DOI:
10.1039/D1GC04327G
(Critical Review)
Green Chem., 2022,
24, 1762-1779
Progresses and future prospects in biodegradation of marine biopolymers and emerging biopolymer-based materials for sustainable marine ecosystems†
Received
20th November 2021
, Accepted 2nd February 2022
First published on 2nd February 2022
Abstract
With approximately 250
000 marine species, the ocean is a vast reservoir of biodiversity and an abundant biological resource of natural polymers. The wide variety, renewable nature, tunable physicochemical and structural behavior and appealing biological properties make these marine biopolymers particularly attractive to the scientific community and numerous industrial sectors. As raw materials, they offer novel opportunities for the development of bio-based materials in response to recent demands for biodegradable plastic materials to lower plastic pollution in marine ecosystems. The biodegradation of marine biopolymers and biopolymer-based materials depends on marine environmental conditions such as temperature, pH and in particular microbial population. Marine microorganisms producing biopolymer-degrading enzymes (i.e., hydrolases, lyases, oxidoreductases) are well studied, nonetheless the biodegradation processes of marine biopolymers-based materials in the marine/aquatic environment need further investigation. This review describes various biodegradation parameters and mechanisms of the degradation of marine biopolymers in the marine environment. It also puts emphasis on the marine microorganisms and the corresponding enzymes that catalyze the degradation of different marine biopolymers. Finally, it focuses on the few studies on biodegradation of emerging bio-based materials in aquatic ecosystems.
 François Samalens, Martin Thomas, Marion Claverie, Natalia Castejon  Yi Zhang, Thierry Pigot, Sylvie Blanc, Susana C. M. Fernandes | F. Samalens, PhD candidate, is working on polymer chemistry and cosmetics with the company Les Laboratoires de Biarritz and the research group MANTA. |
| M. Thomas, PhD candidate, is currently working in the photostability and impact of natural UV-absorbing molecules and their conjugates in aquatic environments. |
| Dr Claverie, has interests in enzyme characterization and engineering; enzymatic functionalization of biopolymers through a biomimetical approach for environmental and health applications. |
| Dr Castejon, Marie Curie REWIRE Fellow, is working in novel cascading approaches based on green chemistry to valorize microalgal biomass by proving potential biological activities. |
| Dr Zhang, Assistant Professor, has research interests in enzymology, biocatalysis, marine proteins and bioinformatics. |
| Prof. Pigot, Full Professor, has interests in water quality and the reactivity of organic compounds in aquatic environments and photochemical processes of oxidation. |
| Dr Blanc, CNRS researcher, has interests in analysis of materials by UV visible and NIR spectral characterization taking into account reactivity and interface properties for environmental or health applications. |
| Dr Fernandes, Associate Professor in Natural Polymers, is Chair in valorization of marine bioresources using green chemistry approaches and in development of marine bio-inspired materials. She is the PI of the research group MANTA. |
1 Introduction
During the evolution over millions of years, marine organisms evolved extraordinary physical and chemical characteristics, which include the ability to biosynthesize and biodegrade natural polymers (also known as biopolymers) to support their survival.1 Marine organisms namely algae, plants, animals and microorganisms can provide a large amount of marine biopolymers, which include proteins (e.g., collagen) and polysaccharides (e.g., chitin, chitosan, cellulose, alginate, etc.) for which the annual production represents 1012 to 1014 tons.2 On the other hand, these natural polymers can be converted to corresponding monomers by enzymes present in microbes, bacteria and fungi.3 Therefore, they have great potential to overcome the biodegradability issues related to the synthetic polymers dumped into the marine environment intentionally or unintentionally. Natural polymers are attracting the interest of academics and industrials as biodegradable substitutes in plastics, materials and products where non-biodegradable and fossil-based polymers are currently used.4
Synthetic polymers are derived from petroleum-based sources and are mainly used for the manufacture of plastic products.5 Multiple industries including packaging and food packaging, building and construction, textiles, biomedical, electrical and electronics are producing millions of tons of plastic materials and disposable plastic products every year.6 Plastic production has increased exponentially, from 2.3 million tons in 1950 to 448 million tons by 2015; and it is expected to double by 2050. As consequence, an enormous amount of plastic trash (∼57 million tons annually) is found and detected in several places in the Planet, including in the ocean.
Every year, at least 8 million tons of plastic end up in the ocean. These plastic products are originated from land-based plastic such us urban runoff, beach visitors, packaging, building and construction, textile sectors, and inadequate waste management, and from ocean-based plastic namely fishing industry, nautical activities and aquaculture.7 A major proportion of the plastics that has been found in the Great Pacific Garbage Patch (GPGP) is from fishing nets, ropes and lines sources.8
The rapidly increasing production of synthetic plastic products overwhelms the ability to deal with their recycling and reuse, and in particular environmental (bio)degradation. For instance, most of the plastics made with synthetic polymers, such as olefin-based plastics, polyethylene (PE), polypropylene (PP), vinyl-based plastics such as polyvinyl chloride (PVC) and aromatic plastics (like polystyrene PS), and polyethylene terephthalate (PET) are resistant to environmental (bio)degradation.9 Because of their high molecular weight and unnatural structure, the biodegradation of synthetic polymers by microorganisms takes extremely long time (hundreds of years),10 and consequently, persist for long time in the environment.
Also, under the effect of environmental factors like temperature, ultraviolet (UV) radiation, pH, salinity, currents and wind, these plastics are fractured into microplastics (particles < 5 mm) and nanoplastics (particles < 100 nm) causing a negative impact on marine wildlife and human health.11–13 Often marine organisms are entangled or ingest these plastic particles causing severe injuries and deaths, and also impacting seafood safety and quality and human health.11,12,14 Accordingly, plastic pollution has become one of the most pressing environmental and socioeconomic issues.
In this context, lower the devastating impact of the emerging pollutants in the marine environment is a major challenge in the 21st century. One possible solution, which has been investigated in the last decades, is the use of natural polymers, in particular derived from marine sources as an alternative to synthetic polymers for the manufacture of biopolymer-based materials and products.15–19Table 1 lists the most investigated and used marine biopolymers for the production of biopolymer-based materials, their products and commercial brands as well as their field of application.
Table 1 Structure of marine biopolymers, their products, commercial brands and sector of application
Marine biopolymers and their structures |
Origin |
Products and brands |
Fields of application |
Ref. |
Polysaccharides |
|
Arthropods (shrimp, lobster, crab and insects) and mollusks |
Anti-cholesterol agent, food preservative and food additive body creams, lotions, emulsifying agent, gelling agent, color stabilizer, thickener and stabilizer for sauces. |
Water treatment and purification, food industry, packaging, agriculture, pulp and paper industry, cosmetics, tissue engineering and drug delivery |
19–28
|
|
|
Ultimate Miracle Worker Eye® Cream, Kristin Ess®, BST-Gel®, ChitoFlex® PRO, Protasan™, Reaxon® Shellworks |
|
|
|
Brown seaweed, bacteria |
Paper adhesion agent of tablets inject able fillers, antiacids. Gaviscon Double Action® Peptac® Algycon® Maalox® |
Pharmaceutical, drug delivery, wound healing, tissue engineering, food industry, textile, pharmacy, facial plastic surgery |
29–33
|
|
Fishes |
Films, and other wound dressings, dermal filler, lubricant. Contipro® gels Ordinary® |
Medical and biological application, ophthalmology, tissue engineering, dermatology, cosmetics and treatment for osteoarthritis |
34–38
|
|
Marine red algae |
Texture improvement stabilizer, stabilizer for yoghurt, cheeses and candy. OBC Skin® Florence By Mills® |
Food, biochemicals culture media for microbiology, electrophoresis, chromatography |
39–43
|
Protein |
|
Fishes, marine sponges and jellyfish |
Skin and eye creams, Moisturizer drug supplements. Elemis Pro-Collagen Marine® Collagen cosmetics® |
Biomedical and cosmetic sectors, tissue engineering and cosmetics. |
44–47
|
There is very little data available concerning the production of biopolymer-based materials. For instance, recently, Mintel Companies have estimated that 12
000 hyaluronic acid-based products were launched in 2019.48 The statistics from the European Bioplastics Organization (http://www.european-bioplastics.org/market) show that bioplastics, which refer to plastics made from renewable biomass materials49 represent less than 1% of the plastic produced annually. Nonetheless, grow is expected to increase greatly in the coming years, with projections showing the global production capacities in 2026 of approximately 7.59 million tons.
As it is a growing market, it is primordial to investigate the life cycle of the new biopolymer-based materials from their origin and generation until their (bio)degradation. Some studies have shown that there is still some hurdles to overcome regarding the replacement of synthetic polymers by biopolymers namely the increase in agricultural activity and the land and water use.50,51 In particular, for the marine biopolymers and ensuing materials and products extraction and production, bold policies and actions are urgently needed for a sustainable and socially equitable blue economy.52,53 Also, in the sense to prevent plastic pollution and detrimental effects on humans, the design of marine biopolymer-based materials for biodegradation is required.
With the trend of using marine biopolymer-based materials to replace synthetic plastic in daily life, this review aims: (i) to describe the biodegradation parameters in marine ecosystems; (ii) to do a biodiversity map of relevant microorganisms and corresponding enzymes identified in marine ecosystems; (iii) to report the biodegradation process of biopolymers in marine ecosystems; (iv) to depict the biodegradation mechanisms of marine biopolymers (e.g. chitin, chitosan, alginate, collagen and hyaluronic acid) by hydrolases, lyases and oxidoreductases; and (v) to give an overview of the current researches and the limitations about the (bio)degradation of marine biopolymers and their bio-based materials in the marine environment.
To the best of our knowledge, there is no review paper on the current knowledge of natural polymer-degrading microorganisms regarding marine biopolymers and their bio-based materials. Recently, Sheth et al. detailed the microorganisms degrading synthetic plastics as well as the locations of the current researches in a review published in 2019.54 The only review about the subject has been published in 2020 by Sun et al., where the authors described the current state-of-the-art of marine polysaccharide degrading enzymes. Nonetheless, it is not focused on enzymes coming from the marine environment and does not mention the current knowledge on the degradation of material made of biopolymers.55
2 (Bio)degradability of (bio)polymers in marine ecosystems: general aspects
2.1 Description of the environmental factors of the different aquatic ecosystems
Polymer degradation is extremely dependent on the surrounding environment. Thus, abiotic factors like water salinity, depth, temperature, flow, and biotic factors like microorganisms biodiversity composition are environmental factors to take into consideration.56 Consequently, polymer (bio)degradation is dependent of the aquatic ecosystems generally classified as: freshwater, estuaries, and marine ecosystems.
