DOI:
10.1039/D2FO01739C
(Paper)
Food Funct., 2022,
13, 11185-11199
Amyloid β-but not Tau-induced neurotoxicity is suppressed by Manuka honey via HSP-16.2 and SKN-1/Nrf2 pathways in an in vivo model of Alzheimer's disease
Received
21st June 2022
, Accepted 29th September 2022
First published on 7th October 2022
Abstract
Alzheimer's is a chronic degenerative disease of the central nervous system considered the leading cause of dementia in the world. It is characterized by two etiopathological events related to oxidative stress: the aggregation of β-amyloid peptide and the formation of neurofibrillary tangles of hyperphosphorylated Tau protein in the brain. The incidence of this disease increases with age and has been associated with inadequate lifestyles. Some natural compounds have been shown to improve the hallmarks of the disease. However, despite its potential, there is no scientific evidence about Manuka honey (MH) in this regard. In the present work we evaluated the effect of MH on the toxicity induced by Aβ aggregation and Tau in a Caenorhabditis elegans model. Our results demonstrated that MH was able to improve indicators of oxidative stress and delayed Aβ-induced paralysis in the AD model CL4176 through HSP-16.2 and SKN-1/NRF2 pathways. Nevertheless, its sugar content impaired the indicators of locomotion (an indicator of tau neurotoxicity) in both the transgenic strain BR5706 and in the wild-type N2 worms.
1. Introduction
Alzheimer's disease (AD) is a progressive neurodegenerative disease of the central nervous system, characterized by accumulation of amyloid-β (Aβ) peptide and abnormal strands of hyperphosphorylated protein Tau (tangles) in the brain.1–3 These modifications are accompanied by neuronal loss2,4 along with synaptic and brain tissue damage2,5 resulting in memory impairment,1,6 depression, disorientation, poor judgment and, in later stages, speaking and walking difficulties.5 It is estimated that AD causes between 60–80% of all dementia cases,5 representing an important economic burden worldwide.4 Currently, most of the approved drugs for the treatment of the illness only alleviate symptoms, but do not modify the underlying biology of Alzheimer's or alter the course of the disease. In addition, most of these drugs usually are accompanied by side effects including nausea, headache,5 insomnia and hepatotoxicity.3 Only one of the FDA-approved drugs, aducanumab, can reduce Aβ plaques in the brain.5 Hence, the search for new drug candidates to fight AD is still an attractive research area. In the last years, several studies have shown that bioactive compounds from natural products possess neuroprotective properties and are capable of relieving AD symptoms, indicating that natural source-based drugs could be a valid alternative in this therapeutic area.3,4,7
In that sense, Manuka honey (MH), a much appreciated honey derived from the Leptospermum scoparium tree (Myrtaceae family), typical in New Zealand, eastern Australia and the Mediterranean region, has shown excellent biological activities including antibacterial, antioxidant, wound-healing and antiproliferative properties as well as a very interesting physicochemical composition.8,9,10–12 However, to the best of our knowledge, there is no evidence on its neuroprotective potential.
On the other hand, the nematode Caenorhabditis elegans (C. elegans), due to its short lifespan and its limited number of neurons and tractable genetics, represents an ideal system to study aging and age-related diseases, such as AD.1,2,13 Likewise, there are multiple transgenic strains, specifically designed to examine the Aβ plaque accumulation and tangle-caused neurotoxicity.2,6,13
In the present study, we investigated the effects of MH on Aβ aggregation and tau neurotoxicity in C. elegans AD models.
2. Materials & methods
2.1 Honey samples and reagents
MH was bought in a local supermarket in Spain after importation into Europe by the Comptoirs et Compagnies. All reagents were purchased from Sigma-Aldrich (St Louis, Missouri, USA), Merck (Darmstadt, Germany), Thermo Fisher (Waltham, Massachusetts, USA) or Roche (Basel, Switzerland).
2.2 MH characterization
2.2.1 Total phenolic compounds (TPC) and total flavonoids content (TFC) determination.
TPC of MH were determined by the Folin-Ciocalteu method,14 while its flavonoid content was evaluated according to the protocol described by Navarro-Hortal et al.15
2.2.2 Total antioxidant capacity (TAC).
TAC of MH was evaluated by ferric reducing antioxidant power (FRAP), trolox equivalent antioxidant capacity (TEAC) and 2,4-DNP; 2,2-diphenyl-1-picrylhydrazyl (DPPH) methods, as previously reported by our group.15–17 In all cases, the absorbance was measured in a Neo2 microplate reader (Biotek, Winooski, Vermont, U.S.A.) and the results were expressed as μM of Trolox equivalents (TE) per gram (g) of honey.
2.2.3 Extraction, identification, and quantification of phenolic compounds.
The extraction of phenolic compounds was performed according to Afrin et al.11 For their identification a SYNAPT G2 HDMS Q-TOF system (Waters, Mildford, USA) equipped with a dual electrospray ionization probe was used, following the protocol reported by Esteban-Muñoz et al.18 UPLC separation was performed using an ACQUITY UPLC ™ HSS T3 2.1 × 100 mm, 1.8 mm column and the program for chromatography was set with a binary gradient consisting of (A) water with 0.5% acetic acid and (B) acetonitrile, as follows: initial 0.0 min 5% (B), 15.0 min 95% (B); 15.1 min 5% (B); and 18.0 min 5% (B) and the flow rate was 0.4 mL min−1. Phenolic compounds were identified through the MassLynx V4 software (Waters Laboratory Informatics, Mildford, USA) by comparing the ions and molecular fragments obtained with previous data.
The quantification was carried out by an ACQUITY UPLC I-Class System (Waters, Mississauga, ON, Canada) equipped with a mass spectrometer Waters XEVO TQ-XS with ionization performed by UniSpray (US), according to the method described by Sánchez-Hernández et al.19 A gradient consisting of (A) water and (B) methanol with 0.1% [v/v] acetic acid for 25 min at a flow rate of 0.4 mL min−1 was set as follows: 0.0 min 5% B, 15–15.10 min 95% B and 15.10–25 min 5% B. An ACQUITY UPLC ™ HSS T3 1.8 mm column was used. Quantification of the phenolic compounds was achieved by comparing the retention times of peaks and fragmentation data obtained, with those of the phenolic compound standards. Measurements in Multiple reaction monitoring was used.
