DOI:
10.1039/D2FO02598A
(Paper)
Food Funct., 2022,
13, 11811-11824
Curcumin alleviates LPS-induced intestinal homeostatic imbalance through reshaping gut microbiota structure and regulating group 3 innate lymphoid cells in chickens†
Received
3rd September 2022
, Accepted 17th October 2022
First published on 28th October 2022
Abstract
Gastrointestinal dysfunction is associated with a disturbance of immune homeostasis, changes in the intestinal microbiome, alteration of the composition of the bile acid pool, and dynamic imbalance of group 3 innate lymphoid cells (ILC3s). Curcumin (CUR), a polyphenolic compound isolated from turmeric, has been known to attenuate intestinal inflammation in potential therapies for gastrointestinal diseases. It was hypothesized that CUR could target the gut microbiome to modulate bile acid (BA) metabolism and the function of ILC3s in ameliorating lipopolysaccharide (LPS)-induced imbalance of intestinal homeostasis in chickens. Seven hundred and twenty 1-day-old crossbred chickens were randomly divided into four treatments, namely CON_saline (basal diet + saline control), CUR_saline (basal diet + 300 mg kg−1 curcumin + saline), CON_LPS (basal diet + LPS), and CUR_LPS (basal diet + 300 mg kg−1 curcumin + LPS), each consisting of 6 replicates of 30 birds. On days 14, 17, and 21, the chickens in the CON_LPS and CUR_LPS treatments were intraperitoneally injected with LPS at 0.5 mg per kg BW. Dietary CUR supplementation significantly decreased LPS-induced suppression of growth performance and injury to the intestinal tight junctions and decreased the vulnerability to LPS-induced acute inflammatory response by inhibiting pro-inflammatory (interleukin-1β and tumor necrosis factor-α) cytokines. CUR reshaped the cecal microbial community and BA metabolism, contributing to regulation of the intestinal mucosal immunity by promoting the anti-inflammatory (interleukin 10, IL-10) cytokines and enhancing the concentrations of primary and secondary BA metabolites (chenodexycholic acid, lithocholic acid). LPS decreased farnesoid X receptor (FXR) and G protein-coupled receptor class C group 5 member A synthesis, which was reversed by CUR administration, along with an increase in interleukin 22 (IL-22) production from ILC3s. Dietary supplementation of CUR increased the prevalence of Butyricicoccus and Enterococcus and enhanced the tricarboxylic acid cycle of intestinal epithelial cells. In addition, curcumin supplementation significantly increased sirtuin 1 and sirtuin 5 transcription and protein expression, which contributes to regulating mitochondrial metabolic and oxidative stress responses to alleviate LPS-induced enteritis. Our findings demonstrated that curcumin played a pivotal role in regulating the structure of the intestinal microbiome for health promotion and the treatment of intestinal dysbiosis. The beneficial effects of CUR may be attributed to the modulation of the BA-FXR pathway and inhibition of inflammation that induces IL-22 secretion by ILC3s in the intestinal lamina propria.
Introduction
The intestine of newly hatched chickens is susceptible to external stimuli, such as pathogenic bacterial infections, mycotoxins, and a hostile environment during their early growth phase. The gut microbiome is regarded as a virtual organ of the body, which protects the host against intestinal damage. It is apparent that gut microbial communities and their microbial metabolites substantially contribute to the interaction between the microbiota and mucosal immunity, leading to involvement in inflammatory bowel disease initiation and progression.1,2 Curcumin (CUR), an important natural polyphenol isolated from turmeric rhizomes (Curcuma longa), possesses immunomodulatory, anti-inflammatory, anti-bacterial, and anti-oxidative characteristics.3 Numerous studies have been conducted on the efficacy of CUR in poultry nutrition. Dietary supplementation of CUR at 150–300 mg kg−1 exhibited enhanced antioxidant capacity and improved immune responses in broiler chickens.4–6 It is increasingly acknowledged that curcumin's therapeutic potential might not solely depend on its bioavailability but that the beneficial effects might be attributable to its positive impact on gut health and barrier function.7–9 Interactions between CUR and microbiota are potentially crucial for curcumin's overall activity. The intestinal flora plays an indispensable role in the metabolism of bile acid (BA).10 In this regard, CUR and its metabolites have been shown to induce alterations in the gut microbiome and may influence BA pools, thus demonstrating therapeutic potential in inflammatory enteritis.
Group 3 innate lymphoid cells (ILC3s) are tissue-resident lymphocytes, associated with “barriers”, and exist predominantly in the gut lamina propria that are crucial in orchestrating immunity, inflammation, and microbiome equilibrium, and maintaining tissue homeostasis.11 Activation of ILC3s relies on glycolysis. Mitochondrial dysfunction and energy metabolism disturbance are strongly associated with ILC3 function and play a prominent role in metabolic and immune homeostasis.12,13 As a superfamily of nuclear receptors, the farnesoid X receptor (FXR) is expressed mainly within the gastrointestinal tract and liver. Liganded FXR is involved in regulating multiple genes participating in the metabolism of carbohydrates, fat, and bile acid (BA), thus governing cellular and systemic energy metabolism. The FXR mediates antioxidant and cytoprotective effects, closely linked to the capacity of the FXR system in improving mitochondrial function.14 Previous studies implicate FXR playing a role in gut barrier function and antibacterial defenses, with FXR activation inhibiting intestinal inflammation.
Sirtuins, which are essential metabolic sensors of energy status, are one of the preserved family of NAD+-dependent deacetylases. They can modulate the metabolic and inflammatory challenges from intestinal diseases.15 G-protein-coupled receptors (GPRs) contain a large number of various families of membrane proteins that sense and transduce extracellular signals into pivotal physiological effects.16 GPRs participate in several gastrointestinal functions, such as tissue repair, mucosal immunity, and nutrient digestion.17 The anti-microbial properties and aggregation of ILC3s in vivo are regulated by GPR183.18 GPRC6A deficiency reduced ILC3 generation and IL-22 synthesis in ILC3s, thus leading to high colon damage vulnerability, pathogen infection, and impaired mucosal healing.19 There is presently only a limited understanding of the regulation of mitochondria by intestinal microorganisms and their metabolites, the mechanism by which ILC3s modulate intestinal mucosal immune homeostasis, and curcumin-related FXR regulation and mechanisms of ILC3s.
Lipopolysaccharide (LPS), extracted from Gram-negative bacteria, is an effective bio-stimulant for the immune system. As a well-established mechanism, the LPS-induced intestinal inflammatory stress model has been widely used in in vivo studies.5 Previous studies have shown that LPS increased pro-inflammatory cytokine secretion and suppressed immunity, which might be due to natural or innate immune responses.20 Exploring the mechanisms of a series of bi-directional relationships between the intestinal microbiome and mitochondria will generate new theory regarding the protective response of CUR. Therefore, we speculated that CUR may alleviate LPS-induced inflammation via modulating the gut microbiota structure, and subsequent altering of BA signaling and regulation of ILC3s in the intestinal lamina propria. In the current study, crossbred broiler chickens were fed a diet supplemented with CUR for 21 days after LPS challenge to test the research hypothesis.
Materials and methods
Animals, treatments, and ethics statement
In total, 720 one-day-old 817 crossbred broiler chickens with similar hatchling weights were purchased from Guangdong Wenshi Southern Poultry Breeding Co., Ltd (Yunfu, China) and randomly divided into 4 dietary treatments with 6 replicates each (30 birds per replicate). All birds were reared in floor pens (length 3.5 m × width 1.5 m) with wood shaving litter (depth of 5 cm), given ad libitum access to feed and non-antibiotic tap water, and housed in an environmentally controlled room. All animal experimental procedures used in the research were performed under the approval of the Animal Care and Ethics Committee of the Institute of Animal Science, Guangdong Academy of Agriculture Sciences (no. 2021039).