Freshwater ecosystems
have a small amount of salt dissolved in water. They are often divided into two categories: the ones in which the water is stationary like lakes and ponds, and the ones in which there is a flow of water like rivers. In stationary water ecosystems, the conditions are heterogeneous affecting their populations. For instance, deep oligotrophic lakes have clear and cold water with a low amount of nutrients. Consequently, the microorganisms tend to be less productive. On the other hand, eutrophic lakes are shallow, nutrient rich and warm. Therefore, the productivity is different and it affects the potential biodegradability of organic materials. The microbial diversity of freshwater ecosystems is dominated by cyanobacteria and microalgae.57
Estuaries
are partially enclosed areas located at the junction between freshwater ecosystems and marine ecosystems (ocean). Unlike in marine or freshwater ecosystems, the amount of salt dissolved is not constant and it changes with the flow of water. Because of these particular conditions, the number of microorganism species present in estuaries is lower than that in marine or freshwater ecosystems.58
Marine ecosystems
are characterized by the presence of a high salt content (higher than in freshwater ecosystems and estuaries). These ecosystems are classified according to the distance to the coast and to the water depth. There are two main domains: Benthic and Pelagic (Fig. 1). The Pelagic Domain or open ocean waters correspond to the zones that are not in contact with the seabed (Fig. 1). The organisms living there are not attached to the seabed of the ocean (or sea). In these zones, the majority of organisms that carry out photosynthesis are microscopic: microalgae or bacteria. The principal factor that influences the type of microbial community is the amount of dissolved inorganic matter (nutrients).
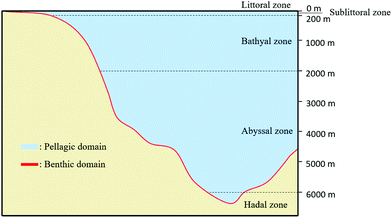 |
| Fig. 1 Representation of the two main domains of marine ecosystems: Benthic Domain and Pelagic Domain (inspired by ref. 59). | |
Benthic Domain, zones located at the interface with the sedimentary deposits of the seabed (Fig. 1), contains a great quantity of nutrients and, consequently the biggest activity of microorganisms. Sponges, crustaceans or seaweed are commonly found in these areas. The population highly depends on temperature and substrate present at the seabed (e.g., sand, mud). For instance, coral reefs or mangrove swamps are only found in warm Benthic Domains.59 Most of biodegradation is achieved at the interface of water and sediments because it contains a large quantity of materials that are able to support the growth of microorganisms. According to the water depth, Benthic Domain is often divided as follows: the littoral zone; the sublittoral zone (between 0 to 200 m) where it has been found that some microorganisms like Streptomyces sp. D1 release amylase;60 the bathyal zone (between 200 to 2000 m) where microorganisms like Thalassomonas sp. JAMB-A33 release agarase;61 the abyssal zone (between 2000 to 6000 m) where the quantity of nutrients are low due to the absence of light;62 and the hadal zone (bellow 6000 m).
Depending on the marine ecosystem, the activity of microorganisms’ changes and therefore, the biodegradation kinetics. A study compared the activity of hydrolytic enzymes (e.g., chitobiase, lipase and β-glucosidase) in different marine environments, and it found that the enzymatic activity was higher in shallow water sediments than that in deep-sea sediments, while the activity exponentially decreased with the sediment depth.63
Since our focus is on marine ecosystems, the biodegradation of polymeric matter will be discussed with environmental conditions like temperature and pH which are major factors influencing the microorganism's proliferation and activity in marine ecosystem.
2.2 Biodegradation factors in marine ecosystem
2.2.1 Effects of environmental parameters.
Environmental conditions at specific pH and temperature affect the bacterial population and, consequently the properties of the enzymes.64 It has been demonstrated that slight changes in pH values (less than 1) can lead to a modification of the bacterial community. Moreover, some enzymes derived from marine bacteria living in extreme conditions (extremophiles) show the highest activity at extreme temperature and pH. For instance, an amylase obtained from a bacteria in Antarctica had maximum activity at around 10 °C.65
On the other hand, some (bio)polymers-degrading enzymes show optimal catalysis at non-extreme conditions. For example, the biodegradation of bio-sourced polymers like poly(3-hydroxybutyrate) (P(3HB)), belonging to the family of polyhydroxyalcanoates (PHA), by bacteria Pseudomonas stutzeri YM1414 present in lake water was investigated.66 It was found that at pH of 9.5 and temperature of 55 °C, the depolymerases secreted by the bacteria had the highest activity for the degradation. Various alginate lyases from molluscans also showed the highest activity at pH values from 5.6 to 8.5 and temperature ranges between 35 to 53 °C.67
2.2.2 Effects of (bio)polymer properties.
Biodegradation process is also dependent on the physico-chemical properties of (bio)polymers. Surface properties (e.g., surface area, roughness), physic-chemical properties (e.g., chemical structure, molecular weight and distribution, crystallinity, crystal structure, amorphous nature), thermal properties (e.g., glass transition and melting temperatures), mechanical properties (e.g., modulus of elasticity), hydrophilicity, hydrophobicity, shape and porosity of the (bio)polymers are all the factors that can influence biodegradation processes.68
All these properties have been studied for the biodegradation of synthetic polymers, however, only few studies have been done regarding natural polymers. For instance, the biodegradation of cellulose was greater when it had a low degree of crystallinity.69 Biodegradation of natural polymer chitin by enzymes (like lysozyme or lipase) is variable according to the structure of chitin whiskers (are nanocrystals of chitin obtained after removing the amorphous domains of the biopolymer).70 When the deacetylation degree of chitin increases, the biodegradation rate increases. Liu et al. described that the presence of amino groups allowed a better combination between the enzymes and the chitin whiskers70 that helps with the enzymatic biodegradation. Besides, the shape of (bio)polymers in marine ecosystems affects its biodegradation. It was demonstrated that PHA films were degraded faster than PHA pellets due to their larger surface area. The larger polymer/water interface improves the adhesion of the microorganisms to the surface of the polymer.71
2.3 Biodiversity map of relevant microorganisms and enzymes in marine ecosystem
A wide variety of microorganisms like anaerobes, aerobes and photosynthetic bacteria as well as fungi present in marine ecosystems are able to degrade synthetic and natural polymers by releasing molecules and/or enzymes. These microorganisms are diverse, for example, it is possible to find hundreds of millions of bacteria per gram of marine sediment only.72 The degradation of synthetic polymers by microorganisms has recently been reviewed.54,73 Recently, Sheth et al. reviewed 50 different species of marine bacteria, fungi and enzymes that have been isolated to degrade synthetic polymers like Nylon, PET (polyethylene terephthalate), PU (polyurethane), PE (polyethylene), PVC (polyvinyl chloride).54 Nonetheless, the research on polymer-degrading organisms is still scarce. Herein, for the first time, a biodiversity map regarding the location of the different extracted marine biopolymers-degrading enzymes is presented in Fig. 2 and an updated list in Table 2 (and ESI Table S1†). The enzymes that are listed belong to the Carbohydrate-Active enZYmes database (CAZy, http://www.cazy.org) which list and classify the enzymes that catalyze the breakdown of polysaccharides (marine and non-marine). The present list of biopolymer-degrading organisms and enzymes was obtained by reviewing the current literature using different database like Scopus and Google Scholar with the keywords: ‘enzyme’, ‘degradation’, ‘degrading’, ‘marine’, ‘biopolymer’, ‘natural polymer’, ‘organism’, ‘polysaccharide’, ‘agar’, ‘starch’, ‘chitin’, ‘chitosan’, ‘cellulose’, ‘alginate’, ‘pullulan’, ‘xylan’, ‘hyaluronic acid’, ‘collagen’. As displayed in Fig. 2, the identified microorganisms and their corresponding enzymes are mostly from Japan, Pacific Ocean, Europe and Antarctica.60,61,65,74–104 The identified microorganisms were isolated from marine organisms (in orange pastel color), water (Freshwater or Seawater, in blue color) or sediments (in green pastel color) from the Benthic Domain, and most of the responsible enzymes are hydrolases from bacteria (Fig. 2 and Table 2).
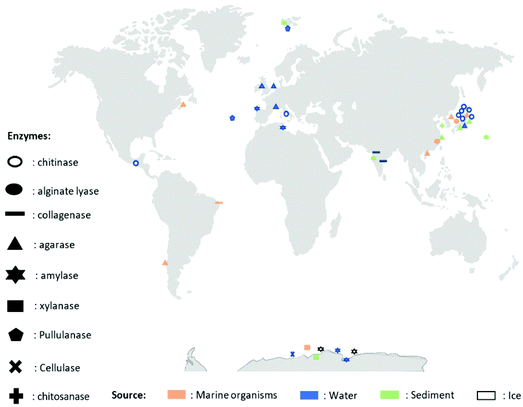 |
| Fig. 2 Map representing different enzymes degrading biopolymers, their extraction location and their source. | |
Table 2 List of microorganisms and enzymes degrading polysaccharides and proteins: agar, starch, chitin, chitosan, cellulose, alginate, pullulan, xylan, hyaluronic acid, collagen in marine environments
As showed in Fig. 2 and listed in Table 2, there are diverse marine microorganisms from different marine ecosystems producing enzymes that are able to degrade natural polymers in diverse conditions. For instance, the marine organisms known as extremophiles, organisms that live in extreme conditions of temperature and pH, present different properties from those living in “normal conditions”. As example, extremophiles are: (i) psychrophiles living in environments of low temperature (between −2 and 20 °C); (ii) thermophiles living in high temperature mediums (between 55 and 113 °C); (iii) acidophiles living in acidic mediums (pH < 4); and (iv) alkaliphiles living in basic mediums (pH > 9).105
The enzymes that can break down chemical bonds between monomers of natural polymers are mainly hydrolases and lyases. Chitinases (biosynthesize by Vibrio fluvialis and Vibrio parahaemolyticus74), alginate lyases (by Microbulbifer sp. ALW and Cobetia sp. NAP175,76) or agarases (by Vibrio sp. JT0107 and by Alteromonas agarlyticus GJ1B84,86) have been reported. Regarding extremophilic organisms, Alteromonas sp. TAC 240B, a psychrophile found in Antarctica, produced amylase, an enzyme degrading starch;77Flavobacterium frigidarium sp. was found in Antarctica producing xylanase, an enzyme degrading xylan.78
2.4 Biodegradation process of (bio)polymers in marine ecosystems
The biodegradation process via the action of microbial enzymes occurs through a sequence of steps, i.e. biodeterioration (initial change of physical and chemical properties of the polymer), bio-fragmentation (disintegration of the polymeric structure into smaller and simpler fragments via enzymes) and bioassimilation (ingestion of molecules by microorganisms),106 as described in Fig. 3.
 |
| Fig. 3 Different steps of biodegradation of (bio)polymers by bacteria: biodeterioration, biofragmentation, bioassimilation. (inspired by ref. 54). | |
2.4.1 Biodeterioration.
The deterioration process of the polymers in the marine environment is considered to be the same whether it is synthetic or natural. It is a combination of abiotic (caused by the environmental conditions) and biotic factors (caused by the action of living organisms) leading to the fragmentation of the polymer chains and a deterioration of the global shape of a material, resulting in the structure deterioration.107,108
Microorganisms like bacteria, algae or fungi can deteriorate the polymers mechanically, chemically or enzymatically.10,109 The microorganisms deteriorate (bio)polymers in a physical way to increase the size of the pores in polysaccharides and proteins to weaken the material.110,111 Some microorganisms like chemolithotropic or chemoorganotrophic bacteria can degrade polymers in a chemical way (i.e., Nitrobacter spp. ). They produce acids (e.g., nitric acid, sulphuric acid) known to deteriorate organic matter. These two families of microorganisms are different in terms of the way that they obtain energy to produce acids. Chemolithotropic bacteria use inorganic sources whereas chemoorganotrophic microorganism use organic matter.112–114 As the biodeterioration goes on, the water in environment cause swelling of the (bio)polymers, facilitating the degradation. Moreover, (bio)polymers can also undergo biodeterioration by enzymes produced by marine microorganisms. Even synthetic polymers like polyvinylchloride and polyamide that are known to be hardly degradable115 could be deteriorated by enzymes from marine organisms like esterases or proteases.116 For instance, Webb et al. showed that plasticized polyvinyl chloride was deteriorated by esterases.117 The degradation of polymers derived from natural sources like poly(L-lactide) in seawater was studied with enzymatic biodeterioration which was described as a bulk erosion of the polymers.118 There are two types of erosion when it comes to enzymatic biodeterioration: the surface erosion traduces a loss of matter but no change in the molecular weight; and the bulk erosion happens when the molecular weight of the polymer is reduced due to bond cleavage.119 However, the bulk erosion can belong to biofragmentation as it breaks chemical bonds.