2.3
C. elegans strains and maintenance conditions
Wild-type N2 Bristol C. elegans, the transgenic strains CL802 [smg-1(cc546) I; rol-6(su1006) II], CL4176 (dvIs27 [myo-3p::A-Beta (1–42)::let-851 3′ UTR) + rol-6(su1006)] X), CF1553 (mu1s84[pAD76 (sod-3::GFP) + rol-6(su1006)]), CL2166 (dvIs19 [(pAF15)gst-4p:GFP:NLS] III), LD1 (ldIs7 [skn-1b/c::GFP + rol-6(su1006)]), TJ375 (gpIs1[hsp-16.2::GFP]), OS3062 [myo-2p::hsf-1 + hsp-16.2::GFP + hsp-16.41::GFP + rol-6(su1006)], TJ356 (zIs356[daf-16p::daf-16a/b::GFP + rol-6 (su1006)]), BR5706 (bkIs10 [aex-3p:hTau V337M + myo-2p:GFP]) and E. coli OP50 were obtained from the Caenorhabditis Genetics Center (Minneapolis, MI, USA). All worms were grown and maintained on nematode growth medium (NGM)-agar plates seeded with a lawn of E. coli OP50 at 20 °C or 16 °C, depending on the strain's characteristics or the experiment design.
To obtain age-synchronized nematodes, the bleaching protocol was used. Briefly, gravid hermaphrodites were exposed to an alkaline bleach solution (0.5 N NaOH in 20% NaOCl) and vigorously stirred for six minutes followed by centrifugation at 1280g × 2 minutes. Settled eggs were then resuspended and washed for three times with M9 medium. After that, eggs were seeded on fresh NGM agar plates with a lawn of E. coli OP50 and allowed to hatch. Worms were allowed to mature until the desired larval stage, changing the plates every 2–3 days.
2.4 Lethality test
To assess acute lethality, N2 Bristol worms synchronized at L4 larval stage were placed on NGM plates containing 0, 25, 50, 100, 200 or 300 mg mL−1 of MH for 24 h at 20 °C in the absence of E. coli OP50. After that time, nematodes were scored as live or dead by using a Motic dissecting microscope (Motic Inc., Ltd, Hong Kong, China). Worms that did not respond to repeated stimuli inflicted with a platinum wire were considered dead. At least 30 worms were used per group and the experiment was repeated at least three times. Results were expressed as survival rate. For the subsequent experiments a non-lethal submaximal concentration (100 mg mL−1) of MH was used.
2.5 Pharyngeal pumping assay and growth evaluation
Egg-synchronized N2 worms were placed on NGM plates seeded with E. coli OP50 and containing or not 100 mg mL−1 MH and then incubated at 20 °C. Ninety-six hours later, worms were transferred to fresh plates and the number of contractions per minute of the pharynx terminal bulb was counted using a Motic microscope (Motic Inc. Ltd Hong Kong, China). At the end, worms were collected by washing with M9 medium and analyzed through a MultiRange large particle flow cytometer Biosorter (Union Biometrica. Massachusetts, USA) to determine the time of flight (TOF) which is indicative of the length. At least 10 worms were used per group for pharyngeal pump counting and about 100 nematodes to determine the size. Both experiments were performed in triplicate.
2.6 Eggs fertility assay
L4 stage synchronized N2 worms were individually placed in 24 well NGM plates seeded with E. coli OP50 and containing or not 100 mg mL−1 MH. After 24 h at 20 °C, the laid eggs were counted by using a Motic dissecting microscope (Motic Inc., Ltd, Hong Kong, China) and the worms were moved to a fresh plate to distinguish from the offspring. This procedure was repeated every single day until egg-laying stopped. At least 15 worms were used per group and the experiment was repeated at least three times. Results were expressed as the mean of the total number of eggs per group.
2.7 Oxidative stress resistance assays
To assess oxidative stress resistance, the intracellular reactive oxygen species (ROS) levels in L4 stage-synchronized N2 worms were measured by using the 2′,7′-dichlorofluorescein diacetate (DCFDA) assay, as previously described by Navarro-Hortal et al.15 Briefly, eggs-synchronized embryos were placed on NGM plates seeded with E. coli OP50 containing or not 100 mg mL−1 MH for 48 h at 20 °C. Next, young-adult worms were collected and washed three times with M9 medium in order to remove bacteria and were exposed (except control group) to 2.5 μM AAPH (2,2′-azobis-2-amidinopropane dihydrochloride) for 15 min to induce oxidative stress. Worms were further washed with M9 medium and directly incubated with the fluorogenic dye (final concentration, 25 μM) for 2 h. The fluorescence was measured by a MultiRange large particle flow cytometer Biosorter (Excitation wavelength 490 nm, emission wavelength 510 nm) (Union Biometrica. Massachusetts, USA). Results were expressed as the mean of the fluorescence intensity normalized by TOF. At least 200 nematodes were used per group and the experiments were repeated in triplicate.
2.8 Paralysis test
Transgenic strain CL4176 and the non-paralyzable control strain CL802 were synchronized as described above in the maintenance conditions section and placed on NGM plates seeded with E. coli OP50 containing or not 100 mg mL−1 MH. Worms were incubated for 48 h at 16 °C and then transferred to a 25 °C incubator to stimulate the Aβ-induced paralysis. After 20 h, 25 nematodes of each group were picked to fresh NGM plates (maintaining temperature conditions) and scored for paralysis every 2 h until 32 h after the temperature rise. Nematodes were considered paralyzed when they did not respond to repeated stimuli inflicted with a platinum wire or if they only moved the head without showing a full body wave. Results were expressed as percentage of non-paralyzed worms. The experiment was performed in triplicate.
2.9 β-Amyloid aggregates visualization
Worms of the paralyzable strain CL4176 and the negative control strain CL802 were grown as described above for the paralysis assay. After 26 h of incubation at 16 °C, worms were fixed with a 4% paraformaldehyde/M9 medium solution (pH 7.4) for 24 h and subsequently permeabilized with a solution containing 5% fresh β-mercaptoethanol, 125 mM Tris and 1% Triton X-100 (pH 7.4) for another 24 h at 37 °C. Aβ-aggregates were stained with 0.125% Thioflavin T (Sigma-Aldrich, St Louis, MI, USA) in 50% ethanol for 30 min and were observed by using a Nikon epi-fluorescence microscope (Eclipse Ni, Nikon, Tokyo, Japan). About 20–30 images per group were acquired at 40× magnification using the GFP filter with a Nikon DS-Ri2 camera (Tokyo, Japan).