Chickens were randomly allocated as a 2 × 2 factorial design; the main factors included diet [basal diet (control) or diet containing 300 mg kg−1 CUR] and challenge with LPS or sterile saline as a control. The 4 experimental treatments were (1) non-challenged chickens with no challenge, fed the basal diet and intraperitoneally (i.p.) administered NaCl solution (CON_saline); (2) non-challenged chickens fed the basal diet supplemented with 300 mg kg−1 CUR and i.p. administered NaCl solution (CUR_saline); (3) LPS-challenged chickens fed the basal diet and i.p. administered LPS (CON_LPS); and (4) LPS-challenged chickens fed the basal diet supplemented with 300 mg kg−1 CUR and i.p. administered LPS (CUR_LPS). The basal diet was a corn–wheat–soybean meal-type feed satisfying the nutritional requirements of the chickens, as recommended by Chinese standards (Table S1†). The optimum supplementation level of CUR was determined following the previous literature.4–6 LPS-challenged chickens received an i.p. injection of 0.5 mg per kg BW Escherichia coli LPS at 8:00 am on days 14, 17, and 21, and the non-challenged birds were i.p. injected with the same volume of sterile saline. The dose of injected LPS was based on previous studies.21,22 LPS from Escherichia coli 055:B5 (CAS: L4005, >99% purity) was obtained from Sigma-Aldrich Trading Co., Ltd (Shanghai, China). Curcumin, from Curcuma longa, was kindly provided by Cohoo Biotech Co., Ltd (Guangzhou, China) with a purity of more than 98%. The dose of CUR was chosen in accordance with Li et al.6
Sample collection and preparation
Three hours following LPS challenge at 21 d of age, 2 chickens per replicate (12 chickens per treatment) were randomly selected, electrically stunned and subsequently euthanized by exsanguination. Samples of the mid-portions of the jejunum and ileum were harvested and stored at −80 °C for the determination of intestinal indices and measurement of cytokine levels. Samples of the distal-third portions of the ileum without the lymph gland were harvested as above for immediate measurement of the subpopulation of intestinal lamina propria lymphocytes (LPL). Cecal digesta were collected in sterile EP tubes and stored at −80 °C for further analysis.
Growth performance parameters
During the experimental period of 21 d, birds were weighed by pen and feed consumption was recorded on a per replicate basis. The average daily gain (ADG), average daily feed intake (ADFI), and feed conversion ratio (FCR; feed
:
gain, g
:
g) were determined accordingly. Mortality was recorded daily and the mortality rate was calculated.
Flow cytometry analysis of lamina propria lymphocyte subpopulations
The ileal LPLs were isolated and purified and lymphocyte subpopulations were determined as described previously.23,24 Briefly, the isolated lymphocytes were stained using anti-chicken CD3-FITC, CD4-FITC, CD8a-PE, and BU-1-FITC from Southern Biotech Associates (Birmingham, AL) and incubated for 30 min at 37 °C, then washed with PBS twice. A FACSCalibur flow cytometer (Becton Dickinson, Franklin Lakes, NJ) was used to measure the proportions of CD3+, CD4+, CD8+ and B cells. Gates were drawn around cells and a 2-parameter dot-plot histogram was used to analyze the fluorescence data collected on at least 50
000 lymphocytes. Cell phenotypic data are expressed as a percentage of gated lymphocytes.
Quantitative real-time PCR
Total RNA extraction from the frozen jejunum and ileum followed by reverse transcription and quantitative real-time PCR was conducted according to previously described procedure.25 The used primers were synthesized and purchased from Sangong Biological Engineering Co., Ltd (Guangzhou, China) and are listed in the ESI, Table S2.† The transcription levels of the intestinal genes were calculated using the 2−ΔΔCt method. β-Actin was used as an internal control for the normalization of target gene expression.
Western blot analysis
Western blot analysis was performed as described previously.26 The antibodies used included OCLN (1
:
1000, Bioss Biotechnology, Beijing, China), FXR (1
:
1000, Cell Signaling Technology, Danvers, MA), SIRT1 (1
:
1000, Cell Signaling Technology), SIRT5 (1
:
800, Cell Signaling Technology), and GAPDH (1
:
1000, Cell Signaling Technology). The immunoreactive bands were detected by the FluorChem M fluorescence imaging system (ProteinSimple, Santa Clara, CA). The band densities were normalized against GAPDH and quantified by densitometry using the Image J software.
Enzyme-linked immunosorbent assay
Ileal concentrations of IL-1β, IL-6, TNF-α, IL-10, IL-22 and TGF-β were detected using commercial chicken-specific ELISA kits (Beijing Fangcheng Biotechnology Co., Ltd, Beijing, China) as previously described by Fan et al.22 The intra-assay coefficient of variation was below 8% and inter-assay coefficients of variation were below 10% for the above cytokines.
16S rRNA sequencing analysis
Total genomic DNA was harvested from each sample of cecal digesta using the Mag-Bind Soil DNA kit (Omega, Norcross, GA). The purity and concentration of all DNA samples were assessed using a Nanodrop ND-2000 (Thermo Fisher Scientific, Waltham, MA). Amplification of the bacterial 16S rRNA gene covering the V3–V4 region was carried out using the universal 338F-806R primers. The amplicons of PCR products were purified using the Qiagen gel extraction kit (Qiagen, Santa Clarita, CA) and further sequenced on an Illumina MiSeq platform at Majorbio Bio-Pharm Technology Co., Ltd (Shanghai, China). The bioinformatics analysis was conducted as described previously.27
GC-MS based metabolomics study
The metabolomes of dried cecal content samples were analyzed with 6 biological replicates for each treatment. Each sample of 50 mg powdered cecal contents was extracted according to the steps previously mentioned.28 The peak integration, alignment, deconvolution, and raw GC-MS data processing were performed according to the protocol.29 Multivariate statistical analyses including unsupervised principal component analysis (PCA) and supervised orthogonal partial least-squares discriminant analysis (OPLS-DA) were carried out using the SIMCA-P software (Version 13.0, Umetrics AB, Umeå, Sweden) after normalizing to an internal standard. The discriminant metabolites between the two comparison treatments were defined as VIP > 0.5 and |p(corr)| > 0.5.
Quantification of bile acids by LC-MS/MS
Targeted LC-MS/MS profiling of bile acids in chicken cecal digesta was conducted as previously described with some modifications.30 The concentrations of chenodexycholic acid (CDCA), deoxycholic acid (DCA), lithocholic acid (LCA), and cholic acid (CA) were determined in negative ion multiple reaction monitoring (MRM) detection mode under the optimized MS/MS conditions (Table S3†).
Statistical analysis
All data were statistically analyzed by ANOVA using the GLM procedures of SAS 9.4 (SAS Institute Inc., Cary, NC) with factors of diet type and LPS challenge and their interaction. When the interaction was observed to be significant, Duncan's multiple comparison tests were applied to evaluate the differences among treatments. A probability value of P < 0.05 was considered statistically significant, and 0.05 < P < 0.10 was defined as a tendency towards significance. Partial least squares discriminant analysis (PLS-DA) was carried out by the mixOmics R package to reveal the microbiota variation among treatments. The co-occurrence relationships between gut metabolites, gut microbiota, inflammatory cytokines, and gene expression were constructed based on Pearson's correlation coefficients, and features were considered significant at P < 0.05.
Results
Growth performance
As shown in Table 1, ADG and 21 d BW showed decreasing tendencies on LPS treatment (P = 0.089 and P = 0.091), whereas CUR significantly increased ADG and 21 d BW and decreased FCR (P < 0.05), compared with the CON. A trend for CUR × LPS interaction was observed for ADFI, with CUR increasing ADFI in the presence of LPS challenge (P = 0.074). No significant differences in mortality rates were observed among the treatments (P > 0.05).