2.4.2 Biofragmentation and enzymatic hydrolysis.
Biofragmentation is the process that reduces the molecular weight of a (bio)polymer by cleaving bonds to produce oligomers and monomers. This step is necessary to allow bioassimilation in the microbial cells because full-size polymers are unable to cross cell membranes.
There are many different microorganisms taking action during this step. They secrete specific enzymes, and each one has specific function. There are two main reactions during biofragmentation: enzymatic hydrolysis and enzymatic oxidation. The hydrolases in this step are capable of converting the carbohydrates into sugars, lipids into long chain fatty acids, and proteins into amino acids.120,121 Taking polysaccharides as an example, enzymatic hydrolysis reaction of the glycosidic bond is catalyzed and usually requires a proton donor (HA), and a nucleophile or a base (B-). If the polymer is hardly hydrolzyed because of its crystallinity, oxidoreductases (mono-oxygenases, di-oxygenases, oxidases) can take part in the biofragmentation step. They are capable of oxidizing polymers to create alcohols.
2.4.3 Bioassimilation.
After the biofragmentation step, the biodegradation of the material ends with the bioassimilation (Fig. 3). The monomers formed during the bioassimilation depends on the type of microorganism. The monomers can cross the cellular membrane of microorganisms giving the cells sources of energy to grow (e.g., carbon, oxygen…). The molecules entering the cells are oxidized and participate in the formation of adenosine triphosphate (ATP). The molecules can be assimilated by organisms using aerobic respiration or anaerobic respiration.107
3 Biodegradation mechanisms of marine biopolymers
An increasing number of novel materials are made up from natural polymers synthesized by marine organisms. They have been used in various applications, including in medical or cosmetic products.122,123 Similar to the fate of synthetic polymers (e.g., plastic), the novel marine biopolymer-based materials can also end up in the ocean, therefore, it is important to understand their biodegradation mechanisms and determine their impact on marine ecosystems.
3.1 Biodegradation mechanisms by hydrolases and lyases
As described previously in section 2.3, hydrolases and lyases are the two major enzyme classes (EC 3 and EC 4, respectively) that can catalyze the degradation of marine biopolymers. Herein, the biodegradation mechanisms of principal marine polymers including polysaccharides namely chitin and chitosan, alginate, hyaluronic acid, as well as a protein, collagen are summarized and compared.
3.1.1 Biodegradation of marine polysaccharides.
3.1.1.1 Chitin and chitosan.
Chitin is a linear long-chain polysaccharide composed of N-acetylglucosamine units linked via β-(1 → 4) bonds.124 Chitin is considered the most abundant biopolymer in the biosphere after cellulose.125 Chitin is present in arthropods (shrimp, lobster, crab and insects) and mollusks (squid pen). Moreover, in the marine environment a variety of unicellular eukaryotic organisms synthesize chitin such as ciliates, cnidesporida, rhizopoda, diatoms, yeast and fungi.126–128 Its chemical structure is similar to cellulose but chitin contains predominantly acetamide groups (–NHCOCH3) or residual amine groups (–NH2) at the C-2 position. In nature, chitin exists as ordered crystalline microfibrils wrapped in protein and embedded in minerals like calcium carbonate and residual calcium phosphate. It is insoluble in water and in most organic solvents.129 Chitin is present in three different polymorphic forms α-, β- and γ-chitin that have different packing and polarities of near chains in the successive sheets of which they are composed. It occurs in different morphological forms in living organisms such as small microfibrils with diameter of 2.5–3 nm (in arthropod cuticles), large microfibrils 9 to 27 nm in diameter and up to 1 μm length (in fungi Vestimentifera worms) and nematic liquid crystal structure (in Pogonophora). Due to its abundancy and unique physicochemical and biological properties like biocompatibility, antimicrobial activity, and biodegradation, chitin is becoming one of the most important chemical raw materials for the fabrication of emergent sustainable polymer materials. In the last decades, important advances have been reported in: chitin extraction and solubilization,15,130,131 use of chitin as functional materials for environment depollution,132–134 chitin nanocrystals/nanofibers and polyols synthesis17,131,135–139 and characterization,18 as well as biomimetic materials design for tissue engineering.140,141
Chitosan (β-1,4-linked glucosamine) is the main chitin derivative, and it has emerged as a relevant bio-based polymer for the fabrication of novel sustainable materials. Chitosan is obtained via the deacetylation of chitin with concentrated sodium hydroxide. It is considered that when the degree of deacetylation is higher than 40–60%, the biopolymer is called chitosan (corresponding to the fraction for which the polymer becomes soluble in acidic solutions). Chitosan has many applications such as water treatment and purification (removal of heavy metal ions and dyes),20 in food industry as anti-cholesterol agent, food preservative and food additive,21 packaging,22 agriculture (seed and fertilizer coating),23 pulp and paper industry (surface treatment, adhesive paper),19,24 cosmetics (body creams, lotions, etc.),25 tissue engineering and drug delivery.26
The biodegradation of chitin and chitosan as such has been found with function of endoenzymes and exoenzymes. There are two pathways of chitin biodegradation characterized: chitinolytic pathway and chitosan pathway (Fig. 4(a)).142
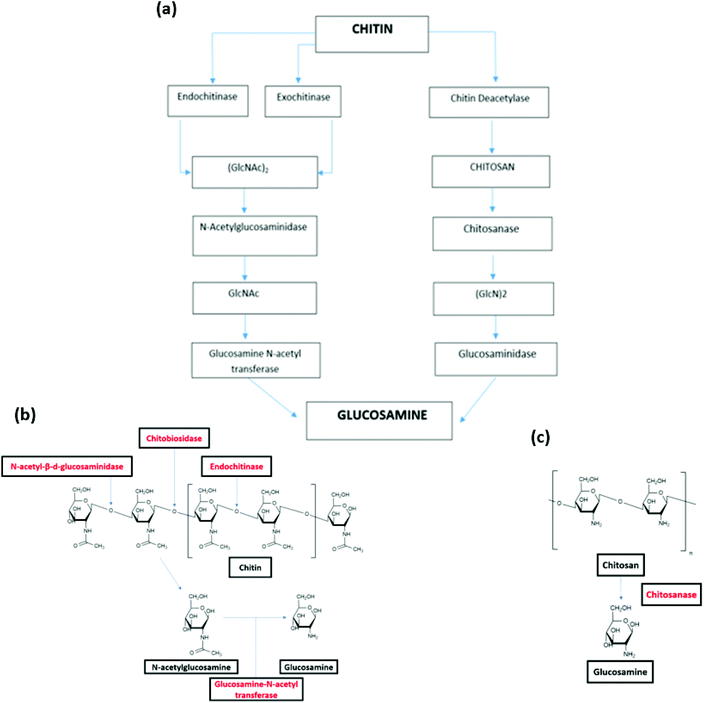 |
| Fig. 4 (a) Pathways of chitin biodegradation in marine environments via the chitinolytic and chitosan pathway.142 (b) Degradation of chitin by endoenzymes and exoenzyme.146 (c) Degradation of chitosan by chitosanase.146 (a) Reprinted with permission from ref. 142, copyright American Chemical Society, 1998. (b) Reprinted with permission from ref. 146, copyright Springer Nature, 2015. (c) Reprinted with permission from ref. 146 copyright Springer Nature, 2015. | |
In the chitinolytic pathway, the depolymerisation of chitin is realized by endochitinases (chitinase) and exochitinases (chitobiase and chitobiosidase). Endochitinases hydrolyze the chitin resulting in the formation of oligosaccharides of different chain lengths, however this endo-hydrolysis does not occur on small chains containing less than three acetyl glucosamine residues. Endochitinases cleave bonds randomly along the chitin strand and form loose ends. It is worth noting that the rate of hydrolysis is directly in proportional to the degree of polymerization of the chitin chains.143 After the explosion of a number of chain ends, exochitinases catalysis allow the production of disaccharides, transforming chitin into chitobiose.144 Subsequently, N-acetyl-β-D-glucosaminidase converts these disaccharides into N acetyl-glucosamine (GlcNAc). The monosaccharides products can be directly absorbed by cells. Finally, the glucosamine N-acetyl transferase catalyzes the conversion of N-acetylglucosamine to glucosamine (Fig. 4(b)).145
In the chitosan pathway, there is a partial or total deacetylation step, where chitin deacetylase change chitin into chitosan. Chitosan is then hydrolyzed by chitosanase to oligomers of glucosamine ((GlcN)2) (Fig. 4(c)). These oligomers are hydrolyzed by glucosaminidase, yielding to free glucosamine residues that can be used as direct substrates by cells.146
The degree of deacetylation of chitin and chitosan have a great impact on the biodegradation. For instance, chitosan with low degree of deacetylation (<65% of acetylated units) was proven to be degraded by chitinases.147 Chitinase are mainly synthesized by bacteria and fungi, which ingest chitin in their diet. The marine organisms that produce chitinase and chitobiase include Vibrio fluvialis, Vibrio parahaemolyticus or Clonostachys rosea74,80 (Fig. 2 and Table 2). Chitosanase hydrolyze the breakdown of chitosan.148 This enzyme has been found in 25 types of fungi and 15 types of bacterial strains comprising marine bacteria such as Bacillus sp. and Pseudoalteromonas sp. SY39.149,150
3.1.1.2 Alginate.
Alginate is a polysaccharide formed by linear block copolymerization of (1 → 4)-linked β-D-manuronic acid (M block) and α-L-guluronic acid (G block).151 The most important source of this natural polymer is brown seaweed, including Phaeophyceae, Laminaria hyperborea, Laminaria digitata, Laminaria japonica, Ascophyllum nodosu and Macrocystis pyrifera.151 Moreover, alginates are also synthesized by some bacteria such as Pseudomonas aeruginosa and Azotobacter vinelandii. Its intrinsic properties make alginate, one of the most promising biopolymers for a variety of applications in the pharmaceutical,30 drug delivery,31 wound healing,32 tissue engineering33 and food industry.152 Alginates are widely used due to their biodegradability, biocompatibility and low toxicity as well as their low extraction and purification costs. In addition, alginate can be processed into various forms such as matrices, hydrogels, particles and beads that make it very attractive.