2.10 RNA Interference (RNAi) Assay
Superoxide dismutase (SOD)-2 and 3, heat shock protein 16.2 (HSP-16.2), transcription factor skinhead-1 (SKN-1) and dauer formation (DAF-16) gene expression was knocked down by feeding CL4176 worms with E. coli strain HT115 carrying the specific dsRNA. Briefly, L3/L4 stage-synchronized CL4176 worms were transferred to RNAi plates containing 1 mM isopropyl β-D-1-thiogalactopyranoside (IPTG) and 25 μg mL−1 carbenicillin and incubated for 48–72 h. Once they reached the fertile age, another synchronization was carried out and the eggs/embryos obtained were placed on fresh RNAi plates containing or not 100 mg mL−1 MH and were allowed to mature to L4 young larvae. The resulting adult worms were used for the paralysis assay as previously described.
2.11 Green fluorescence protein (GFP)-reporter transgenic strains
Egg-synchronized worms of the transgenic strains CF1553, CL2166, LD1, OS3062, TJ375, and TJ356 expressing the GFP reporter SOD-3, glutathione S-transferase 4 (GST-4), SKN-1, heat-shock transcription factor 1 (HSF-1), HSP-16.2 and DAF-16, respectively, were grown in NGM plates seeded with E. coli OP50 containing or not 100 mg mL−1 MH for 48 h at 20 °C. Next, 20–30 worms per group were immobilized with sodium azide (1 mM) on a slide and subjected to imaging using a Nikon epi-fluorescence microscope (Eclipse Ni, Nikon, Tokyo, Japan). The total GFP fluorescence was analyzed using the NIS-Elements BR software (Nikon, Tokyo, Japan). The subcellular DAF-16 distribution in the TJ356 strain was analyzed by classifying the expression/location patterns into three categories: cytosolic, intermediate, and nuclear; each of which was assigned a value (1, 2, 3) to carry out a semi-quantification. The experiments were repeated at least three times.
2.12 Locomotion behavior analysis
Egg-synchronized embryos of wild type-N2 and BR5706 strains were placed on NGM plates seeded with E. coli OP50 containing or not different treatments (100 mg mL−1 MH, 100 mg mL−1 artificial honey (AH) or 232 μg mL−1 of MH hydroalcoholic extract (ME) for 72 h at 20 °C. Next, about 20–30 worms per group were forced to swim by placing them in a drop of M9 medium in the center of a slide. Worms movement was tracked, recorded and analyzed by using a WormLab Imaging System (MBF Bioscience, Williston, Vermont, EE. UU). The wavelength, swimming speed and activity were analyzed as representative parameters of locomotive behavior. The experiment was carried out at least in triplicate.
2.13 Statistical analysis
Statistical analysis was performed by SPSS 24.0 software (IBM, Armonk, NY, USA). Data were subjected to one-way analysis of variance (ANOVA) followed by Tukey's honestly significant difference (HSD) post hoc test to compare statistical difference among multiple groups or to t-student test for pairwise data sets. A p < 0.05 was considered statistically significant. Data are expressed as mean ± SEM from at least three independent experiments.
3. Results
3.1 MH characterization
A total of 31 compounds were identified in MH (Table 1) and despite the limit of quantification for some of them, it was possible to quantify quercetin, quercetin-3-O-glucopyranoside, chrysanthemin, epicatechin, apigenin, naringenin, m-coumaric acid, ferulic acid, rosmarinic acid, ellagic acid and o-vanillin (Table 2). Naringenin (295.3 ± 4.7 ppb) and o-vanillin (373.5 ± 5.6 ppb) were the major compounds. Hydroxybenzaldehydes represented 45.14% of the quantified compounds, while flavonoids and phenolic acids represented 42.78% and 11.97%, respectively (Table 2).
Table 1 Phenolic compounds identified in MH
Compounds |
CAS number |
Ion mode |
[M]+/− (m/z) |
Ref. |
Flavanones
|
Naringenin |
67604-48-2 |
+ |
153 |
20–23
|
− |
151 |
Pinocembrin |
480-39-7 |
+ |
151 |
23–26
|
− |
255 |
Sakuranetin |
2957-21-3 |
+ |
133 |
25 and 26 |
− |
287 |
Flavones
|
Apigenin |
520-36-5 |
+ |
153 |
22–25 and 27 |
− |
117 |
Chrysin |
480-40-0 |
+ |
143 |
21 and 23–28 |
− |
253 |
Luteolin |
491-70-3 |
+ |
153 |
20 and 22–28 |
− |
133 |
Nepetin |
520-11-6 |
− |
315 |
29
|
Flavonols
|
Galangin |
548-83-4 |
− |
213 |
23–28
|
Isorhamnetin |
480-19-3 |
− |
199 |
20, 25, 26 and 28 |
Kaempferol |
520-18-3 |
+ |
153 |
20 and 22–27 |
− |
151 |
Quercetin |
117-39-5 |
− |
151 |
20–27
|
Quercetin 3-O-rutinoside |
153-18-4 |
+ |
609 |
20, 22–25 and 27 |
Rhamnetin |
90-19-7 |
− |
315 |
25, 26 and 28 |
Isoflavonoids
|
Biochanin A |
491-80-5 |
+ |
283 |
27
|
− |
268 |
Daidzein |
486-66-8 |
+ |
253 |
23 and 27 |
− |
209 |
Formononetin |
485-72-3 |