Table 1 Effects of curcumin (CUR) supplementation on growth performance of LPS challenged chickens
Items |
Saline |
LPS |
SEM |
P-Value |
CON |
CUR |
CON |
CUR |
CUR |
LPS |
CUR × LPS |
Values are mean and pooled SEM, n = 6. Means with no common superscript in the same row are significantly different (P < 0.05). |
1 d BW (g) |
39.49 |
39.45 |
39.55 |
39.39 |
0.049 |
0.153 |
1.000 |
0.194 |
21 d BW (g) |
404.07 |
426.38 |
382.06 |
424.47 |
6.742 |
<0.001 |
0.091 |
0.152 |
ADFI (g) |
27.74ab |
27.99a |
26.37b |
28.40a |
0.472 |
0.025 |
0.319 |
0.074 |
ADG (g d−1) |
17.36 |
18.43 |
16.31 |
18.34 |
0.321 |
<0.001 |
0.089 |
0.149 |
FCR (g : g) |
1.60 |
1.52 |
1.62 |
1.55 |
0.024 |
0.039 |
0.314 |
0.892 |
Mortality (%) |
2.00 |
0.67 |
4.00 |
1.33 |
1.238 |
0.292 |
0.298 |
0.599 |
T-Lymphocyte phenotypic and B cells analysis
The results of the flow cytometry analysis of lamina propria lymphocyte subpopulations are shown in Table S4.† A significant interaction between CUR and LPS was observed for CD3+, CD4+, CD8+, and BU_1+ proportions in the ileum (P < 0.05). Chickens supplemented with CUR showed an increased CD3+, CD4+, CD8+ ratio compared to chickens fed with the CON diet (P < 0.05); with LPS they showed a decreased CD3+, CD4+, CD8+ ratio and increased BU_1+ ratio (P < 0.05) (Table S4†).
Ileal concentrations of inflammatory cytokines
In Table 2, LPS significantly increased ileal IL-1β and TNF-α concentrations but decreased ileal concentrations of IL-10 and IL-22 (P < 0.05). No significant interaction between diet and LPS was detected for any variable in the ileum. CUR significantly decreased ileal concentrations of IL-1β and TNF-α (P < 0.05). There was a trend for CUR × LPS interaction in ileal TNF-α concentration (P = 0.061).
Table 2 Effects of curcumin supplementation on concentrations of inflammatory cytokines in ileum of LPS challenged chickens
Items |
Saline |
LPS |
SEM |
P-Value |
CON |
CUR |
CON |
CUR |
CUR |
LPS |
CUR × LPS |
Values are mean and pooled SEM, n = 12. Means with no common superscript in the same row are significantly different (P < 0.05). IL, interleukin; TNF-α, tumor necrosis factor-α; TGF-β, transforming growth tactor-β. |
IL-1β pg mg−1 prot |
22.13 |
19.23 |
31.60 |
23.98 |
1.205 |
<0.001 |
<0.001 |
0.532 |
IL-6 pg mg−1 prot |
22.4 |
22.2 |
25.2 |
22.3 |
1.344 |
0.256 |
0.266 |
0.320 |
IL-10 pg mg−1 prot |
42.79 |
41.81 |
33.33 |
39.89 |
2.285 |
0.234 |
0.021 |
0.115 |
IL-22 pg mg−1 prot |
153.3 |
159.2 |
124.9 |
148.1 |
7.798 |
0.029 |
0.020 |
0.283 |
TNF-α pg mg−1 prot |
51.42b |
46.73c |
64.66a |
55.34ab |
3.697 |
0.026 |
0.026 |
0.061 |
TGF-β pg mg−1 prot |
459.4 |
421.1 |
403.3 |
413.6 |
21.78 |
0.528 |
0.160 |
0.278 |
mRNA expression of tight junction and gut barrier-related genes in jejunum and ileum
There were no significant CUR × LPS interactions on jejunal and ileal transcripts of OCLN, CLDN1, ZO-1, and MUC2 (Fig. 1A and B). LPS downregulated the jejunal and ileal transcript abundance of OCLN compared to CON (P < 0.05). CUR upregulated the transcript abundance of OCLN and ZO-1 (P < 0.05).
 |
| Fig. 1 Effects of curcumin supplementation on the expression of tight junction protein genes in jejunum (A) and ileum (B) of chickens after LPS challenge. Values are mean and SE, n = 6. Means with no common superscript in the same column are significantly different (P < 0.05). CON_saline, chickens fed the control diet and injected with saline; CUR_saline, chickens fed the curcumin diet and injected with saline; CON_LPS, chickens fed the control diet and challenged with LPS; CUR_LPS, chickens fed the curcumin diet and challenged with LPS. OCLN, occludin; CLDN1, claudin 1; ZO-1, zona occludens 1; MUC2, mucin 2. | |
Gut microbiome
In total, 11
712 OTUs from 24 cecal samples (6 samples per treatment) were identified and each sample had 488 OTUs on average. Venn diagrams are shown in Fig. 2A. There were 40, 59, 29, and 39 unique OTUs identified in the CON_saline, CUR_saline, CON_LPS, and CUR_LPS treatments, respectively, and they shared 448 OTUs. Table 3 shows that supplementation with CUR resulted in significant reduction in alpha diversity indexes in the observed species and Shannon index (P < 0.05). There were no significant differences in alpha diversity including ACE, Chao, Simpson, and Good coverage among the four treatments (P > 0.05). Distinct clustering of microbiota in each treatment was exposed by PLS-DA (Fig. 2B). The relative abundances of the top 5 major phyla (Firmicutes, Bacteroidota, Proteobacteria, Actinobacteria, and others) are shown in Fig. 2C and Table 3. At the phylum level, LPS decreased Firmicutes, Proteobacteria, and Firmicutes/Bacteroidota (P < 0.05), but tended to increase Bacteroidota (P = 0.053). Chickens fed diets supplemented with CUR showed increased Firmicutes abundance but decreased Bacteroidota abundance compared to the CON (P < 0.05). There was a CUR × LPS interaction observed for Proteobacteria (P < 0.05). The top 30 primary genera and their relative abundance among treatments are shown in Fig. 2D. There was a CUR × LPS interaction observed for Bacteroides (P < 0.05). Chickens after LPS administration showed reduced Enterococcus and increased Bacteroides, Alistipes, and unclassified_f_Ruminococcaceae (P < 0.05) abundance. Chickens fed the diet with CUR had lower unclassified_f_Ruminococcaceae, Alistipes, and higher Butyricicoccus and Enterococcus compared to chickens fed with the control diet (P < 0.05).