Alginate is naturally degraded by enzymes, alginate lyases that can be produced by marine bacteria, such as Cobetia sp. NAP1 and Microbulbifer sp. ALW1,75,76 (Fig. 2 and Table 2). These enzymes act on the glycosidic linkage of alginates, to produce polysaccharides of different carbon chain lengths (Fig. 5).153
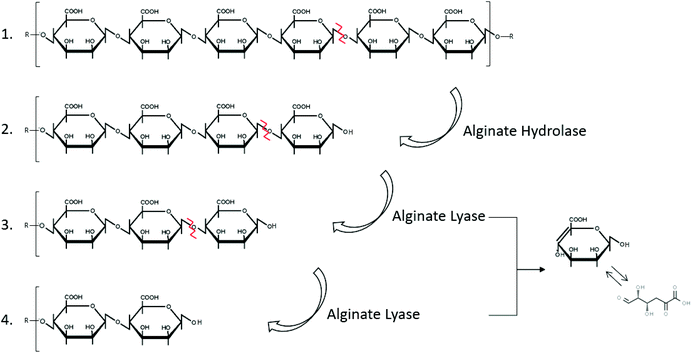 |
| Fig. 5 Degradation of alginate by the enzymes hydrolase and lyase (inspired by ref. 154). | |
The alginate degrading enzyme complex consists of at least two different enzyme components: alginate lyase (eliminase) and endo-alginate hydrolase. These enzymes reduce the viscosity of alginate by scissoring the polymer chains resulting in the formation of saturated and unsaturated uronic acid with non-reducing groups.154 The unsaturated mannuronic acid can be converted to 4-deoxy 5-keto uronic acid via tautomerism.
3.1.1.3 Hyaluronic acid.
Hyaluronic acid (hyaluronan, HA) is a linear high molecular-weight polysaccharide composed of D-glucuronic acid and N-acetyl-D-glucosamine units linked by (1 → 4) and (1 → 3) bonds.34 Each repeating disaccharide unit has one carboxylate group, four hydroxyl groups and an acetamido group. It belongs to the glycosamino glycans (GAGs) category of substances that include chondroitin sulphate, dermatan sulphate and heparin sulphate. Unlike other members in the GAG group, HA is devoid of sulphate group. It is highly hydrophobic with lubricating property. In terms of configuration, it exists as random coils that entangle at very high molecular weight to form viscoelastic gels. HA is abundantly available in living organisms especially the soft connective tissues. In marine organisms, HA can be found in cartilages and vitreous humor of fish.155 HA has important medical and biological applications due to its nonimmunogenic nature. Its biocompatibility and biodegradability make it useful in, ophthalmology,35 tissue engineering,36 dermatology,37 cosmetics38 and treatment for osteoarthritis.34
Hyaluronic acid is degraded by enzymes such as hyaluronidase (hyaluronate lyase), β-D-glucuronidase and β-N-acetyl-hexosaminidase.156,157 The enzyme hyaluronidase cleaves the high molecular weight hyaluronic acid into smaller oligosaccharides. Subsequently, the β-D-glucuronidase and β-N-acetyl hexosaminidase degrade the oligosaccharide fragments by removing non reducing terminal sugars.158 The degradation of hyaluronate lyase primarily produce unsaturated disaccharide and 2-Adgpuag (2-acetamido-2-deoxy 3-O-(β-D-gluco-4-enepyranosyluronic acid)-D-glucose) as the final products (Fig. 6).159 In marine environment, the only bacteria identified that releases hyaluronidase is Vibrio sp. FC509.160
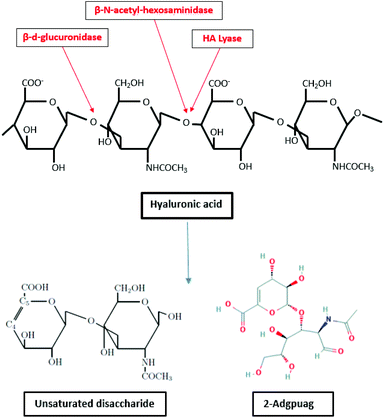 |
| Fig. 6 Degradation of hyaluronic acid by enzymes β-D-glucuronidase, hyaluronate lyase and β-N-acetyl-hexosaminidase (inspired by ref. 157). | |
3.1.1.4 Agar.
Agar is a complex polysaccharide found in the cell walls of marine red algae. The structure of agar varies depending on the source, but it is commonly considered that this polysaccharide consists of mainly 3,6-anhydro-L-galactoses, D-galactoses and L-galactoses units linked by β-(1,4) and α-(1,3) linkages. The main chemical structure of agar is agarose and the main repeating moieties are 3-O-linked β-D-galactopyranose (G) and 3,6-anhydro-α-L-galactose (LA).161 Agar may also refers to moieties called porphyrobiose consisting of 3-O-linked β-D-galactopyranose (G) and α-L-galactose-6-sulfate (L6S). Due to the gelling properties, agar is used in various industries such as food (food additive40) and biochemicals (culture media for microbiology41), and agarose gel electrophoresis42 and chromatography.43
The complete mechanism of biodegradation of agar by marine organisms and enzymes has been reviewed by Chi et al.162 Herein, the focus of this review is on the degradation of agarose by marine enzymes. The biodegradation of agarose occurs in two pathways depending on if the structure is α-agarose or β-agarose. Agarose is initially cleaved by the enzymes α- or β-agarase at the positions α-(1,3) or β-(1,4), releasing agarotetraose or neoagarotetraose, respectively. Then, these products are broken down to agarobiose (also called neoagarobiose). Finally, agarobiose hydrolases cleave the β-(1,4) or the α-(1,3) bonds to form the monomeric units 3-O-linked β-D-galactopyranose (G) and 3,6-anhydro-α-L-galactose (LA), respectively. The porphyrobiose moieties are further biodegraded in a different pathway called the β-porphyran hydrolytic pathway involving other enzymes like β-porphyranase that hydrolyzes porphyran at the β-(1,4) linkage. There have been several agarose enzymes reported from marine bacteria in sea water, marine sediments or marine algae and mollusks. For instance, Alteromonas agarlyticus GJ1B in seawater,86Agarivorans sp. HZ105 from marine sediments88 and Alteromonas sp. SY37-12 from red algae89 were reported to express agarase. The Fig. 7 represents one of the possible agarose degradation pathways by a freshwater bacterium Cellvibrio sp. KY-GH-1 (KCTC13629BP) using only β-agarases.163
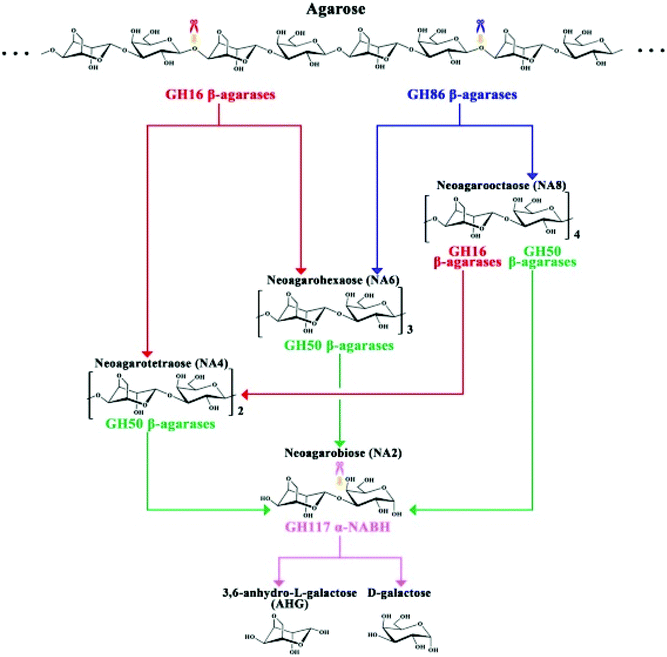 |
| Fig. 7 Schematic diagram of the process by which Cellvibrio sp. KY-GH-1 degrades agarose. Reprinted with permission from ref. 163, copyright Elsevier, 2019. | |
3.1.2 Biodegradation of marine proteins.
3.1.2.1 Collagen.
The collagen molecule contains three peptide chains that are wound together to form a triple helix. In nature, it is found as the insoluble polymerized form of fibers and filaments. The primary sequence of collagen comprises a repeating tripeptide (Gly-X-Y), in which X is proline and Y is hydroxyproline. The chains form a left-handed polyproline helix structure and three chains interact and adopt a right-handed triple helix.164 Collagen molecules are mostly found in fibrous tissues in which each molecule is joined to the other in an end to end pattern.165 Up to date, about 20 different types of collagens have been found (type I, II, III, …). In the marine environment, collagen can be found in fishes, marine sponges or jellyfish.166 Due to its diverse properties, such as haemostatic effect, low antigenicity, and good mechanical characteristics, collagen has potential in biomedical and cosmetic sectors. Moreover, it is a promising candidate in tissue engineering45 and cosmetics46 because of its excellent biocompatibility and biodegradability.47
Collagen is degraded by microorganisms that release collagenases, and some of those bacteria in the marine environment are Pseudomonas and Pseudomonas marinoglutinosa (Fig. 2 and Table 2).82,83 Collagenases are endopeptidases that can digest native collagen in the triple helix region. Collagenases catalyse the breakdown of collagen molecules to collagen fragments by breaking the peptide bonds (Fig. 8). Collagen fibrils (aggregates of collagen molecules) start degrading from the exterior. During the biodegradation process, enzyme collagenase first binds to the triple helix at the outer surface to initiate the degradation. As the degradation proceeds, the enzyme gains access to the inner molecules resulting in the cracking of the triple helix. Further degradation of collagen molecules by the actions of other non-specific enzymes (like gelatinases and proteinases) that can cause cleavage of the primary protein fragments into small peptides and amino acids.167
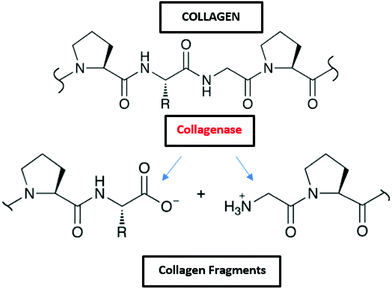 |
| Fig. 8 Degradation of collagen by the enzyme collagenase. | |
3.2 Biodegradation by oxidoreductase
Lytic polysaccharide monooxygenases (LPMOs, EC 1.14.99.54) belong to oxidoreductase (EC 1), and it is non-specific to the degradation of polysaccharides. LPMOs are found in fungi and bacteria, which catalyzes the cleavage of glycosidic bonds of polysaccharides by hydroxylation of one of the carbons in the bonds. LPMOs are mono-copper enzymes and the first LPMOs were discovered in the early 1990s but at the time they were considered as glycoside hydrolases that degrade cellulose. In 2011, they were named polysaccharide monooxygenases (PMOs) and then LPMOs.168 The first fungal LPMO isolated was from Talaromyces cellulolyticus and was named as TrCel61A followed by another LPMO from Trichoderma reesei namely TrCel61B. Its function resulted in hydrolysis of cellulose but its crystal structure didn't support hydrolase characteristics.169,170 LPMOs have a flat surface on the overall structure with a putative metal-binding site. Its structure include a copper coordination sphere composed of three nitrogen ligands coordinated by two histidine residues,171,172 and its unique structure partially decides its function to oxidize polysaccharides.173 LPMOs can break down the structure of polysaccharide chains in a crystalline environment. It also eases the access to the biopolymer for hydrolytic enzymes. LPMOs act on polysaccharides in different ways: either with a monooxygenase reaction or peroxygenase reaction (Fig. 9).174 Both mechanisms lead to the hydroxylation of carbons C1 or C4 in the polysaccharides. Many LPMOs have been reported to work on polysaccharides like xylan, chitin, cellulose or starch.173 Recently, Vaaje-Kolstad et al. showed that dioxygen is essential for the monooxygenase reaction and therefore, for the degradation of polysaccharides. Hydrogen peroxide is formed during the reaction because of the reduction of O2 and because of the oxidase activity of LPMOs. After the reduction of O2, H2O2 act as a co-substrate enabling further catalytic cycles of the enzyme.174,175 The reactions involved in the catalysis are shown in the Fig. 9(a) and (b) with the LPMOs from Lentinus similis (namely, LsAA9A).