− |
252 |
27
|
Genistein |
446-72-0 |
+ |
269 |
27
|
− |
181 |
Hydroxybenzaldehydes
|
Syringaldehyde |
134-96-3 |
+ |
181 |
28 and 30 |
Vanillin |
121-33-5 |
− |
151 |
30 and 31 |
Hydroxycoumarins
|
Scopoletin |
92-61-5 |
− |
190 |
32
|
Hydroxybenzoic acids
|
Syringic acid |
530-57-4 |
+ |
197 |
20, 22, 28, 31 and 33 |
4-Hydroxybenzoic acid |
99-96-7 |
+ |
137 |
22, 23, 26–28, 30 and 33 |
Vanillic acid |
121-34-6 |
− |
152 |
22, 23, 27, 30 and 33 |
Hydroxycinnamic acids
|
3-Caffeoylquinic acid |
906-33-2 |
− |
353 |
25
|
5-Caffeoylquinic acid |
327-97-9 |
− |
353 |
23, 31 and 34 |
Caffeic acid |
331-39-5 |
+ |
179 |
20–22, 24–28, 30 and 35 |
− |
135 |
Cinnamic acid |
621-82-9 |
− |
147 |
25 and 33 |
Ferulic acid |
1135-24-6 |
+ |
193 |
20–24, 26, 27, 30, 32, 33 and 35 |
− |
134 |
− |
|
p-Coumaric acid |
501-98-4 |
+ |
163 |
20, 22, 23, 25–27, 30, 32 and 35 |
− |
119 |
− |
|
Hydroxyphenylacetic acids
|
4-Hydroxyphenylacetic acid |
156-38-7 |
− |
107 |
22 and 23 |
Phenylacetic acid |
103-82-2 |
− |
107 |
27
|
Table 2 Quantification of the phenolic compounds identified in MH
Phenolic compounds |
Mean (ppb) ± SD |
LOQ: limit of quantification. Data are means (n = 3). |
Flavonols
|
Quercetin |
52.6 ± 1.4 |
Flavonol glycosides
|
Rutin |
<LOQ |
Kaempferol-3-O-glucoside |
<LOQ |
Quercetin-3-O-glucopyranoside |
0.6 ± 0.2 |
Anthocyanins
|
Chrysanthemin |
0.3 ± 0.0 |
Flavanols
|
Epicatechin |
4.5 ± 0.4 |
Flavones
|
Apigenin |
1.6 ± 0.4 |
Flavanones
|
Naringenin |
295.3 ± 4.7 |
Isoflavones
|
Glycitein |
<LOQ |
Formononetin |
<LOQ |
Phenolic acids
|
m-Coumaric acid |
16 ± 1.0 |
Ferulic acid |
6.3 ± 0.1 |
Rosmarinic acid |
3.5 ± 0.2 |
Ellagic acid |
73.2 ± 6.1 |
Hydroxybenzaldehydes
|
o-Vanillin |
373.5 ± 5.6 |
|
Total phenolic content
|
827.4
|
According to the colorimetric methods, the TPC of MH was 0.47 ± 0.03 mg GAE g−1, while its TFC was 0.05 ± 0.01 mg CAE g−1. Likewise, its antioxidant capacity was 2.65 ± 0.10 μM Trolox eq. per g, 1.40 ± 0.13 μM Trolox eq. per g and 4.58 ± 0.22 μM Trolox eq. per g according to the FRAP, ABTS and DPPH determination methods, respectively. The yield of the hydroalcoholic extraction of phenolic compounds was 2.32 ± 0.42 mg g−1 of honey (Table 3).
Table 3 Phytochemical characterization, antioxidant capacity and yield of MH
Parameter |
Mean ± SEM |
mg of hydroalcoholic extract obtained per g of honey. FRAP: ferric reducing antioxidant power; DPPH: 2,2-diphenyl1-picryl-hydrazyl-hydrate; TEAC: trolox equivalent antioxidant capacity; mg GAE g−1: mg gallic acid equivalent; mg CAE g−1: mg catechin equivalent; TE: trolox equivalent. |
Total phenolic content (mg GAE g−1) |
0.47 ± 0.03 |
Total flavonoids content (mg CAE g−1) |
0.05 ± 0.01 |
FRAP (μM TE g−1) |
2.65 ± 0.10 |
DPPH (μM TE g−1) |
1.40 ± 0.13 |
TEAC (μM TE g−1) |
4.58 ± 0.22 |
|
Yielda |
2.32 ± 0.42 |
3.2 Short term toxicity of MH
The short-term toxicity of MH in C. elegans was evaluated through different assays, including 24 h lethality, pharyngeal pumping, egg-laying (fertility) and growth. As shown in Fig. 1A, no statistically significant differences (p < 0.05) were found between the control group and worms treated with increasing concentrations (up to 300 mg mL−1) of MH in terms of survival percentage. Likewise, MH supplementation did not affect the number of pumps per minute or the worm's fertility, understood as their spawning capacity. However, worms treated with 100 mg mL−1 MH were slightly smaller (p < 0.05) (Fig. 1B).
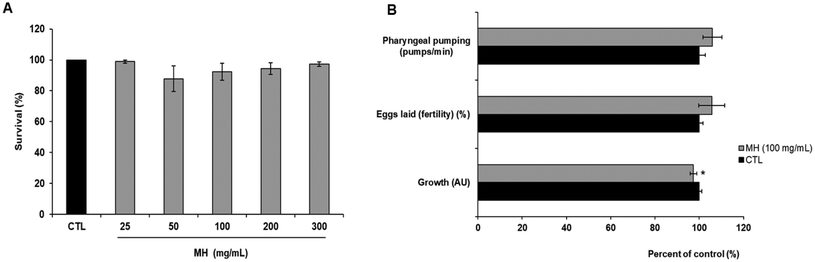 |
| Fig. 1 Short-term toxicity of Manuka honey in the N2 wild type strain. (A) 24 h lethality. (B) Pharyngeal pumping, egg-laying (fertility) and growth. Worms were treated with the indicated concentration of MH for 24 h. Data are expressed as mean ± SEM of three independent experiments (n = 3). Asterisk marks (*) indicate statistically significant differences (p < 0.05) compared to control (non-treated worms). CTL: control; MH: Manuka honey. | |
3.3 Effects of MH against AAPH-induced oxidative stress
An escalation in endogenous ROS levels accelerates the aging process and, in turn, the nematodes ability to deal with harmful stimuli decreases drastically over time. To evaluate the effects of MH against AAPH-induced stress, we measured intracellular ROS levels by DCF-DA method. As shown in Fig. 2, nematodes treated with MH presented a greater resistance against these stress conditions. MH completely prevented the increase in ROS observed in the stressed group as no significant difference (p < 0.05) was found compared to the control.