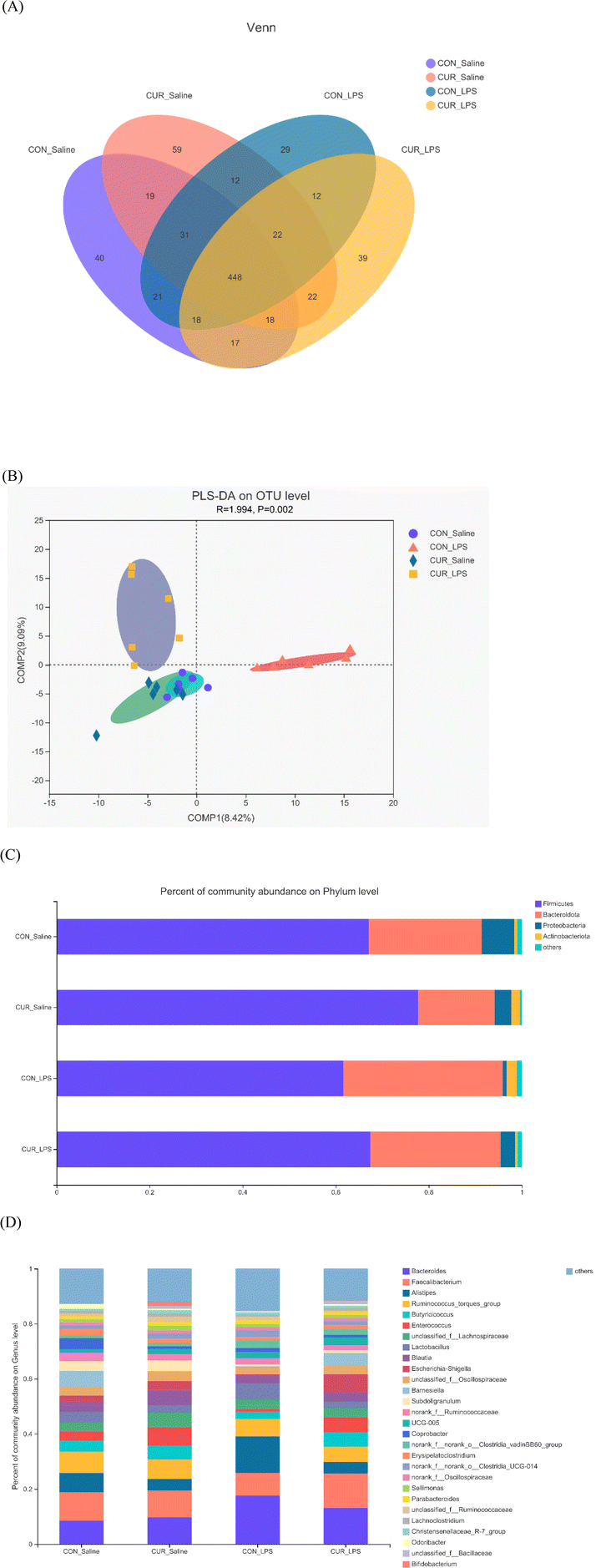 |
| Fig. 2 Effects of curcumin supplementation on the gut microbiota of chickens after LPS challenge. Values are mean and SE, n = 6. (A) Venn diagram depicting unique and shared OTU dietary groups. (B) Partial least squares discriminant analysis (PLS-DA) at the OTU level. (C) Stacked bar chart representing the relative abundance of colonic bacteria, at the phylum level (top 5) in the different dietary groups. (D) Stacked bar chart representing the relative abundance of colonic bacteria, at the genus level (top 30) in the different dietary groups. CON_saline, chickens fed the control diet and injected with saline; CUR_saline, chickens fed the curcumin diet and injected with saline; CON_LPS, chickens fed the control diet and challenged with LPS; CUR_LPS, chickens fed the curcumin diet and challenged with LPS. OTU, operational taxonomic unit. | |
Table 3 Effects of curcumin (CUR) supplementation on the α-diversity and relative abundance of intestinal bacteria in chickens after LPS challenge
Variable |
Saline |
LPS |
SEM |
P-Value |
CON |
CUR |
CON |
CUR |
CUR |
LPS |
CUR × LPS |
Values are mean and pooled SEM, n = 6. Means with no common superscript in the same row are significantly different (P < 0.05). CON_saline, chickens fed the control diet and injected with saline; CUR_saline, chickens fed the curcumin diet and injected with saline; CON_LPS, chickens fed the control diet and challenged with LPS; CUR_LPS, chickens fed the curcumin diet and challenged with LPS. |
α-Diversity index
|
Species observed |
388 |
339 |
395 |
347 |
22.3 |
0.043 |
0.733 |
0.991 |
Shannon |
3.94 |
3.52 |
3.89 |
3.85 |
0.137 |
0.041 |
0.483 |
0.310 |
Simpson |
0.05 |
0.08 |
0.05 |
0.05 |
0.007 |
0.324 |
0.243 |
0.318 |
Chao |
453 |
383 |
407 |
411 |
24.3 |
0.122 |
0.988 |
0.232 |
ACE |
442 |
379 |
406 |
411 |
23.5 |
0.164 |
0.716 |
0.307 |
Good's coverage |
0.99 |
0.99 |
0.99 |
0.99 |
<0.001 |
0.276 |
0.475 |
0.847 |
Relative abundance of total sequences at the phylum level
|
Firmicutes |
67.59 |
76.48 |
62.25 |
66.06 |
2.781 |
<0.001 |
0.010 |
0.516 |
Bacteroidota |
27.77 |
17.14 |
33.97 |
23.82 |
3.135 |
0.022 |
0.053 |
0.940 |
Firmicute/Bacteroidota |
2.43 |
4.46 |
1.74 |
2.77 |
0.526 |
<0.001 |
0.015 |
0.282 |
Proteobacteria |
8.39a |
4.19ab |
0.79b |
2.27ab |
1.025 |
0.454 |
<0.001 |
0.041 |
Relative abundance of total sequences at the genus level
|
Bacteroides
|
8.41b |
10.60ab |
17.93a |
13.15ab |
2.508 |
0.949 |
0.016 |
0.039 |
Alistipes
|
6.66 |
3.97 |
12.56 |
4.02 |
1.514 |
0.006 |
0.011 |
0.128 |
Butyricicoccus
|
3.03 |
6.88 |
2.18 |
5.43 |
0.829 |
0.005 |
0.256 |
0.467 |
Enterococcus
|
3.29 |
6.85 |
0.93 |
4.20 |
1.607 |
0.019 |
0.011 |
0.629 |
Subdoligranulum
|
3.60 |
3.90 |
0.76 |
1.19 |
0.911 |
0.751 |
0.025 |
0.958 |
Unclassified_f_Ruminococcaceae
|
0.85 |
0.46 |
1.87 |
1.23 |
0.303 |
0.029 |
0.022 |
0.517 |
Primary and secondary bile acid and metabolite concentrations in cecal digesta
The primary and secondary BA and metabolites related to energy metabolism in cecal contents were quantified by LC-MS/MS (Fig. 3 and 4). The primary BA cholic acid (CA), originating from conjugated endogenous BA by particular intestinal microbiota, was not changed by the treatment, while chickens challenged with LPS had significantly decreased concentrations of CDCA and LCA (P < 0.05), and dietary CUR supplementation significantly increased their levels (P < 0.05). Meanwhile, dietary supplementation of CUR led to higher levels of butyric, 3-hydroxybutyric, malic, and succinic acids in comparison with CON or LPS (P < 0.05).
 |
| Fig. 3 Effects of curcumin supplementation on bile acid concentrations in the cecum of chickens after LPS challenge. Values are mean and SE, n = 6. Means with different letters in the same column are significantly different (P < 0.05). CON_saline, chickens fed the control diet and injected with saline; CUR_saline, chickens fed the curcumin diet and injected with saline; CON_LPS, chickens fed the control diet and challenged with LPS; CUR_LPS, chickens fed the curcumin diet and challenged with LPS. CA, cholic acid; CDCA, chenodexycholic acid; DCA, deoxycholic acid; LCA, lithocholic acid. | |
 |
| Fig. 4 Relative contents of differential metabolites related to energy metabolism in cecal digesta of chickens. Values are mean and SE, n = 6. Means with different letters in the same column are significantly different (P < 0.05). CON_saline, chickens fed the control diet and injected with saline; CUR_saline, chickens fed the curcumin diet and injected with saline; CON_LPS, chickens fed the control diet and challenged with LPS; CUR_LPS, chickens fed the curcumin diet and challenged with LPS. | |
FXR, GPR and sirtuin transcripts and protein expression in the ileum
The transcript abundances of FXR and G protein-coupled receptors (GPRC5A, GPRC5B, GPRC5C, and GPRC6A) in the ileum were analyzed (Fig. 5 and 6). There were no significant CUR × LPS interactions on ileal transcripts of FXR or GPRs. LPS significantly decreased GPRC5A transcript abundance (P < 0.05). Dietary inclusion of CUR significantly increased ileal FXR mRNA (98.1%) and protein expression (67.3%) and GPRC5A and GPRC5B gene expression (150.3%, 42.2%; P < 0.05) in comparison with CON in the absence of the LPS challenge. LPS decreased ileal SIRT1, SIRT5 gene and protein expression (Fig. 7, P < 0.05). Diet supplemented with CUR dramatically increased SIRT1, SIRT5 gene and protein expression, compared to the CON diet (P < 0.05). There was a CUR × LPS interaction observed for SIRT5 mRNA expression (P < 0.05), and a trend for a CUR × LPS interaction was observed for SIRT5 protein expression in the ileum (P = 0.065).