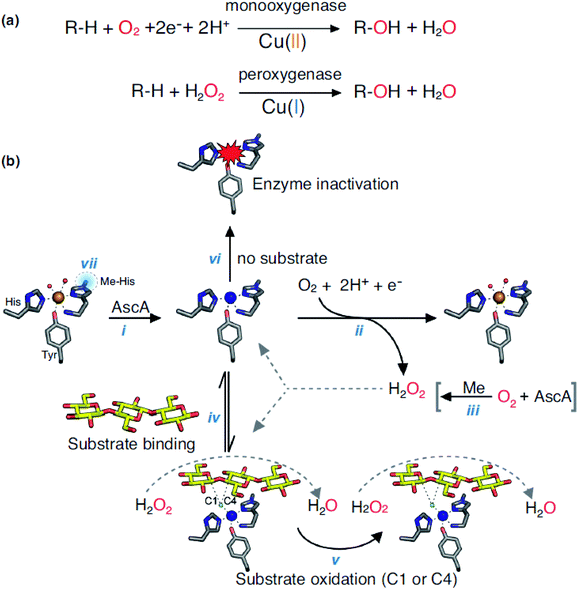 |
| Fig. 9 (a) Monooxygenase and peroxygenase reactions occurring with LPMOs and (b) overview of LPMOs reactions (LsAA9A). Reprinted with permission from ref. 173 copyright Elsevier, 2019. | |
Studies on the degradation of chitin by LPMOs have also been investigated. Kuusk et al. studied the kinetics of this reaction with the AA10 LPMO from Serratia marcescens, also known as SmLPMO10A176 and proposed a reaction scheme for the degradation of chitin nanowhiskers. It seems that the degradation of chitin by LPMOs highly depend on the O2, H2O2 and polysaccharide concentration. Only a few LPMOs from the marine environment have been described, which include one LPMO in a shipworm symbiont: Teredinibacter turnerae.177
4 Biodegradation of marine bio-based materials in marine ecosystem: where do we stand today?
Contrarily to the investigation on biodegradation of natural polymers, up to date, few studies have been done on the biodegradation of biopolymer-based materials. Some of these studies have been done in aquatic ecosystems. Brandl et al.178 carried out the biodegradation experiments of polyhydroxyalkanoates (PHA) bottles in a Swiss lake, and Imam et al.179 studied the biodegradation of starch-polyethylene films in a river. As for the marine environment, biodegradation studies have been made on films and bags containing starch.180,181 The biodegradation of polylactic acid (PLA)-based materials also had a few reports in seawater and marine ecosystems, which includes: PLA films118,182 as well as flax/PLA composites,183 but they showed very little biodegradation in the marine environment.
Regarding the marine biopolymer-based materials, the degradation studies in the marine environment is scarcely found with exceptions on chitin and chitosan films. It is probably because that chitosan-based films for food packaging has been developed and ready for commercialization184 like “The Shellworks” using chitin and “CuanTec” using chitosan. However, the degradation studies for chitin and chitosan films were mainly in soil environment with only a few in aquatic environments. The biodegradation rate of chitin films was higher than chitosan films using degrading enzymes from soil (Sphingobacterium multivorum).185 Another biodegradation study on polyethylene (PE)-chitin and polyethylene-chitosan films in soil environment was reported with bacteria extracted from soil (Serratia marcescens, Pseudomonas aeruginosa),186 and results showed that 100% of the chitin and chitosan films were degraded in soil environment after six months, for which rate of biodegradation was higher for PE-chitin and PE-chitosan films than starch-based films. Marine bacterium Pseudoalteromonas sp. was reported to colonize on chitin films and the bacterial chitinase was assessed187 but no evaluation was found on the biodegradation of chitin films by such bacteria. There is one study reporting the biodegradability of polyhydroxybutyrate (PHB)-chitin and PHB-chitosan films in river water. The blended films degraded over 60% after 30 days showing a better biodegradability than films made of pure biopolymer.188
Monitoring the biodegradation of materials in environmental conditions can be done in various ways. It can be done in laboratory with enzyme assays, plate tests, respiration tests. Still, the best measure of the fate of a material is by exposure in a marine environment. However, the results are relevant only to the specific environment it was exposed to. The material can be then analysed by different methods to assess its degradation for instance: visual examination, infrared or ultraviolet spectroscopy, nuclear magnetic resonance, X-ray diffraction, etc.
As shown, in this section, there is a limited knowledge on the biodegradation mechanisms and experiments of biopolymer-based materials, including in marine environments. This shows the need for future research to determine and define methods to assess biodegradation in marine ecosystems, and to suggest novel directions on the development of sustainable bio-based materials and products and their rapid biodegradation.
5 Conclusion and future perspectives
The problematic biodegradation of petroleum-based polymers in the marine environment is a serious environmental issue that needs to be addressed. The natural polymers derived from marine sources represent an alternative to petroleum-based products as they are biodegradable and renewable.
In this sense, marine biopolymers have shown a great interest in many commercial applications in cosmetics, packaging, construction or medicine. Moreover, it is highly optimistic regarding the potential fast biodegradation of those marine biopolymers in the marine ecosystems by several microorganisms. To date, different biopolymers-degrading enzymes were identified in marine environment, including hydrolases, and lyases and LPMOs. Their catalysis mechanisms of marine polymers are well described; nevertheless, the number of isolated organisms and enzymes in this regard is limited across the scientific literature, suggesting that there are many to discover. Also, the elucidation of their biodegradation in marine ecosystem is necessary to encourage their use in future.
This review paper fills up a gap to describe systematically the biodegradability of must popular marine biopolymers in the marine environment. Nonetheless, it was observed that there is a limited knowledge on the biodegradation mechanisms of biopolymer-based materials, including in marine environments.
In this context, we would suggest some directions for future scientific research: (i) to isolate microorganisms from different marine ecosystems (including, extreme environments) for the discovery of new biopolymer-degrading robust enzymes; (ii) to focus on biodegradation experiments of biopolymer-based materials and products; and (iii) to understand and describe the biodegradation mechanisms of biopolymer-based materials and products to accelerate their commercialization and use.
This review is a support for the development of sustainable marine environments and the green strategies to overcome marine environmental concerns.
Conflicts of interest
There are no conflicts to declare.
Acknowledgements
This work was carried under the framework of E2S UPPA Partnership Chair MANTA (Marine Materials) funded by the ‘Investissements d'Avenir’ French program managed by ANR, grant number #ANR-16-IDEX-0002; by the Région Nouvelle-Aquitaine and by the Communauté d'Agglomération du Pays Basque (France); and by the FLAG “Côte Basque-Sud Landes” through the community-led local development (CLDD: ref number # PFEA621219CR0720005 and ref number # PFEA621219CR0720024) program funded by the European Maritime and Fisheries Fund (EMFF) and the Région Nouvelle-Aquitaine, France. M. Thomas is the recipient of a Make Our Planet Great Again PhD Fellowship (grant number # MOPGA-927921L) co-funded by Campus France with the Ministère de l'Europe et des Affaires étrangères, and E2S UPPA.
References
- G. Boeuf, C. R. Biol., 2011, 334, 435–440 CrossRef PubMed.
- G. S. Dhillon, S. Kaur, S. K. Brar and M. Verma, Crit. Rev. Biotechnol., 2013, 33, 379–403 CrossRef CAS PubMed.
- A. Steinbüchel, Curr. Opin. Biotechnol., 1992, 3, 291–297 CrossRef.
- M. Claverie, C. McReynolds, A. Petitpas, M. Thomas and S. C. M. Fernandes, Polymers, 2020, 12, 1002 CrossRef CAS PubMed.
- A. A. Shah, F. Hasan, A. Hameed and S. Ahmed, Biotechnol. Adv., 2008, 26, 246–265 CrossRef CAS PubMed.
- R. Geyer, J. R. Jambeck and K. L. Law, Sci. Adv., 2017, 3, e1700782 CrossRef PubMed.
-
M. Roser, Our World in Data, 2018.
- L. Lebreton, B. Slat, F. Ferrari, B. Sainte-Rose, J. Aitken, R. Marthouse, S. Hajbane, S. Cunsolo, A. Schwarz, A. Levivier, K. Noble, P. Debeljak, H. Maral, R. Schoeneich-Argent, R. Brambini and J. Reisser, Sci. Rep., 2018, 8, 4666 CrossRef CAS PubMed.
-
J. A. Glaser, in Plastics in the Environment, ed. A. Gomiero, IntechOpen, 2019 Search PubMed.
- J.-D. Gu, Int. Biodeterior. Biodegrad., 2003, 52, 69–91 CrossRef CAS.
- K. Mattsson, L.-A. Hansson and T. Cedervall, Environ. Sci.: Processes Impacts, 2015, 17, 1712–1721 RSC.
- M. Smith, D. C. Love, C. M. Rochman and R. A. Neff, Curr. Environ. Health Rep., 2018, 5, 375–386 CrossRef CAS PubMed.
- C. J. Thiele, M. D. Hudson, A. E. Russell, M. Saluveer and G. Sidaoui-Haddad, Sci. Rep., 2021, 11, 2045 CrossRef CAS PubMed.
- S. Reynaud, A. Aynard, B. Grassl and J. Gigault, Curr. Opin. Colloid Interface Sci., 2021, 101528 Search PubMed.
- R. Fernández-Marín, S. C. M. Fernandes, M. Á. A. Sánchez and J. Labidi, Food Hydrocolloids, 2022, 123, 107119 CrossRef.
- S. C. M. Fernandes, A. Alonso-Varona, T. Palomares, V. Zubillaga, J. Labidi and V. Bulone, ACS Appl. Mater. Interfaces, 2015, 7, 16558–16564 CrossRef CAS PubMed.