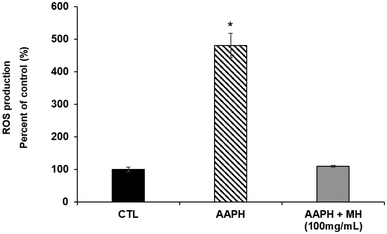 |
| Fig. 2 Effects of Manuka honey on the reactive oxygen species (ROS) production. Worms were pre-treated with MH at 100 mg mL−1 for 24 h and then exposed to AAPH (2.5 mM) for 15 min. Data are expressed as mean ± SEM of three independent experiments (n = 3). Asterisk marks (*) indicate statistically significant differences (p < 0.05) compared to control (non-treated worms). CTL: control; MH: Manuka honey; AAPH: 2,20-Azobis (2-methylpropionamidine) dihydrochloride. | |
3.4 Effects of MH against Aβ-induced paralysis
Aβ aggregation is considered one of the major pathological causes of AD. To evaluate whether MH ameliorated the Aβ-induced toxicity, we used the transgenic C. elegans strain CL4176, which expresses the human Aβ peptide in body wall muscle cells following temperature upshift from 16 to 25 °C, resulting in a paralysis phenotype. As shown in Fig. 3A, non-treated CL4176 worms exhibited almost complete paralysis after 34 h of the temperature upshift, while 100 mg mL−1 MH treatment significantly (p < 0.05) delayed this process. In the MH-treated group, no significant differences (p < 0.05) were observed with respect to the non-paralyzable strain CL802 (negative control) up to 30 h after temperature upshift. At the end point of the analysis, the paralyzed worms represented only 25% of the entire population. In addition, MH significantly reduced Aβ deposits in the tail regions of CL4176 worms as evidenced by thioflavin T staining (Fig. 3B).
 |
| Fig. 3 Effects of Manuka honey on Aβ-induced paralysis. (A) Worm paralysis over time. Data are expressed as mean ± SEM of three independent experiments (n = 3). For each time, different superscript letters among groups indicate statistically significant differences (p < 0.05). (B) Representative images of Thioflavin T aggregates after 30 h of temperature shifting. Aβ aggregates are indicated with white arrows (40× magnification; scale bar, 100 μm). CTL_802: non-paralyzable strain CL802 (negative control); CTL_4176: non-treated worms of the paralyzable strain CL4176 (positive control); MH (100 mg mL−1)_4176: worms of the paralyzable strain CL4176 treated with 100 mg mL−1 of Manuka honey. | |
To further elucidate the molecular mechanisms underlying the protective effects of MH on Aβ toxicity, we repeated the paralysis test in CL4176 worms silencing some genes by RNAi, including sod-2 and sod-3, hsp-16.2, skn-1, and daf-16. 34 h after the temperature upshift, RNAi for sod-3 and daf-16 had no effect on the percentage of non-paralyzed worms compared to the MH-treated group. In contrast, RNAi for hsp-16.2 and skn-1 showed a significantly lower % of non-paralyzed worms, which indicates that there was a reduction in the protective effects of MH on Aβ-induced paralysis, suggesting that these genes are likely implicated in the protective effects of MH (Fig. 4).
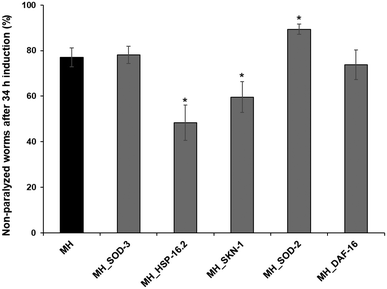 |
| Fig. 4 Effects of different RNAi (sod-3, hsp-16.2, skn-1, sod-2 and daf-16) on the Aβ-induced paralysis after 34 h of induction. Worms of the paralyzable strain CL4176 treated with MH (100 mg mL−1) were used in all groups. Data are expressed as mean ± SEM of three independent experiments (n = 3). Asterisk marks (*) indicate statistically significant differences (p < 0.05) compared to MH (worms non-exposed to RNAi and treated with MH). MH: Manuka honey. | |
3.5 Effects of MH on SOD-3::GFP, GST-4::GFP, SKN-1::GFP, HSP-16.2::GFP and DAF-16::GFP expression
To deepen the comprehension of the effects of MH on the genes knocked down in the experiments described above, we used several transgenic strains carrying the GFP transcriptional reporters of interest. A marked diminution (p < 0.05) in the expression of HSP-16.2::GFP in TJ375 worms treated with MH (by 34.41%) as well as in the expression of SOD-3::GFP in CF1553 nematodes (by 16.30%) was found. No significant effects from MH addition (p < 0.05) were observed on the expression of GFP from the promoters for GST-4, SKN-1 and HSF-1 compared to control. Also, MH did not induce the translocation of DAF-16 from the cytoplasm to nuclei (Fig. 5A and B).
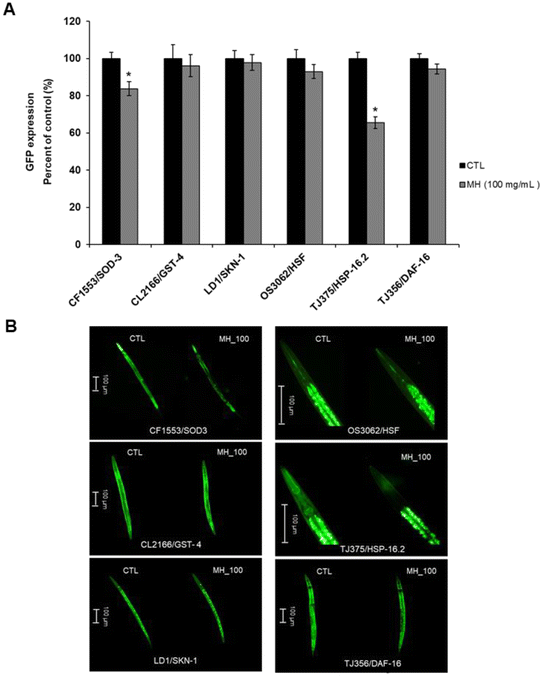 |
| Fig. 5 Effects of Manuka honey on GFP-reporter transgenic strains. (A) Quantification of the GFP expression in the different transgenic transcriptional reporter strains with or without MH treatment (100 mg mL−1). Data are expressed as mean ± SEM of three independent experiments (n = 3). Asterisk marks (*) indicate statistically significant differences (p < 0.05) compared to control (non-treated worms). (B) Representative images for each transgenic strain (CF1553/SOD-3::GFP strain, CL2166/GST-4::GFP strain, LD1/SKN-1::GFP strain, OS3062/HSF::GFP strain, TJ375/HSP-16.2::GFP strain, TJ356/DAF-16::GFP strain); (40× magnification; scale bar, 100 μm). CTL: control; MH: Manuka honey. | |
3.6 Effects of MH on Tau Proteotoxicity
Along with Aβ plaques, the presence of tau protein aggregates is another hallmark of AD. Although the mechanisms of tau-associated neurodegeneration remain unclear, aggregation of this protein is thought to be toxic to neurons. Therefore, the effects of MH on the transgenic strain BR5706 were also evaluated. BR5706 is characterized by accelerated aggregation of insoluble Tau, resulting in severe developmental defects of the nervous system, impaired locomotion, and slowed growth.