 |
| Fig. 5 Effects of curcumin supplementation on the expression of SIRT1 to SIRT7 genes in ileum of chickens after LPS challenge. Values are mean and SE, n = 6. Means with different letters in the same column are significantly different (P < 0.05). CON_saline, chickens fed the control diet and injected with saline; CUR_saline, chickens fed the curcumin diet and injected with saline; CON_LPS, chickens fed the control diet and challenged with LPS; CUR_LPS, chickens fed the curcumin diet and challenged with LPS. SIRT1, sirtuin 1; SIRT2, sirtuin 2; SIRT3, sirtuin 3; SIRT4, sirtuin 4; SIRT5, sirtuin 5; SIRT6, sirtuin 6; SIRT7, sirtuin 7. | |
 |
| Fig. 6 Effects of curcumin supplementation on the expression of FXR and GPRs genes in the ileum of chickens after LPS challenge. Values are mean and SE, n = 6. Means with different letters in the same column are significantly different (P < 0.05). CON_saline, chickens fed the control diet and injected with saline; CUR_saline, chickens fed the curcumin diet and injected with saline; CON_LPS, chickens fed the control diet and challenged with LPS; CUR_LPS, chickens fed the curcumin diet and challenged with LPS. FXR, farnesoid X receptor; GPRC6A, G protein-coupled receptor class C group 6 member A; GPRC5A, G protein-coupled receptor class C group 5 member A; GPRC5B, G protein-coupled receptor class C group 5 member B; GPRC5C, G protein-coupled receptor class C group 5 member C. | |
 |
| Fig. 7 Effects of curcumin supplementation on protein expression of OCLN, FXR, SIRT1 and SIRT5. Values are mean and SE, n = 6. Means with no common superscript in the same column are significantly different (P < 0.05). (A) Western blot analysis of OCLN, ZO-1, FXR, SIRT1, and SIRT5 in ileum of chickens after LPS challenge. GAPDH was used as the loading control. (B) Mean ± SEM of immunoblotting bands of OCLN, FXR, SIRT1, and SIRT5 (n = 6). CON_saline, chickens fed the control diet and injected with saline; CUR_saline, chickens fed the curcumin diet and injected with saline; CON_LPS, chickens fed the control diet and challenged with LPS; CUR_LPS, chickens fed the curcumin diet and challenged with LPS. FXR, farnesoid X receptor; SIRT1, sirtuin 1; SIRT5, sirtuin 5. | |
Correlation analyses between intestinal metabolites, microbial communities, inflammatory cytokines, and intestinal gene expression
As shown in Fig. 8, Pearson's correlation test was then conducted to examine the association between key gene expression, inflammatory cytokine levels and the primary intestinal bacterial genera and metabolite abundances. Our findings indicate that SIRT1 transcript abundance was negatively correlated with Alistipes and unclassified_f_Ruminococcaceae relative abundance (P < 0.05), but positively correlated with concentrations of CDCA, LCA, malic acid, and butyric acid (P < 0.01). In addition, SIRT5 transcript abundance correlated negatively with Bacteroides and Alistipes relative abundances (P < 0.05), but correlated positively with Butyricicoccus (P < 0.05). There was a positive correlation between the transcript abundance of FXR and concentrations of 3-hydroxybutyric acid, and LCA, but there was a negative association between FXR transcript abundance and alanine concentration (P < 0.05). TNF-α concentration showed positive correlation with the relative abundances of Bacteroides and unclassified_f_Ruminococcaceae (P < 0.05). IL22 concentration was negatively correlated with the relative abundance of Alistipes and LCA concentration (P < 0.05). Moreover, ADG showed a positive correlation with the relative abundance of Enterococcus, LCA, and concentrations of malic and succinic acids (P < 0.01), and was negatively correlated with the relative abundance of Alistipes (P < 0.05).
 |
| Fig. 8 Heatmap of Pearson correlation coefficients between cecal metabolites, cecal microbiota, the relative abundance of genera, ADG, inflammatory cytokines, and intestinal gene expression level affected by CUR and LPS. *, **, *** Significant correlation: * P < 0.05, ** P < 0.01, *** P < 0.001. Red shading with P < 0.05 represents a significant negative correlation; blue shading with P < 0.05 represents a significant positive correlation; white shading represents no correlation, n = 6. ADG, average daily gain; CDCA, chenodexycholic acid; DCA, deoxycholic acid; LCA, lithocholic acid; FXR, farnesoid X receptor; GPRC5A, G protein-coupled receptor class C group 5 member A; SIRT1, sirtuin 1; SIRT3, sirtuin 3; SIRT5, sirtuin 5; IL10, interleukin 10; IL22, interleukin 22; TNF-α, tumor necrosis factor alpha. | |
Discussion
LPS i.p. injection is extensively used to establish an animal model of inflammation and bacterial infection.5 Excessive LPS can destroy the integrity of the intestinal mucosal barrier, permit bacterial translocation, and activate an inflammatory reaction, leading to a decrease in host growth and immune function.20 In our experiment, there was a trend observed in the CUR × LPS interaction for ADFI, with CUR increasing ADFI in chickens challenged with LPS. Compared with the CON diet, supplementation of CUR improved the growth performance of chickens and showed a greater ADG and 21d BW, and more efficient FCR. Dietary CUR supplementation attenuated the LPS-induced decrease in ADG. Previous studies using various birds and strains have provided consistent results that supplementation with curcumin enhances growth performance, likely because of CUR's promotion of nutrient utilization, antioxidative function and the immune status of broiler chickens.4,6 In addition, dietary supplementation with bisdemethoxycurcumin mitigated the negative impact of LPS on ADFI.5
Intestinal microbiota is a complex community of microorganisms that colonizes the gastrointestinal tract and contributes to the host health. In the present study, chickens challenged with LPS showed decreased Firmicutes and Proteobacteria relative abundances and Firmicutes/Bacteroidota ratio in cecal digesta, whereas CUR had the reverse effects. As a predominant bacteria in the gut, Firmicutes are more effective energetically than Bacteroidota in promoting the more efficient absorption of calories.31,32 Firmicutes are primary contributors to major short-chain fatty acid generation. Therefore, an enhanced population of Firmicutes results in improvement of the functions of the intestinal barrier and inhibition of inflammatory responses.33,34 At the genus level, LPS decreased the relative abundances of Enterococcus and Subdoligranulum and increased those of Bacteroides, Alistipes and unclassified_f_Ruminococcaceae, while CUR increased Enterococcus and decreased unclassified_f_Ruminococcaceae and Alistipes abundances. Alistipes, considered an opportunistic pathogen, is observed in diarrhea and ulcerative colitis.28,35 Enrichment of Bacteroides and unidentified-Ruminococcaceae is involved in the inflammation of colonic mucosa, leading to the destruction of the epithelial barrier function in the colon.36,37 Moreover, CUR increased Butyricicoccus abundance, in accordance with previous findings.38,39Butyricicoccus, a butyric acid-producing genus in the intestinal tract, decreases intestinal epithelial permeability with anti-inflammatory potential.40 These results suggest that CUR might alleviate LPS-induced inflammatory enteritis by regulating the intestinal microbiota.
