. Chen, D. Poe, Y. Zhang, J. M. Klein, A. Horton, L. Adhikari, T. Zelovich, B. W. Doherty, B. Gurkan, E. J. Maginn, A. Ragauskas, M. Dadmun, T. A. Zawodzinski, G. A. Baker, M. E. Tuckerman, R. F. Savinell and J. R. Sangoro, Chem. Rev., 2021, 121, 1232–1285 CrossRef CAS PubMed;
(c) D. Yu, Z. Xue and T. Mu, Chem. Soc. Rev., 2021, 50, 8596–8638 RSC;
(d) F. M. Perna, P. Vitale and V. Capriati, Curr. Opin. Green Sustain. Chem., 2020, 21, 27–33 CrossRef.
-
(a) M. Zhou, O. A. Fakayode, A. E. A. Yagoub, Q. Ji and C. Zhou, Renewable Sustainable Energy Rev., 2022, 156, 111986 CrossRef CAS;
(b) H. Balaraman, R. Selvasembian, V. Rangarajan and S. Rathnasamy, ACS Sustainable Chem. Eng., 2021, 9, 11290–11313 CrossRef CAS;
(c) Y. Wang, K. H. Kim, K. Jeong, N.-K. Kim and C. G. Yoo, Curr. Opin. Green Sustain. Chem., 2021, 27, 100396 CrossRef;
(d) B. Chen, Z. Peng, C. Li, Y. Feng, Y. Sun, X. Tang, X. Zeng and L. Lin, ChemSusChem, 2021, 14, 1496–1506 CrossRef CAS PubMed;
(e) A. Kalyniukova, J. Holuša, D. Musiolek, J. Sedlakova-Kadukova, J. Płotka-Wasylka and V. Andruch, Ind. Crops Prod., 2021, 172, 114047 CrossRef CAS;
(f) S. Hong, X.-J. Shen, Z. Xue, Z. Sun and T.-Q. Yuan, Green Chem., 2020, 22, 7219–7232 RSC.
-
(a) F. G. Calvo-Flores and C. Mingorance-Sánchez, ChemistryOpen, 2021, 10, 815–829 CrossRef CAS PubMed;
(b) D. A. Alonso, S.-J. Burlingham, R. Chinchilla, G. Guillena, D. J. Ramón and M. Tiecco, Eur. J. Org. Chem., 2021, 4065–4071 CrossRef CAS.
- L. Cicco, G. Dilauro, F. M. Perna, P. Vitale and V. Capriati, Org. Biomol. Chem., 2021, 19, 2558–2577 RSC.
- Y. Nahar and S. C. Thickett, Polymers, 2021, 13, 447 CrossRef CAS PubMed.
- Y. Liu, J. B. Friesen, J. B. McAlpine, D. C. Lankin, S.-N. Chen and G. F. Pauli, J. Nat. Prod., 2018, 81, 679–690 CrossRef CAS PubMed.
- S. H. Zeisel and K.-A. da Costa, Nutr. Rev., 2009, 67, 615–623 CrossRef PubMed.
-
(a) A. Triolo, F. Lo Celso, M. Brehm, V. Di Lisio and O. Russina, J. Mol. Liq., 2021, 331, 115750 CrossRef CAS;
(b) M. S. Rahman and D. E. Raynie, J. Mol. Liq., 2021, 324, 114779 CrossRef CAS.
- H. Zhang, M. L. Ferrer, M. J. Roldán-Ruiz, R. J. Jiménez-Riobóo, M. C. Gutiérrez and F. del Monte, J. Phys. Chem. B, 2020, 124, 4002–4009 CrossRef CAS PubMed.
- R. Gautam, N. Kumar and J. G. Lynam, J. Mol. Struct., 2020, 1222, 128849 CrossRef CAS.
-
(a) A.-M. Dechert-Schmitt, M. R. Garnsey, H. M. Wisniewska, J. I. Murray, T. Lee, D. W. Kung, N. Sach and D. G. Blackmond, ACS Catal., 2019, 9, 4508–4515 CrossRef CAS;
(b) B. C. Raju, N. D. Theja and J. A. Kumar, Synth. Commun., 2009, 39, 175–188 CrossRef CAS.
-
(a) R. A. Sheldon, Green Chem., 2007, 9, 1273–1283 RSC;
(b) P. J. Dunn, Chem. Soc. Rev., 2012, 41, 1452–1461 RSC;
(c) C. Jiménez-González, D. J. C. Constable and C. S. Ponder, Chem. Soc. Rev., 2012, 41, 1485–1498 RSC.
|
This journal is © The Royal Society of Chemistry 2022 |