Worms treated with 100 mg mL−1 MH showed a significant decrease (p < 0.05) in swimming speed (by 70.76%), wavelength (by 21.68%) and activity (by 39.32%) compared to the control group (Fig. 6A and B). Trying to find an explanation for these results, the contribution of sugars to the assayed parameters was evaluated. For that, two additional experimental groups were included: (i) worms treated with 232 μg mL−1 of a hydroalcoholic extract (ME) only containing the phenolic compounds of MH and (ii) worms treated with 100 mg mL−1 of an artificial honey (AH). AH was prepared by dissolving 22.0 g of maltose, 4.4 g of sucrose, 98.5 g glucose and 119.1 g of fructose in 50 mL of de-ionized water.9 As it can be appreciated in Fig. 6A and B, no significant differences (p < 0.05) were found between the ME-treated group and the control group, while AH addition showed the same worsening effects as MH, leading to 39.43-, 71.21- and 70.51-fold diminution of the swimming speed, wavelength, and activity, respectively, compared to the control.
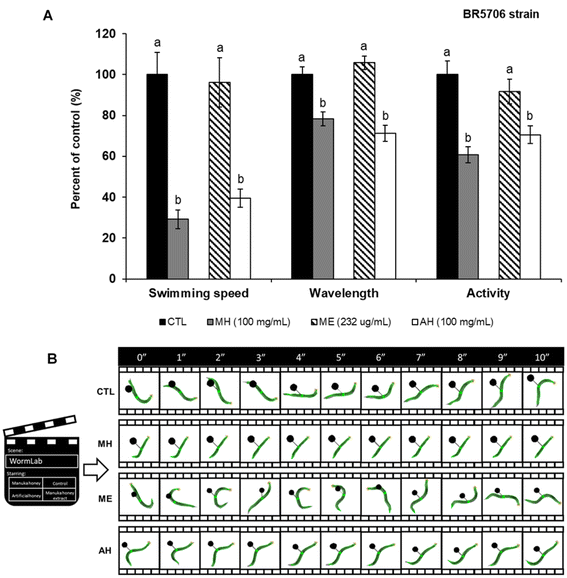 |
| Fig. 6 Effects of Manuka honey on Tau-induced altered locomotive behavioral phenotype. The BR5706 transgenic strain was used in all groups. (A) Swimming speed, wavelength and activity. Data are expressed as mean ± SEM of three independent experiments (n = 3). Different superscript letters for the same set of data indicate statistically significant differences (P < 0.05) compared to control (non-treated worms). (B) Image sequence illustrating how the nematodes move from left to right in the experimental groups. Images were obtained from frame-by-frame tracking data provided by the Wormlab system/software. CTL: control; MH: Manuka honey, ME: Manuka honey hydroalcoholic extract, AH: artificial honey. | |
To further determine whether the observed effects were directly related to Tau protein aggregation, the mobility assay was performed in the wild type N2 strain (Fig. 7A and B). Also in this case, MH decreased swimming speed, wavelength, and activity by 54.92, 28.96 and 22.32%, respectively, compared to non-treated nematodes. Considering these data, it can be suggested that the sugar content of MH affects the general mobility of C. elegans and not necessarily the aggregation of the Tau protein. However, future analyzes are needed to corroborate this hypothesis.
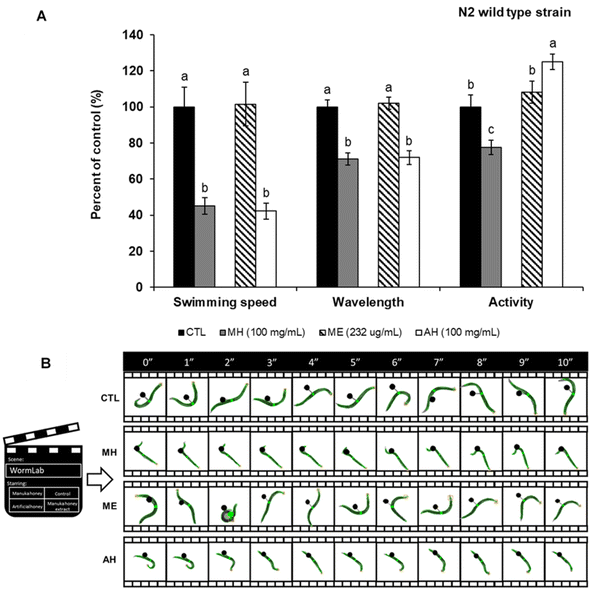 |
| Fig. 7 Effects of Manuka honey on locomotive behavior in wild-type C. elegans. Wild type-N2 strain was used in all groups. (A) Swimming speed, wavelength, and activity. Data are expressed as mean ± SEM of three independent experiments (n = 3). Different superscript letters for the same set of data indicate statistically significant differences (P < 0.05) compared to control (non-treated worms). (B) Image sequence illustrating how the nematodes move from left to right in the experimental groups. Images were obtained from frame-by-frame tracking data provided by the Wormlab system/software. CTL: control; MH: Manuka honey, ME: Manuka honey hydroalcoholic extract, AH: artificial honey. | |
4. Discussion
Despite the fact that MH is a natural product with remarkable biological properties both in vitro8–11,36–38 and in vivo,39,40 little is known about its neuroprotective potential. Considering its ability to scavenge free radicals and the implication of oxidative stress in the pathogenesis of age-related diseases such as AD, we decided to investigate the possible effects of MH in alleviating Aβ and Tau-induced toxicity in C. elegans models of AD.