ILC3s reside in intestinal lamina propria and are involved in maintaining intestinal homeostasis by orchestrating immunomodulation, tissue repair, and microorganisms balance.11 A limited encounter with a pathogen can promote durable phenotypic and functional changes in intestinal ILC3s, leading to improvements in mucosal defense.41 IL-22 is predominantly secreted by innate lymphoid cells, of which ILC3s are the primary source. The regeneration and reconstruction of the intestinal epithelium depend mainly on IL-22. In our experiment, chickens administered LPS showed decreased concentrations of CDCA and LCA in cecal digesta and decreased IL-22 production, while CUR substantially enhanced their levels after LPS challenge. Mice fed a diet that increased DCA in the digesta showed damaged ILC3s and suppressed IL-22 production in ileum.42 Inflammatory intestinal disease, involving diarrhea and ulcerative colitis, leads to increasing disruption of the mucosal barrier function, insulin resistance, altered BA metabolism, and reduced IL-22 secretion.43 Dysmetabolism of BA in inflammatory bowel diseases is characterized by the enrichment of primary and conjugated BAs and the reduction of secondary BAs such as DCA and LCA.44 The previous study showed that ILC3s in terminal ileal Peyer's patches were negatively regulated by microbiota-derived butyrate.45C. tyrobutyricum alleviated LPS-induced inflammation and colonic barrier malfunction through IL-22 signaling.46 In addition, there were alterations in the levels of energy metabolism-related metabolites. CUR supplementation increased butyric acid, 3-hydroxybutyric acid, malic acid, and succinic acid concentrations and decreased alanine. As the tricarboxylic acid cycle substrate, succinate generated by gut microorganisms expanded the tuft cells and reduced the inflammatory response in the ileum, increased IL22, IL25, and IL13 (cytokines: type 2) but suppressed IL23 (cytokines: type 17), which was linked to upregulating the expression of genes that control the tricarboxylic acid cycle.47 Previous research demonstrated that CUR could protect the mitochondrial membrane of animal intestinal epithelial cells from oxidative stress and mitochondrial dysfunction.25 It is worth noting that, in the small intestine and cecum, most of the dietary CUR may be hydrolyzed to generate acetate, butyrate, fumarate, lactate, pyruvate, and succinate.48 The effects of CUR noted here are perhaps related to its capacity to influence energy homeostasis and further influence the function of ILC3s.
FXR regulates multiple gene expression participating in carbohydrate, fat, and bile acid (BA) metabolism, thus governing cellular and systemic energy metabolism. FXR mediates antioxidant and cytoprotective effects, closely linked to the capacity of the FXR system to improve mitochondrial function.14 A growing number of studies demonstrate that FXR has essential roles in intestinal inflammation and the immune response.49 In the current study, our data showed that CUR supplementation increased the ileal gene and protein expression of FXR. GPRs participate in several gastrointestinal functions, such as tissue repair, mucosal immunity, and nutrient digestion.17 Secondary BAs can interact with mitochondria via FXR and GPRC5 to regulate lipid and carbohydrate metabolism.50In vivo, deficiency of GPR183 resulted in an imbalance in the mesenteric lymph nodes due to the redistribution of ILC3s combined with a reduction in ILC3s in the intestine.18 GPR43 regulates colonic ILC3 proliferation and function.51 Also, GPRC6A deficiency reduced ILC3 proliferation and IL-22 secretion by ILC3s, thus leading to high colon damage vulnerability, pathogen infection, and impaired mucosal healing.19 Interestingly, CUR increased the expression of the ileal GPRC5A and GPRC5B genes, but not that of GPRC6A. DCA and LCA are the two most potent ligands for GPR5, and a previous study revealed that CUR depends on GPR5 and gut microbiota to increase energy consumption.52 This may be related to the spatial structure difference of the GPRs.
Sirtuins, which are essential sensors of energy status, are one of the preserved family of NAD+-dependent deacetylases. SIRT3, SIRT4, and SIRT5 are mitochondrial sirtuins that control energy expenditure through regulation of mitochondrial enzyme activities.53 SIRT5 deficiency increases BA-induced immunosuppressive microenvironments in the liver.54 The present study demonstrated that LPS challenge reduced the expression of the ileal SIRT5 gene and protein, whereas CUR had the reverse effects. Previous research also demonstrated that CUR activated mitochondrial biogenesis to counteract LPS-induced mitochondrial dysfunction.55 Above all, it can be speculated that SIRT5 may also mediate the FXR signaling pathway to maintain energy homeostasis, in part through BAs. The correlation analysis showed that SIRT5 was significantly positively related to cecal Butyricicoccus abundance and succinic acid level, with a significant negative relationship with alanine. IL-22 concentrations were negatively correlated with Alistipes abundance and LCA level. In summary, the study demonstrates that giving chickens dietary CUR could effectively suppress inflammation and preserve the immune homeostasis of intestinal mucosa, partly through modulation of the BA-FXR pathway and the function of ILC3s.
Conclusions
In conclusion, the present results indicate that CUR could be used as a modulator of gut microbiota for intestinal health improvement and immunity homeostasis. The beneficial effects of CUR may be involved in the modulation of the BA-FXR pathway and inhibition of inflammation that induces IL-22 secretion by ILC3s in the intestinal lamina propria.
Author contributions
Dong Ruan: conceptualization, methodology, data curation, formal analysis, original draft writing, funding acquisition. Shaowen Wu: software, formal analysis, validation. Ahmed Mohamed Fouad: writing – review and editing. Yongwen Zhu: data curation, validation. Wenjie Huang: data curation, validation. Zhilong Chen: data curation, validation. Zhongyong Gou: data curation, validation. Yibing Wang: data curation, validation. Yongquan Han: supervision. Shijuan Yan: supervision. Chuntian Zheng: supervision, project administration. Shouqun Jiang: conceptualization, funding acquisition, methodology.
Conflicts of interest
The authors declare no competing interests.
Acknowledgements
We sincerely thank Prof. W. Bruce Currie (Cornell University) and Prof. Dayong Wu (Tufts University) for great assistance in the presentation of the manuscript. This study was supported by the Collaborative Innovation Center of GDAAS (XTXM 202205), Guangdong Natural Science Foundation (2019A1515010912), China Agricultural Research System of MOF and MARA (CARS-41), the National Key Research and Development Program (2021YFD1300404), the Key Realm R&D Program of Guangdong Province (2020B0202090004), the Supporting Program for the Research of State Key Laboratory of Livestock and Poultry Breeding, Key Laboratory of Animal Nutrition and Feed Science in South China, Ministry of Agriculture and Rural Affairs, Guangdong Key Laboratory of Animal Breeding and Nutrition, Scientific Innovation Strategy Construction of High Level Academy of Agriculture Science Fund (202106TD), and a Research Supporting Project (RSP-2022R439), King Saud University, Riyadh, Saudi Arabia.
References
- M. Rooks and W. S. Garrett, Gut microbiota, metabolites and host immunity, Nat. Rev. Immunol., 2016, 16, 341–352 CrossRef CAS PubMed.
- P. Gonçalves and J. Araújo, A cross-talk between microbiota-derived short-chain fatty acids and the host mucosal immune system regulates intestinal homeostasis and inflammatory bowel disease, Inflamm. Bowel Dis., 2018, 24, 558–572 CrossRef PubMed.
- Z. Hussain, H. E. Thu, M. W. Amjad, F. Hussain, T. A. Ahmed and S. Khan, Exploring recent developments to improve antioxidant, anti-inflammatory and antimicrobial efficacy of curcumin: A review of new trends and future perspectives, Mater. Sci. Eng., C, 2017, 77, 1316–1326 CrossRef CAS PubMed.
- S. Yadav, P. Teng, T. S. dos Santos, R. L. Gould, S. W. Craig, A. L. Fuller, R. Pazdro and W. K. Kim, The effects of different doses of curcumin compound on growth performance, antioxidant status, and gut health of broiler chickens challenged with Eimeria species, Poult. Sci., 2020, 99, 5936–5945 CrossRef CAS PubMed.
- J. Zhang, Y. Yang, H. Han and T. Wang, Bisdemethoxycurcumin attenuates lipopolysaccharide-induced intestinal damage through improving barrier integrity, suppressing inflammation, and modulating gut microbiota in broilers, J. Anim. Sci., 2021, 99, skab296 CrossRef PubMed.
- S. Li, R. Liu, S. Xia, G. Wei, M. Ishfaq, Y. Zhang and X. Zhang, Protective role of curcumin on aflatoxin B1-induced TLR4/RIPK pathway mediated-necroptosis and inflammation in chicken liver, Ecotoxicol. Environ. Saf., 2022, 233, 113319 CrossRef CAS PubMed.
- A. L. Lopresti, The problem of curcumin and its bioavilability: Could its gastrointestinal influence contribute to its overall health-enhancing effects?, Adv. Nutr., 2018, 9, 41–50 CrossRef PubMed.