- A. M. Salaberria, R. H. Diaz, J. Labidi and S. C. M. Fernandes, React. Funct. Polym., 2015, 89, 31–39 CrossRef CAS.
- S. Fernandes, C. S. R. Freire, C. P. Neto and A. Gandini, Green Chem., 2008, 10, 93–97 RSC.
- S. C. M. Fernandes, C. S. R. Freire, A. J. D. Silvestre, J. Desbrières, A. Gandini and C. P. Neto, Ind. Eng. Chem. Res., 2010, 49, 6432–6438 CrossRef CAS.
- V. K. Thakur and S. I. Voicu, Carbohydr. Polym., 2016, 146, 148–165 CrossRef CAS PubMed.
- R. Ylitalo, S. Lehtinen, E. Wuolijoki, P. Ylitalo and T. Lehtimäki, Arzneimittelforschung, 2011, 52, 1–7 CrossRef PubMed.
-
A. Muxika, I. Zugasti, P. Guerrero and K. de la Caba, in Reference Module in Food Science, Elsevier, 2017, p. B9780081005965224000 Search PubMed.
- R. Sharif, M. Mujtaba, M. Ur Rahman, A. Shalmani, H. Ahmad, T. Anwar, D. Tianchan and X. Wang, Molecules, 2018, 23, 872 CrossRef PubMed.
- S. C. M. Fernandes, C. S. R. Freire, A. J. D. Silvestre, C. P. Neto, A. Gandini, J. Desbriéres, S. Blanc, R. A. S. Ferreira and L. D. Carlos, Carbohydr. Polym., 2009, 78, 760–766 CrossRef CAS.
- I. Aranaz, N. Acosta, C. Civera, B. Elorza, J. Mingo, C. Castro, M. Gandía and A. Heras Caballero, Polymers, 2018, 10, 213 CrossRef PubMed.
- S. M. Ahsan, M. Thomas, K. K. Reddy, S. G. Sooraparaju, A. Asthana and I. Bhatnagar, Int. J. Biol. Macromol., 2018, 110, 97–109 CrossRef CAS PubMed.
- M. Yadav, P. Goswami, K. Paritosh, M. Kumar, N. Pareek and V. Vivekanand, Bioresour. Bioprocess., 2019, 6, 8 CrossRef.
- N. Morin-Crini, E. Lichtfouse, G. Torri and G. Crini, Environ. Chem. Lett., 2019, 17, 1667–1692 CrossRef CAS.
- A. Nagarajan, A. Shanmugam and A. Zackaria, J. Algal Biomass Util., 2016, 7, 45–55 Search PubMed.
- A. Dalmoro, A. A. Barba, G. Lamberti, M. Grassi and M. d'Amore, Adv. Polym. Technol., 2012, 31, 219–230 CrossRef CAS.
- H. H. Tønnesen and J. Karlsen, Drug Dev. Ind. Pharm., 2002, 28, 621–630 CrossRef PubMed.
- R. Pereira, A. Carvalho, D. C. Vaz, M. H. Gil, A. Mendes and P. Bártolo, Int. J. Biol. Macromol., 2013, 52, 221–230 CrossRef CAS PubMed.
- E. Alsberg, K. W. Anderson, A. Albeiruti, R. T. Franceschi and D. J. Mooney, J. Dent. Res., 2001, 80, 2025–2029 CrossRef CAS PubMed.
-
G. Kogan, L. Šoltés, R. Stern, J. Schiller and R. Mendichi, in Studies in Natural Products Chemistry, Elsevier, 2008, vol. 34, pp. 789–882 Search PubMed.
- A. Huynh and R. Priefer, Carbohydr. Res., 2020, 489, 107950 CrossRef CAS PubMed.
- C. Chircov, A. M. Grumezescu and L. E. Bejenaru, Rom. J. Morphol. Embryol., 2018, 59, 71–76 Search PubMed.
- N. J. Lowe, C. A. Maxwell, P. Lowe, M. G. Duickb and K. Shah, J. Am. Acad. Dermatol., 2001, 45, 930–933 CrossRef CAS PubMed.
- A. Sionkowska, B. Kaczmarek, M. Michalska, K. Lewandowska and S. Grabska, Pure Appl. Chem., 2017, 89, 1829–1839 CAS.
-
V. V. Menon, in Handbook of Marine Macroalgae, ed. S.-K. Kim, John Wiley & Sons, Ltd, Chichester, UK, 2011, pp. 541–555 Search PubMed.
- A. Mortensen, F. Aguilar, R. Crebelli, A. Di Domenico, M. J. Frutos, P. Galtier, D. Gott, U. Gundert-Remy, C. Lambré, J. Leblanc, O. Lindtner, P. Moldeus, P. Mosesso, A. Oskarsson, D. Parent–Massin, I. Stankovic, I. Waalkens–Berendsen, R. A. Woutersen, M. Wright, M. Younes, L. Brimer, P. Peters, J. Wiesner, A. Christodoulidou, F. Lodi, A. Tard and B. Dusemund, EFSA J., 2016, 14, 12 Search PubMed.
- M. Bonnet, J. C. Lagier, D. Raoult and S. Khelaifia, New Microbes New Infect., 2020, 34, 100622 CrossRef CAS PubMed.
- P. Y. Lee, J. Costumbrado, C.-Y. Hsu and Y. H. Kim, JoVE, 2012, 3923 Search PubMed.
- O. Coskun, North. Clin. Istanbul, 2016, 3(2), 156–160 Search PubMed.
- V. Rudovica, A. Rotter, S. P. Gaudêncio, L. Novoveská, F. Akgül, L. K. Akslen-Hoel, D. A. M. Alexandrino, O. Anne, L. Arbidans, M. Atanassova, M. Bełdowska, J. Bełdowski, A. Bhatnagar, O. Bikovens, V. Bisters, M. F. Carvalho, T. S. Catalá, A. Dubnika, A. Erdoğan, L. Ferrans, B. Z. Haznedaroglu, R. H. Setyobudi, B. Graca, I. Grinfelde, W. Hogland, E. Ioannou, Y. Jani, M. Kataržytė, S. Kikionis, K. Klun, J. Kotta, M. Kriipsalu, J. Labidi, L. Lukić Bilela, M. Martínez-Sanz, J. Oliveira, R. Ozola-Davidane, J. Pilecka-Ulcugaceva, K. Pospiskova, C. Rebours, V. Roussis, A. López-Rubio, I. Safarik, F. Schmieder, K. Stankevica, T. Tamm, D. Tasdemir, C. Torres, G. C. Varese, Z. Vincevica-Gaile, I. Zekker and J. Burlakovs, Front. Mar. Sci., 2021, 8, 723333 CrossRef.
- Y.-S. Lim, Y.-J. Ok, S.-Y. Hwang, J.-Y. Kwak and S. Yoon, Mar. Drugs, 2019, 17, 467 CrossRef CAS PubMed.
-
J. Rao, R. Pallela and G. Prakash, in Marine Cosmeceuticals, CRC Press, 2011, pp. 77–104 Search PubMed.
- E. Berteanu, D. Ionita, M. Simoiu, M. Paraschiv, R. Tatia, A. Apatean, M. Sidoroff and L. Tcacenco, Bull. Mater. Sci., 2016, 39, 377–383 CrossRef CAS.
- Mintel Companies, 2021.
-
A. Rudin and P. Choi, in The Elements of Polymer Science & Engineering, Elsevier, 2013, pp. 521–535 Search PubMed.
- J. Brizga, K. Hubacek and K. Feng, One Earth, 2020, 3, 45–53 CrossRef.
- S. R. Unger, T. A. Hottle, S. R. Hobbs, C. L. Thiel, N. Campion, M. M. Bilec and A. E. Landis, J. Health Serv. Res. Policy, 2017, 22, 218–225 CrossRef PubMed.
- N. J. Bennett, A. M. Cisneros-Montemayor, J. Blythe, J. J. Silver, G. Singh, N. Andrews, A. Calò, P. Christie, A. Di Franco, E. M. Finkbeiner, S. Gelcich, P. Guidetti, S. Harper, N. Hotte, J. N. Kittinger, P. Le Billon, J. Lister, R. López de la Lama, E. McKinley, J. Scholtens, A.-M. Solås, M. Sowman, N. Talloni-Álvarez, L. C. L. Teh, M. Voyer and U. R. Sumaila, Nat. Sustainable, 2019, 2, 991–993 CrossRef.
- D. Belhabib, P. Le Billon and N. J. Bennett, Nat. Hum. Behav., 2022, 6, 6–8 CrossRef PubMed.
- M. U. Sheth, S. K. Kwartler, E. R. Schmaltz, S. M. Hoskinson, E. J. Martz, M. M. Dunphy-Daly, T. F. Schultz, A. J. Read, W. C. Eward and J. A. Somarelli, Front. Mar. Sci., 2019, 6, 624 CrossRef.
- H. Sun, L. Gao, C. Xue and X. Mao, Compr. Rev. Food Sci. Food Saf., 2020, 19, 2767–2796 CrossRef CAS PubMed.
- G. Wang, D. Huang, J. Ji, C. Völker and F. R. Wurm, Adv. Sci., 2021, 8, 2001121 CrossRef CAS PubMed.
-
P. Sharma, P. S. Slathia, N. Raina and D. Bhagat, in Freshwater Microbiology, Elsevier, 2019, pp. 341–392 Search PubMed.
- J. Klier, O. Dellwig, T. Leipe, K. Jürgens and D. P. R. Herlemann, Front. Microbiol., 2018, 9, 236 CrossRef PubMed.
-
E. D. Enger and B. F. Smith, Environmental science: a study of interrelationships, McGraw-Hill Education, New York, NY, Fifteenth edition, 2019 Search PubMed.
- S. Chakraborty, A. Khopade, C. Kokare, K. Mahadik and B. Chopade, J. Mol. Catal. B: Enzym., 2009, 58, 17–23 CrossRef CAS.
- Y. Ohta, Y. Hatada, M. Miyazaki, Y. Nogi, S. Ito and K. Horikoshi, Curr. Microbiol., 2005, 50, 212–216 CrossRef CAS PubMed.
-
P. S. Meadows and J. I. Campbell, An Introduction to Marine Science, Springer Netherlands, Dordrecht, 1988 Search PubMed.
- A. Boetius, Helgoländer Meeresuntersuchungen, 1995, 49, 177–187 CrossRef.
- E. Krause, A. Wichels, L. Giménez, M. Lunau, M. B. Schilhabel and G. Gerdts, PLoS One, 2012, 7, e47035 CrossRef CAS PubMed.
- S. Srimathi, G. Jayaraman, G. Feller, B. Danielsson and P. R. Narayanan, Extremophiles, 2007, 11, 505–515 CrossRef CAS PubMed.
- K. Mukai, K. Yamada and Y. Doi, Polym. Degrad. Stab., 1994, 43, 319–327 CrossRef CAS.
-
T. Ojima, in Marine Enzymes for Biocatalysis, Elsevier, 2013, pp. 333–371 Search PubMed.
-
N. R. Nair, V. C. Sekhar, K. M. Nampoothiri and A. Pandey, in Current Developments in Biotechnology and Bioengineering, Elsevier, 2017, pp. 739–755 Search PubMed.