The qualitative profile of MH phenolic compounds determined by UPLC-TOF-MS/MS was similar to that found by other authors.8,9,11,41,42 Nevertheless, we were unable to quantify some of the most representative compounds reported for this honey, such as syringic acid,11 methyl syringate,9,41,42 pinocembrin and pinobanksin.8,42 It is well known that the extraction, conservation, and detection methods may influence these results. Regarding the TPC and TFC, the values obtained (0.47 ± 0.03 mg GAE g−1 and 0.05 ± 0.01 mg CAE g−1, respectively) were slightly lower than those reported by Y.-Z. Zhang et al.,43 Gośliński et al.,44 Marshall et al.,45 and Anand et al.46 However, the TAC values (2.65 ± 0.10 μM TE g−1 by FRAP; 1.40 ± 0.13 μM TE g−1 by TEAC and 4.58 ± 0.22 μM TE g−1 by DPPH) were quite similar to those reported by Sadia Afrin et al.10 and Marshall et al.45
Besides that, 100 mg mL−1 MH did not show short term toxicity in the C. elegans nematodes as demonstrated by the results of the lethality, pharyngeal pumping and egg-laying (fertility) assays. Next, we also evaluated the effects of MH against AAPH-induced oxidative stress, since multiple lines of evidence indicate that ROS overproduction and consequently, an oxidative stress escalation, not only contributes to the aging process4,47 but is also one of the main factors involved in the initiation and progression of neurodegenerative diseases.48 In the particular case of AD, oxidative stress is related to Aβ aggregation4,15 and the phosphorylation and polymerization of Tau, two hallmarks of the disease.4 Here, we demonstrated that MH increased resistance to stress conditions as revealed by the DCF-DA method. In similar studies, echinacoside, a phenylethanoid glycoside from Cistanche deserticola,47 carnosic acid,3 cannabidiol,48 gennianchun, a Chinese herbal formula7 and caffeic acid4 reduced ROS levels in the wild-type C. elegans strain.
In addition to the ROS theory of AD, there are multiple hypotheses about AD etiology, including the (i) tau protein hyperphosphorylation hypothesis, (ii) mitochondrial function hypothesis, (iii) cholinergic hypothesis and (iv) amyloid cascade hypothesis.3 Although the topic is the subject of debate, the amyloid cascade hypothesis, which suggests that Aβ deposition triggers a series of events (microglial activation, inflammatory response, reactive astrocytosis)49 that lead to neuronal dysfunction, is the most widely accepted.3,7,49,50 Consequently, alleviating Aβ accumulation has been considered one of the most promising therapeutic approaches for AD.48 To evaluate whether MH was able to delay the Aβ-induced neurotoxicity, we conducted a paralysis test in the transgenic strain CL4176, which has been widely used to study this process because of its ability to express human Aβ peptide in nematode body wall muscle and develop a progressive and reproducible paralysis phenotype.2,6,13,51 Our results showed that MH significantly delayed the paralysis of CL4176 worms caused by Aβ aggregation in response to the temperature upshift and reduced Aβ deposits as evidenced by thioflavin T staining. It would be interesting, for future research, to assess whether MH, in addition to reducing the aggregation of the Aβ peptide, is capable of reducing Aβ oligomers, since it is well known that they are more toxic than Aβ monomers and have been related to memory loss and severity of dementia13,52 Aβ oligomers may damage neurons by interfering with neuron-to-neuron communication at synapses.5 Many other natural compounds, such as betaine,49 Ginkgo biloba extract,7 gennianchun,7Sideritis scardica extracts,53 fucoidan,54 ergothioneine,55 frondoside A,56 spermidine,57 essential Oil of Acorus tatarinowii Schott,58 allophycocyanin,50 caffeic acid,4 strawberry extract,15 carnosic acid,3 scutellarein,59 oleuropein,17 hydroxytyrosol,16,59 and red-beet betalain pigments,60 also protect C. elegans nematodes against Aβ-induced toxicity.
Most of the studies evaluating the mechanism underlying the effect of natural compounds against Aβ-induced paralysis in C. elegans, focus on pathways related to oxidative stress, heat shock response, insulin signaling cascade and redox homeostasis.51 Hence, we knocked down the expression of SOD-2 and SOD-3, HSP-16.2, SKN-1, and DAF-16 genes by RNAi technology and repeated the paralysis test in the CL4176 strain.