- W. Zam, Gut microbiota as a prospective therapeutic target for curcumin: A review of mutual influence, J. Nutr. Metab., 2018, 2018, 1367984 Search PubMed.
- B. Scazzocchio, L. Minghetti and M. D'Archivio, Interaction between gut microbiota and curcumin: A new key of understanding for the health effects of curcumin, Nutrient, 2020, 12, 2499 CrossRef CAS PubMed.
- J. A. Winston and C. M. Theriot, Diversification of host bile acids by members of the gut microbiota, Gut Microbes, 2020, 11, 158–171 CrossRef PubMed.
- W. Zhou and G. F. Sonnenberg, Activation and suppression of group 3 innate lymphoid cells in the gut, Trends Immunol., 2020, 41, 721–733 CrossRef CAS PubMed.
- B. D. Luccia, S. Gilfillan, M. Cella, M. Colonna and S. C. Huang, ILC3s integrate glycolysis and mitochondrial production of reactive oxygen species to fulfill activation demands, J. Exp. Med., 2019, 216, 2231–2241 CrossRef CAS PubMed.
- Z. Fu, J. W. Dean, L. Xiong, M. W. Dougherty, K. N. Oliff, Z. E. Chen, C. Jobin, T. J. Garrett and L. Zhou, Mitochondrial transcription factor A in RORγt+ lymphocytes regulate small intestine homeostasis and metabolism, Nat. Commun., 2021, 12, 4462 CrossRef CAS PubMed.
- C. Y. Han, T. H. Kim, J. H. Koo and S. G. Kim, Farnesoid X receptor as a regulator of fuel consumption and mitochondrial function, Arch. Pharmacal Res., 2016, 39, 1062–1074 CrossRef CAS PubMed.
- V. T. Vachharajani, T. Liu, X. Wang, J. J. Hoth, B. K. Yoza and C. E. McCall, Sirtuins link inflammation and metabolism, J. Immunol. Res., 2016, 2016, 8167273 Search PubMed.
- D. Yang, Q. Zhou, V. Labroska, S. Qin, S. Darbalaei, Y. Wu, E. Yuliantie, L. Xie, H. Tao, J. Cheng, Q. Liu, S. Zhao, W. Shui, Y. Jiang and M. Wang, G protein-coupled receptors: Structure- and function-based drug discovery, Signal Transduction Targeted Ther., 2021, 6, 7 CrossRef CAS PubMed.
- M. Quiros, Therapeutic opportunities for repair GPCRs during intestinal mucosal wound healing, Trends Mol. Med., 2020, 26, 971–974 CrossRef CAS PubMed.
- C. Chu, S. Moriyama, Z. Li, L. Zhou, A. Flamar, C. S. N. Klose, J. B. Moeller, G. G. Putzel, D. R. Withers, G. F. Sonnenberg and D. Artis, Anti-microbial functions of group 3 innate lymphoid cells in gut-associated lymphoid tissues are regulated by G-protein-coupled receptor 183, Cell Rep., 2018, 23, 3750–3758 CrossRef CAS PubMed.
- Q. Hou, J. Huang, X. Xiong, Y. Guo and B. Zhang, Role of nutrient-sensing receptor GPRC6A in regulating colonic group 3 innate lymphoid cells and inflamed mucosal healing, J. Crohn's Colitis, 2022, 20, 1–13 Search PubMed.
- C. A. Thaiss, N. Zmora, M. Levy and E. Elinav, The microbiome and innate immunity, Nature, 2016, 535, 65–74 CrossRef CAS PubMed.
- Z. Lv, H. Dai, Q. Wei, S. Jin, J. Wang, X. Wei, Y. Yuan, D. Yu and F. Shi, Dietary genistein supplementation protects against lipopolysaccharide-induced intestinal injury through altering transcriptomic profile, Poult. Sci., 2020, 99, 3411–3427 CrossRef CAS PubMed.
- Q. Fan, K. F. M. Abouelezz, L. Li, Z. Gou, Y. Wang, X. Lin, J. Ye and S. Jiang, Influence of mushroom polysaccharide, nano-copper, copper loaded chitosan, and lysozyme on intestinal barrier and immunity of LPS-mediated yellow-feathered chickens, Animals, 2020, 10, 594 CrossRef PubMed.
- H. S. Lillehoj and K. S. Chung, Postnatal development of T-lymphocyte subpopulations in the intestinal intraepithelium and lamina propria in chickens, Vet. Immunol. Immunopathol., 1992, 31, 347–360 CrossRef CAS PubMed.
- G. I. Solano-Aguilar, K. G. Vengroski, E. Beshah and J. K. Lunney, Isolation and purification lymphocyte subsets from gut associated lymphoid tissue in neonatal swine, J. Immunol. Methods, 2000, 241, 185–199 CrossRef CAS PubMed.
- S. Cao, C. Wang, J. Yan, X. Li, J. Wen and C. Hu, Curcumin ameliorates oxidative stress-induced intestinal barrier injury and mitochondrial damage by promoting Parkin dependent mitophagy through AMPK-TFEB signal pathway, Free Radicals Biol. Med., 2020, 147, 8–22 CrossRef CAS PubMed.
- M. Song, J. Ye, F. Zhang, H. Su, X. Yang, H. He, F. Liu, X. Zhu, L. Wang, P. Gao, G. Shu, Q. Jiang and S. Wang, Chenodeoxycholic acid (CDCA) protects against the lipopolysaccharide-induced impairment of the intestinal epithelial barrier function via the FXR-MLCK pathway, J. Agric. Food Chem., 2019, 67, 8868–8874 CrossRef CAS PubMed.
- R. J. Pandit, A. T. Hinsu, N. V. Patel, P. G. Koringa, S. J. Jakhesara, J. R. Thakkar, T. M. Shah, G. Limon, A. Psifidi, J. Guitian, D. A. Hume, F. M. Tomley, D. N. Rank, M. Raman, K. G. Tirumurugan, D. P. Blake and C. G. Joshi, Microbial diversity and community composition of caecal microbiota in commercial and indigenous Indian chickens determined using 16s rDNA amplicon sequencing, Microbiome, 2018, 6, 115 CrossRef PubMed.
- H. Wang, S. Yan, H. Xin, W. Huang, H. Zhang, S. Teng, Y. Yu, A. R. Fernie, X. Lu, P. Li, S. Li, C. Zhang, Y. Ruan, L. Chen and Z. Lang, A subsidiary cell-localized glucose transporter promotes stomatal conductance and photosynthesis, Plant Cell, 2019, 31, 1328–1343 CrossRef CAS PubMed.
- S. Yan, C. Zhu, T. Yu, W. Huang, J. Huang, Q. Kong, J. Shi, Z. Chen, Q. Liu, S. Wang, Z. Jiang and Z. Chen, Studying the differences of bacterial metabolome and microbiome in the colon
between Landrace and Meihua piglets, Front. Microbiol., 2017, 8, 1812–1826 CrossRef PubMed.
- S. Reiter, A. Dunkel, C. Dawid and T. Hofmann, Targeted LC-MS/MS profiling of bile acids in various animal tissues, J. Agric. Food Chem., 2021, 69, 10572–10580 CrossRef CAS PubMed.
- S. Wu, R. Hu, H. Nakano, K. Chen, M. Liu, X. He, H. Zhang, J. He and D. Hou, Modulation of gut microbiota by Lonicera caerulea L. berry polyphenols in a mouse model of fatty liver induced by high fat diet, Molecules, 2018, 23, 3213 CrossRef PubMed.
- E. Amabebe, F. O. Robert, T. Agbalalah and E. S. F. Orubu, Microbial dysbiosis-induced obesity: Role of gut microbiota in homoeostasis of energy metabolism, Br. J. Nutr., 2020, 123, 1127–1137 CrossRef CAS PubMed.
- Y. Huang, X. Shi, Z. Li, Y. Shen, X. Shi, L. Wang, G. Li, Y. Yuan, J. Wang, Y. Zhang, L. Zhao, M. Zhang, Y. Kang and Y. Liang, Possible association of Firmicutes in the gut microbiota of patients with major depressive disorder, Neuropsychiatr. Dis. Treat., 2018, 14, 3329–3337 CrossRef CAS PubMed.