- T. Miyamoto, S. Takahashi, H. Ito, H. Inagaki and Y. Noishiki, J. Biomed. Mater. Res., 1989, 23, 125–133 CrossRef CAS PubMed.
- W. Liu, Y. Ma, L. Ai, W. Li, W. Li, H. Li, C. Zhou and B. Luo, ACS Biomater. Sci. Eng., 2019, 5, 5316–5326 CrossRef CAS PubMed.
- T. G. Volova, A. N. Boyandin, A. D. Vasiliev, V. A. Karpov, S. V. Prudnikova, O. V. Mishukova, U. A. Boyarskikh, M. L. Filipenko, V. P. Rudnev, B. Bá Xuân, V. Viêt Dũng and I. I. Gitelson, Polym. Degrad. Stab., 2010, 95, 2350–2359 CrossRef CAS.
- J. P. Harrison, M. Sapp, M. Schratzberger and A. M. Osborn, Mar. Technol. Soc. J., 2011, 45, 12–20 CrossRef.
- N. Mohanan, Z. Montazer, P. K. Sharma and D. B. Levin, Front. Microbiol., 2020, 11, 580709 CrossRef PubMed.
- R. Osawa and T. Koga, Lett. Appl. Microbiol., 1995, 21, 288–291 CrossRef CAS.
- H. Yagi, A. Fujise, N. Itabashi and T. Ohshiro, Biosci., Biotechnol., Biochem., 2016, 80, 2338–2346 CrossRef CAS PubMed.
- Y. Zhu, L. Wu, Y. Chen, H. Ni, A. Xiao and H. Cai, Microbiol. Res., 2016, 182, 49–58 CrossRef CAS PubMed.
- J.-P. Chessa, G. Feller and C. Gerday, Can. J. Microbiol., 1999, 45, 452–457 CrossRef CAS PubMed.
- D. R. Humphry, A. George, G. W. Black and S. P. Cummings, Int. J. Syst. Evol. Microbiol., 2001, 51, 1235–1243 CrossRef CAS PubMed.
- R. Salas-Ovilla, D. Gálvez-López, A. Vázquez-Ovando, M. Salvador-Figueroa and R. Rosas-Quijano, PeerJ, 2019, 7, e6102 CrossRef PubMed.
- M. Pasqualetti, P. Barghini, V. Giovannini and M. Fenice, Molecules, 2019, 24, 1880 CrossRef CAS PubMed.
- V. de M. Oliveira, C. R. D. Assis, P. N. Herculano, M. T. H. Cavalcanti, R. de S. Bezerra and A. L. F. Porto, Bol. Inst. Pesca, 2017, 43, 52–64 CrossRef CAS.
- M. Gautam and W. Azmi, J. Appl. Biotechnol. Rep., 2017, 4, 558–565 CAS.
- H. Kazunori, M. Taku, Y. Michio, T. Masaharu, T. Hikoji, M. Tetsuo and S. Jiro, Agric. Biol. Chem., 1971, 35, 1651–1659 CrossRef.
- Y. Sugano, I. Terada, M. Arita, M. Noma and T. Matsumoto, Appl. Environ. Microbiol., 1993, 59, 1549–1554 CrossRef CAS PubMed.
- M. Duckworth and J. R. Turvey, Biochem. J., 1969, 113, 139–142 CrossRef CAS PubMed.
- P. Potin, C. Richard, C. Rochas and B. Kloareg, Eur. J. Biochem., 1993, 214, 599–607 CrossRef CAS PubMed.
- T. Araki, M. Hayakawa, Z. Lu, S. Karita and T. Morishita, J. Mar. Biotechnol., 1998, 6, 260–265 Search PubMed.
- Z. Hu, B.-K. Lin, Y. Xu, M. Q. Zhong and G.-M. Liu, J. Appl. Microbiol., 2009, 106, 181–190 CrossRef CAS PubMed.
- J. Wang, H. Mou, X. Jiang and H. Guan, Appl. Microbiol. Biotechnol., 2006, 71, 833–839 CrossRef CAS PubMed.
- J. Vera, R. Alvarez, E. Murano, J. C. Slebe and O. Leon, Appl. Environ. Microbiol., 1998, 64, 4378–4383 CrossRef CAS PubMed.
- L. M. Morrice, M. W. McLEAN, F. B. Williamson and W. F. Long, Eur. J. Biochem., 1983, 135, 553–558 CrossRef CAS PubMed.
- T. Aoki, T. Araki and M. Kitamikado, Eur. J. Biochem., 1990, 187, 461–465 CrossRef CAS PubMed.
- X. T. Fu, H. Lin and S. M. Kim, Appl. Microbiol. Biotechnol., 2008, 78, 265–273 CrossRef CAS PubMed.
- H. J. van der Meulen and W. Harder, Antonie van Leeuwenhoek, 1975, 41, 431–447 CrossRef CAS PubMed.
- H. Li, Z. Chi, X. Wang and C. Ma, J. Ocean Univ. China, 2007, 6, 60–65 CrossRef CAS.
- A. Bhattacharya and B. I. Pletschke, Enzyme Microb. Technol., 2014, 55, 159–169 CrossRef CAS PubMed.
- F. Pérez-Pomares, V. Bautista, J. Ferrer, C. Pire, F. C. Marhuenda-Egea and M. J. Bonete, Extremophiles, 2003, 7, 299–306 CrossRef PubMed.
- X. Wang, G. Kan, X. Ren, G. Yu, C. Shi, Q. Xie, H. Wen and M. Betenbaugh, BioMed Res. Int., 2018, 2018, 1–16 Search PubMed.
- A. N. M. Ramli, M. A. Azhar, M. S. Shamsir, A. Rabu, A. M. A. Murad, N. M. Mahadi and R. Md. Illias, J. Mol. Model, 2013, 19, 3369–3383 CrossRef CAS PubMed.
- P. V. Vardhan Reddy, S. S. Shiva Nageswara Rao, M. S. Pratibha, B. Sailaja, B. Kavya, R. R. Manorama, S. M. Singh, T. N. Radha Srinivas and S. Shivaji, Res. Microbiol., 2009, 160, 538–546 CrossRef PubMed.
- A. Del-Cid, P. Ubilla, M.-C. Ravanal, E. Medina, I. Vaca, G. Levicán, J. Eyzaguirre and R. Chávez, Appl. Biochem. Biotechnol., 2014, 172, 524–532 CrossRef CAS PubMed.
- S. Elleuche, F. M. Qoura, U. Lorenz, T. Rehn, T. Brück and G. Antranikian, J. Mol. Catal. B: Enzym., 2015, 116, 70–77 CrossRef CAS.
- C. Bertoldo, F. Duffner, P. L. Jorgensen and G. Antranikian, Appl. Environ. Microbiol., 1999, 65, 2084–2091 CrossRef CAS PubMed.
- S. Violot, N. Aghajari, M. Czjzek, G. Feller, G. K. Sonan, P. Gouet, C. Gerday, R. Haser and V. Receveur-Bréchot, J. Mol. Biol., 2005, 348, 1211–1224 CrossRef CAS PubMed.
- C. Zhang and S.-K. Kim, Mar. Drugs, 2010, 8, 1920–1934 CrossRef CAS PubMed.
- V. M. Pathak and Navneet, Bioresour. Bioprocess., 2017, 4, 15 CrossRef.
- H. J. Hueck, Int. Biodeterior. Biodegrad., 2001, 48, 5–11 CrossRef CAS.
- J. H. Walsh, Int. Biodeterior. Biodegrad., 2001, 48, 16–25 CrossRef CAS.
- S. Wallström, E. Strömberg and S. Karlsson, Polym. Test., 2005, 24, 557–563 CrossRef.
- F. Cappitelli, P. Principi and C. Sorlini, Trends Biotechnol., 2006, 24, 350–354 CrossRef CAS PubMed.
- S. Bonhomme, A. Cuer, A.-M. Delort, J. Lemaire, M. Sancelme and G. Scott, Polym. Degrad. Stab., 2003, 81, 441–452 CrossRef CAS.
-
J.-P. Regnault, Microbiologie générale, Décarie, Vigot, Montréal; Paris (France), 1990 Search PubMed.
- C. A. Crispim and C. C. Gaylarde, Microb. Ecol., 2005, 49, 1–9 CrossRef CAS PubMed.
-
J. Pelmont, Biodégradations et métabolismes: les bactéries pour les technologies de l'environnement (Collection Grenoble sciences), EDP Sciences, 2005 Search PubMed.
- M. Shimao, Curr. Opin. Biotechnol., 2001, 12, 242–247 CrossRef CAS PubMed.
- A. Lugauskas, L. Levinskait and D. Pečiulyt, Int. Biodeterior. Biodegrad., 2003, 52, 233–242 CrossRef CAS.
- J. S. Webb, M. Nixon, I. M. Eastwood, M. Greenhalgh, G. D. Robson and P. S. Handley, Appl. Environ. Microbiol., 2000, 66, 3194–3200 CrossRef CAS PubMed.
- H. Tsuji and K. Suzuyoshi, Polym. Degrad. Stab., 2002, 75, 347–355 CrossRef CAS.
- N. Lucas, C. Bienaime, C. Belloy, M. Queneudec, F. Silvestre and J.-E. Nava-Saucedo, Chemosphere, 2008, 73, 429–442 CrossRef CAS PubMed.
- Y. Li, S. Y. Park and J. Zhu, Renewable Sustainable Energy Rev., 2011, 15, 821–826 CrossRef CAS.
-
Biological wastewater treatment: principles, modelling and design, ed. M. Henze, IWA Pub, London, 2008 Search PubMed.
- J.-B. Guillerme, C. Couteau and L. Coiffard, Cosmetics, 2017, 4, 35 CrossRef.
- D. Ozdil and H. M. Aydin, J. Chem. Technol. Biotechnol., 2014, 89, 1793–1810 CrossRef CAS.
- X. Hu, Y. Du, Y. Tang, Q. Wang, T. Feng, J. Yang and J. F. Kennedy, Carbohydr. Polym., 2007, 70, 451–458 CrossRef CAS.
- P. K. Dutta, M. N. V. Ravikumar and J. Dutta, J. Macromol. Sci., Part C: Polym. Rev., 2002, 42, 307–354 CrossRef.
- W. Herth, A. Kuppel and E. Schnepf, J. Cell Biol., 1977, 73, 311–321 CrossRef CAS PubMed.
- W. Herth, J. Ultrastruct. Res., 1979, 68, 16–27 CrossRef CAS PubMed.
- R. A. Smucker, Biochem. Syst. Ecol., 1991, 19, 357–369 CrossRef CAS.
- V. Zargar, M. Asghari and A. Dashti, ChemBioEng Rev., 2015, 2, 204–226 CrossRef.
- B. Bradić, U. Novak and B. Likozar, Green Process. Synth., 2019, 9, 13–25 Search PubMed.
- A. M. Salaberria, S. C. M. Fernandes, R. H. Diaz and J. Labidi, Carbohydr. Polym., 2015, 116, 286–291 CrossRef CAS PubMed.
- A. Labidi, A. M. Salaberria, S. C. M. Fernandes, J. Labidi and M. Abderrabba, J. Dispersion Sci. Technol., 2020, 41, 828–840 CrossRef CAS.
- A. Labidi, A. Salaberria, S. Fernandes, J. Labidi and M. Abderrabba, Materials, 2019, 12, 361 CrossRef CAS PubMed.
- A. Labidi, A. M. Salaberria, S. C. M. Fernandes, J. Labidi and M. Abderrabba, J. Taiwan Inst. Chem. Eng., 2016, 65, 140–148 CrossRef CAS.
- A. M. Salaberria, J. Labidi and S. C. M. Fernandes, Eur. Polym. J., 2015, 68, 503–515 CrossRef CAS.
- A. M. Salaberria, J. Labidi and S. C. M. Fernandes, Chem. Eng. J., 2014, 256, 356–364 CrossRef CAS.
- A. Salaberria, R. H. Diaz, M. Andrés, S. Fernandes and J. Labidi, Materials, 2017, 10, 546 CrossRef PubMed.
- A. M. Salaberría, R. Teruel-Juanes, J. D. Badia, S. C. M. Fernandes, V. Sáenz de Juano-Arbona, J. Labidi and A. Ribes-Greus, Compos. Sci. Technol., 2018, 167, 323–330 CrossRef.
- R. Fernández-Marín, J. Labidi, M. Á. Andrés and S. C. M. Fernandes, Polym. Degrad. Stab., 2020, 109227 CrossRef.
- V. Zubillaga, A. M. Salaberria, T. Palomares, A. Alonso-Varona, S. Kootala, J. Labidi and S. C. M. Fernandes, Biomacromolecules, 2018, 19, 3000–3012 CrossRef CAS PubMed.
- V. Zubillaga, A. Alonso-Varona, S. C. M. Fernandes, A. M. Salaberria and T. Palomares, Int. J. Mol. Sci., 2020, 21, 1004 CrossRef CAS PubMed.
-
Nitrogen-Containing Macromolecules in the Bio- and Geosphere, ed. B. A. Stankiewicz and P. F. van Bergen, American Chemical Society, Washington, DC, 1998, vol. 707 Search PubMed.
- L. Ralph Berger and D. M. Reynold, Biochim. Biophys. Acta, 1958, 29, 522–534 CrossRef.
-
Chitin in Nature and Technology, ed. R. Muzzarelli, C. Jeuniaux and G. W. Gooday, Springer US, Boston, MA, 1986 Search PubMed.
- S. Beier and S. Bertilsson, Front. Microbiol., 2013, 4, 149 CAS.
- Q. Yan and S. S. Fong, Bioresour. Bioprocess., 2015, 2, 31 CrossRef.
- A. Sørbotten, S. J. Horn, V. G. H. Eijsink and K. M. Vårum, FEBS J., 2005, 272, 538–549 CrossRef PubMed.
-
K.-E. L. Eriksson, R. A. Blanchette and P. Ander, Microbial and Enzymatic Degradation of Wood and Wood Components, Springer Berlin Heidelberg, Berlin, Heidelberg, 1990 Search PubMed.
- C. Ma, X. Li, K. Yang and S. Li, Mar. Drugs, 2020, 18, 126 CrossRef CAS PubMed.
- Y. Zhou, X. Chen, X. Li, Y. Han, Y. Wang, R. Yao and S. Li, Molecules, 2019, 24, 183 CrossRef PubMed.
- K. Y. Lee and D. J. Mooney, Prog. Polym. Sci., 2012, 37, 106–126 CrossRef CAS PubMed.
- I. A. Brownlee, A. Allen, J. P. Pearson, P. W. Dettmar, M. E. Havler, M. R. Atherton and E. Onsøyen, Crit. Rev. Food Sci. Nutr., 2005, 45, 497–510 CrossRef CAS PubMed.
- J.-C. Tang, H. Taniguchi, H. Chu, Q. Zhou and S. Nagata, Lett. Appl. Microbiol., 2009, 48, 38–43 CrossRef CAS PubMed.
-
K. Schaumann and G. Weide, in Thirteenth International Seaweed Symposium, ed. S. C. Lindstrom and P. W. Gabrielson, Springer Netherlands, Dordrecht, 1990, pp. 589–596 Search PubMed.
- M. Cardoso, R. Costa and J. Mano, Mar. Drugs, 2016, 14, 34 CrossRef PubMed.
- R. A. Joy, N. Vikkath and P. S. Ariyannur, Drug Metab. Pers. Ther., 2018, 33, 15–32 Search PubMed.
-
Y. Dongol, B. L. Dhananjaya, R. K. Shrestha and G. Aryal, in Pharmacology and Therapeutics, ed. S. J. T. Gowder, InTech, 2014 Search PubMed.
- J. Necas, L. Bartosikova, P. Brauner and J. Kolar, Vet. Med., 2008, 53, 397–411 CrossRef CAS.
- M. J. Jedrzejas, L. V. Mello, B. L. de Groot and S. Li, J. Biol. Chem., 2002, 277, 28287–28297 CrossRef CAS PubMed.
- W. Han, W. Wang, M. Zhao, K. Sugahara and F. Li, J. Biol. Chem., 2014, 289, 27886–27898 CrossRef CAS PubMed.
- S. H. Knutsen, D. E. Myslabodski, B. Larsen and A. I. Usov, Bot. Mar., 1994, 37(2), 163–170 CAS.
- W.-J. Chi, Y.-K. Chang and S.-K. Hong, Appl. Microbiol. Biotechnol., 2012, 94, 917–930 CrossRef CAS PubMed.
- G. H. Kwon, M. J. Kwon, J. E. Park and Y. H. Kim, Biotechnol. Rep., 2019, 23, e00346 CrossRef PubMed.
-
H. P. Wiesmann, U. Meyer, U. Plate and H. J. Höhling, in International Review of Cytology, Elsevier, 2004, vol. 242, pp. 121–156 Search PubMed.
- R. G. Paul and A. J. Bailey, Sci. World J., 2003, 3, 138–155 CrossRef CAS PubMed.
- T. Silva, J. Moreira-Silva, A. Marques, A. Domingues, Y. Bayon and R. Reis, Mar. Drugs, 2014, 12, 5881–5901 CrossRef CAS PubMed.
-
W. Wagermaier and P. Fratzl, in Polymer science: A comprehensive Reference, Elvesier, 2012, pp. 35–55 Search PubMed.
- A. Levasseur, E. Drula, V. Lombard, P. M. Coutinho and B. Henrissat, Biotechnol. Biofuels, 2013, 6, 41 CrossRef CAS PubMed.
- J. Karlsson, M. Saloheimo, M. Siika-aho, M. Tenkanen, M. Penttilä and F. Tjerneld, Eur. J. Biochem., 2001, 268, 6498–6507 CrossRef CAS PubMed.
- S. Karkehabadi, H. Hansson, S. Kim, K. Piens, C. Mitchinson and M. Sandgren, J. Mol. Biol., 2008, 383, 144–154 CrossRef CAS PubMed.
- W. T. Beeson, V. V. Vu, E. A. Span, C. M. Phillips and M. A. Marletta, Annu. Rev. Biochem., 2015, 84, 923–946 CrossRef CAS PubMed.
- R. J. Quinlan, M. D. Sweeney, L. Lo Leggio, H. Otten, J.-C. N. Poulsen, K. S. Johansen, K. B. R. M. Krogh, C. I. Jorgensen, M. Tovborg, A. Anthonsen, T. Tryfona, C. P. Walter, P. Dupree, F. Xu, G. J. Davies and P. H. Walton, Proc. Natl. Acad. Sci. U. S. A., 2011, 108, 15079–15084 CrossRef CAS PubMed.
- Z. Forsberg, M. Sørlie, D. Petrović, G. Courtade, F. L. Aachmann, G. Vaaje-Kolstad, B. Bissaro, Å. K. Røhr and V. G. Eijsink, Curr. Opin. Struct. Biol., 2019, 59, 54–64 CrossRef CAS PubMed.
- B. Bissaro, Å. K. Røhr, G. Müller, P. Chylenski, M. Skaugen, Z. Forsberg, S. J. Horn, G. Vaaje-Kolstad and V. G. H. Eijsink, Nat. Chem. Biol., 2017, 13, 1123–1128 CrossRef CAS PubMed.
- G. Vaaje-Kolstad, B. Westereng, S. J. Horn, Z. Liu, H. Zhai, M. Sørlie and V. G. H. Eijsink, Science, 2010, 330, 219–222 CrossRef CAS PubMed.
- S. Kuusk, R. Kont, P. Kuusk, A. Heering, M. Sørlie, B. Bissaro, V. G. H. Eijsink and P. Väljamäe, J. Biol. Chem., 2019, 294, 1516–1528 CrossRef CAS PubMed.
- C. A. Fowler, F. Sabbadin, L. Ciano, G. R. Hemsworth, L. Elias, N. Bruce, S. McQueen-Mason, G. J. Davies and P. H. Walton, Biotechnol. Biofuels, 2019, 12, 232 CrossRef PubMed.
- H. Brandl and P. Püchner, Biodegradation, 1992, 2, 237–243 CrossRef.
- S. H. Imam, J. M. Gould, S. H. Gordon, M. P. Kinney, A. M. Ramsey and T. R. Tosteson, Curr. Microbiol., 1992, 25, 1–8 CrossRef CAS.
- M. Rutkowska, A. Heimowska, K. Krasowska and H. Zofia Janik, Pol. J. Environ. Stud., 2002, 11, 267–274 CAS.
- N.-C. Pauli, J. S. Petermann, C. Lott and M. Weber, R. Soc. Open Sci., 2017, 4, 170549 CrossRef PubMed.
- H. Tsuji and K. Suzuyoshi, Polym. Degrad. Stab., 2002, 75, 357–365 CrossRef CAS.
- A. Le Duigou, P. Davies and C. Baley, Polym. Degrad. Stab., 2009, 94, 1151–1162 CrossRef CAS.
- R. Priyadarshi and J.-W. Rhim, Innovative Food Sci. Emerging Technol., 2020, 62, 102346 CrossRef CAS.
- T. NAKASHIMA, Y. NAKANO, Y. BIN and M. MATSUO, J. Home Econ. Jpn., 2005, 56, 889–897 CAS.
- I. Makarios-Laham and T.-C. Lee, J. Environ. Polym. Degrad., 1995, 3, 31–36 CrossRef CAS.
- A. M. Baty, C. C. Eastburn, Z. Diwu, S. Techkarnjanaruk, A. E. Goodman and G. G. Geesey, Appl. Environ. Microbiol., 2000, 66, 3566–3573 CrossRef CAS PubMed.
- T. Ikejima and Y. Inoue, Carbohydr. Polym., 2000, 41, 351–356 CrossRef CAS.
Footnotes |
† Electronic supplementary information (ESI) available. See DOI: 10.1039/d1gc04327g |
‡ These authors contributed equally to this work. |
§ Present address: Department for Food Chemistry and Toxicology, University of Vienna, Vienna 1090, Austria. |
|
This journal is © The Royal Society of Chemistry 2022 |
Click here to see how this site uses Cookies. View our privacy policy here.