- J. Libertucci, U. Dutta, S. Kaur, J. Jury, L. Rossi, M. E. Fontes, M. S. Shajib, W. I. Khan, M. G. Surette, E. F. Verdu and D. Armstrong, Inflammation-related differences in mucosa-associated microbiota and intestinal barrier function in colonic Crohn's disease, Am. J. Physiol.: Gastrointest. Liver Physiol., 2018, 315, 420–431 CrossRef PubMed.
- H. Li, H. Zhang, F. Zhao, S. Wang, Z. Wang and Z. Wei, Modulation of gut microbiota, short-chain fatty acid production, and inflammatory cytokine expression in cecum of porcine deltacoronavirus-infected chicks, Front. Microbiol., 2020, 11, 897 CrossRef PubMed.
- Y. Nakanishi, T. Sato and T. Ohteki, Commensal Gram-positive bacteria initiate colitis by inducing monocyte/macrophage mobilization, Mucosal Immunol., 2015, 8, 152–160 CrossRef CAS PubMed.
- J. C. Clemente, J. Manasson and J. U. Scher, The role of the gut microbiome in systemic inflammatory disease, BMJ, 2018, 360, 5145 CrossRef PubMed.
- S. S. Zhai, D. Ruan, Y. W. Zhu, M. C. Li, H. Ye, W. C. Wang and L. Yang, Protective effect of curcumin on ochratoxin A-induced liver oxidative injury in duck is mediated by modulating lipid metabolism and the intestinal microbiota, Poult. Sci., 2020, 99, 1124–1134 CrossRef CAS PubMed.
- F. Zhang, Y. Zhou, H. Chen, H. Jiang, F. Zhou, B. Lv and M. Xu, Curcumin alleviates DSS-induced anxiety-like behaviors via the microbial-brain-gut axis, Oxid. Med. Cell. Longevity, 2022, 2022, 6244757 Search PubMed.
- V. Eckhaut, K. Machiels, C. Perrier, C. Romero, S. Maes, B. Flahou, M. Steppe, F. Haesebrouck, B. Sas, R. Ducatelle, S. Vermeire and F. V. Immerseel,
Butyricicoccus pullicaecorum in inflammatory bowel disease, Gut, 2013, 62, 1745–1752 CrossRef PubMed.
- N. Serafini, A. Jarade, L. Surace, P. Goncalves, O. Sismeiro, H. Veret, R. Legendre, J. Coppee, O. Disson, S. Durum, G. Frankel and J. P. Di Santo, Trained ILC3 responses promote intestinal defense, Science, 2022, 375, 859–863 CrossRef CAS PubMed.
- J. Xu, D. Huang, X. Xu, X. Wu, L. Liu, W. Niu, L. Lu and H. Zhou, An elevated deoxycholic acid level induced by high-fat feeding damages intestinal stem cells reducing the ileal IL-22, Biochem. Biophys. Res.
Commun., 2021, 579, 153–160 CrossRef CAS PubMed.
- A. Lavelle and H. Sokol, Gut microbiota-derived metabolites as key actors in inflammatory bowel disease, Nat. Rev. Gastroenterol. Hepatol., 2020, 17, 223–237 CrossRef PubMed.
- H. Duboc, S. Rajca, D. Rainteau, D. Benarous, M. Maubert, E. Quervain, G. Thomas, V. Barbu, L. Humbert, G. Despras, C. Bridonneau, F. Dummetz, J. Grill, J. Masliah, L. Beaugerie, J. Cosnes, O. Chazouilleres, R. Poupon, C. Wolf, J. Mallet, P. Langella, G. Trugnan, H. Sokol and P. Seksik, Connecting dysbiosis, bile-acid dysmetabolism and gut inflammation in inflammatory bowel diseases, Gut, 2013, 62, 531–539 CrossRef CAS PubMed.
- S. Kim, B. Cho, H. Kiyono and Y. Jang, Microbiota-derived butyrate suppresses group 3 innate lymphoid cells in terminal ileal Peyer's patches, Sci. Rep., 2017, 7, 3980 CrossRef PubMed.
- Z. Xiao, L. Liu, Y. Jin, X. Pei, W. Sun and M. Wang,
Clostridium Tyrobutyricum protects against LPS-induced colonic inflammation via IL-22 signaling in mice, Nutrients, 2021, 13, 215 CrossRef CAS PubMed.
- A. Banerjee, C. A. Herring, B. Chen, H. Kim, A. J. Simmons, A. N. Southard-Smith, M. M. Allaman, J. R. White, M. C. Macedoina, E. T. Mckinley, M. A. Ramirez-Solano, E. A. Scoville, Q. Liu, K. T. Wilson, R. J. Coffey, M. K. Washington, J. A. Goettel and K. S. Lau, Succinate produced by intestinal microbes promotes specification of tuft cells to suppress ileal inflammation, Gastroenterology, 2020, 159, 2101–2115 CrossRef CAS PubMed.
- S. Lin, Z. Wang, K. Lam, S. Zeng, B. Tan and J. Hu, Role of intestinal microecology in regulation of energy metabolism by dietary polyphenols and their metabolites, Food Nutr. Res., 2019, 63, 1518 CAS.
- S. Fiorucci, A. Zampella, P. Ricci, E. Distrutti and M. Biagioli, Immunomodulatory functions of FXR, Mol. Cell. Endocrinol., 2022, 551, 111650 CrossRef CAS PubMed.
- A. Wahlström, S. I. Sayin, H. Marschall and F. Bäckhd, Intestinal crosstalk between bile acids and microbiota and its impact on host metabolism, Cell Metab., 2016, 24, 41–50 CrossRef PubMed.
- E. Chun, S. Lavoie, D. Fonseca-Pereira, S. Bae, M. Michaud, H. R. Hoveyda, G. L. Fraser, C. A. G. Comeau, J. N. Glickman, M. H. Fuller, B. T. Layden and W. S. Garrett, Metabolite-sensing receptor Ffar2 regulates colonic group 3 innate lymphoid cells and gut immunity, Immunity, 2019, 51, 871–884 CrossRef CAS PubMed.
- Z. Han, L. Yao, Y. Zhong, Y. Xiao, J. Gao, Z. Zheng, S. Fan, Z. Zhang, S. Gong, S. Chang, X. Cui and J. Cai, Gut microbiota mediates the effects of curcumin on enhancing Ucp1-dependent thermogenesis and improving high-fat diet-induced obesity, Food Funct., 2021, 12, 6558–6575 RSC.
- S. Pande and S. Raisuddin, Molecular and cellular regulatory roles of sirtuin protein, Crit. Rev. Food Sci. Nutr., 2022, 1–19 CrossRef PubMed.
- R. Sun, Z. Zhang, R. Bao, X. Guo, Y. Gu, W. Yang, J. Wei, X. Chen, L. Tong, J. Meng, C. Zhong, C. Zhang, J. Zhang, Y. Sun, C. Ling, X. Tong, F. Yu, H. Yu, W. Qu, B. Zhao, W. Guo, M. Qian, H. Saiyin, Y. Liu, R. Liu, C. Xie, W. Liu, Y. Xiong, K. Guan, Y. Shi, P. Wang and D. Ye, Loss of SIRT5 promotes bile acid-induced immunosuppressive microenvironment and hepatocarcinogenesis, J. Hepatol., 2022, 77, 453–466 CrossRef CAS PubMed.
- J. Zhang, Y. Yang, H. Han, L. Zhang and T. Wang, Bisdemethoxycurcumin protects small intestine from lipopolysaccharide-induced mitochondrial dysfunction via activating mitochondrial antioxidant systems and mitochondrial biogenesis in broiler chickens, Oxid. Med. Cell. Longevity, 2021, 2021, 9927864 Search PubMed.
|
This journal is © The Royal Society of Chemistry 2022 |
Click here to see how this site uses Cookies. View our privacy policy here.