DAF-16 is the only homologue of the forkhead box transcription factors class O (FOXO) in C. elegans and the main output of the insulin/insulin-like growth factor 1 (IGF-1) signaling (IIS) pathway. Therefore, it plays a fundamental role in the regulation of growth, aging, metabolism, development, reproduction, stress resistance and immunity.7,52,61,62 DAF-16 activation increases the expression of multiple genes involved in the antioxidant response, including the metallothionein homologue (mtl-1), mitochondrial superoxide dismutase (sod-3), the catalase genes ctl-1 and ctl-2 and some small heat-shock protein (HSPs) genes.7,62–64 Moreover, the response against oxidative stress is also mediated by the joint action of other factors such as SKN-1, SMK-1 (a regulatory subunit of the PP4 protein phosphatase complex) and HSF-1.62 Up to the present, it has been reported that several compounds extend lifespan and reduce Aβ-induced toxicity in C. elegans via DAF-16, as for example royal jelly,65 monascin,66 dianxianning,52 gengnianchun,7 phosphatidylcholine,64 Zijuan Pu'er tea extract,67Hibiscus sabdariffa L. extract,68 strawberry extract,15 oleuropein,17Moringa oleífera extract,69 and hydroxytyrosol.16 However, under our experimental conditions, DAF-16 and SOD-3 RNAi failed to delay the Aβ-induced paralysis, indicating that MH attenuation of neurotoxicity is not mediated by these pathways. On the contrary, SKN-1 and HSP-16.2 were required for MH protective effects. Zhu et al.70 also demonstrated that a rose essential oil was protective in a C. elegans model of Aβ-toxicity through SKN-1 activation but independent of DAF-16. Other compounds that have been shown to ameliorate Aβ-induced toxicity through the SKN-1 pathway are Hibiscus sabdariffa L. extract,68 Panax Notoginseng saponins,71 strawberry extract,15 and Cratoxylum formosum extract.72
SKN-1 is a transcription factor that can act in parallel to DAF-16, that can also inhibit the Aβ-induced toxicity by promoting the expression of stress responsive genes.52 This transcriptional factor is analogous to mammalian Nrf2 (nuclear factor erythroid 2-related factor 2) protein, which induce phase 2 detoxification genes regulating both, the xenobiotic and antioxidant response.50,70–72 For its part, the overexpression of the chaperone HSP-16.2 may improve the Aβ associated toxicity by increasing abnormal protein degradation, sequestration and refolding.52,71 Guarana (Paullinia cupana Mart.)73 and palmatine74 also act through this mechanism. In correspondence with the RNAi results, MH did not cause the translocation of DAF-16 from the cytoplasm to nuclei in the transgenic strain TJ356 carrying a GFP-tagged reporter of this gene. In addition, MH did not cause any change in the expression of SKN-1 compared to the control group and it decreased the expression of HSP-16.2 in the transgenic strains LD1 and TJ375, respectively, suggesting that MH effects on Aβ-paralysis mediated by these genes occurs only under stress conditions and not in a basal state. Likewise, MH did not increase the expression of GST-4, SOD-2 and SOD-3, which are target genes of DAF-16 or SKN-1 implicated in ROS elimination, suggesting that the intrinsic antioxidant capacity of MH is essential for its striking effects on the oxidative stress resistance and attenuation of Aβ-toxicity.
As mentioned before, neurofibrillary tangles represent another distinctive feature of AD. Although the relationship between Aβ-peptides and Tau accumulation is not yet well understood, some studies have indicated that both proteins act synergistically in promoting the pathological progression of AD and neuronal loss.75–78 Thus, we also evaluated the effect of MH against Tau-induced neurotoxicity, observing that 100 mg mL−1 MH was not able to improve the locomotion defects related to Tau protein accumulation in the transgenic strain BR5706 nor in N2 nematodes. In fact, MH-treated worms showed significantly lower swimming speed, wavelength, and activity compared to the control group.
Depending on the growth media, C. elegans can swim, burrow and crawl79 and according to the Wormlab software used in this study, the swimming speed refers to the distance covered by the worm along its central axis over a two-stroke interval, whereas activity represents the brush stroke normalized by the time taken to perform the two strokes. For its part, the wavelength denotes the distance between negative and positive inflection points. This parameter is approximately twice the body length, generating alternating C-shapes with each ventral or dorsal muscle contraction.79 Thus, the effects observed in terms of worm mobility are in correspondence with the length diminution observed in the MH-treated groups. Considering that no differences were observed between MH and AH but with the ME (only containing the phenolic fraction of honey), we ascribed the deleterious effects of MH on locomotion to the sugar content of this matrix. Moreover, since the results were similar in both BR5706 and wild-type strain, we assume that the sugar effect is not related to Tau aggregation but directly to mobility. However, further analyzes are needed in this direction.
5. Conclusions
In this report it has been demonstrated that MH improved tolerance to oxidative stress induced by AAPH in the wild-type N2 C. elegans strain, and alleviated Aβ-induced paralysis in the AD model CL4176 through HSP-16.2 and SKN-1/Nrf2 pathways. Likewise, it has been shown that the sugar content of MH impaired the indicators of locomotion in both, the transgenic strain BR5706 and in the wild-type N2 worms but did not influence the rest of the favorable effects described above. Results presented here indicated that the antioxidant activity of MH may be responsible for the observed neuroprotective effects.
Abbreviations
AAPH | (2,2′-Azobis-2-amidinopropane dihydrochloride) |
AD | Alzheimer's disease |
AH | Artificial honey |
Aβ | Amyloid-β |
C. elegans
|
Caenorhabditis elegans
|
DAF-16 | Dauer formation |
DPPH | 2,4-DNP; 2,2-diphenyl-1-picrylhydrazyl |
FOXO | Forkhead box transcription factors class O |
FRAP | Ferric reducing antioxidant power |
GFP | Green fluorescence protein |
GST-4 | Glutathione S-transferase 4 |
HSF1 | Heat-shock transcription factor 1 |
HSP-16.2 | Heat shock protein 16.2 |
HSPs | Heat-shock protein |
IIS | Insulin/insulin-like growth factor 1 (IGF-1) signaling |
IPTG | Isopropyl β-D-1-thiogalactopyranoside |
ME | Hydroalcoholic extract |
MH | Manuka honey |
NGM | Nematode growth medium |
Nrf-2 | Nuclear factor erythroid 2-related factor 2 |
ROS | Reactive oxygen species |
SKN-1 | Transcription factor skinhead-1 |
SOD | Superoxide dismutase |
TE | Trolox equivalents |
TEAC | Trolox equivalent antioxidant capacity |
TFC | Total flavonoids content |
TOF | Time of flight |
TPC | Total phenolic compounds |
Author contributions
Conceptualization, J. L. Q. and T. Y. F.-H.; data curation, F. G.; formal analysis, investigation and methodology, M. D. N.-H., J. M. R.-M, P. M.-O, V. J.-T., A. E.-M., K. T., L. R.-G., C. S.-G. and J. L.; funding acquisition, J. L. Q., M. B. and C. S.-G.; supervision, J. L. Q and T. Y. F.-H.; writing—original draft, M. D. N.-H. and T. Y. F.-H; writing—reviewing & editing; M. D. N.-H, T. Y. F.-H., M. B., C. S.-G., J. L. and J. L. Q. All authors have read and agreed to the published version of the manuscript.
Conflicts of interest
The authors declare no conflict of interest.
Acknowledgements
María D. Navarro-Hortal and José M. Romero-Márquez are FPU fellows with grant reference FPU2017/04358 and FPU2018/05301, respectively, funded by MCIN/AEI/10.13039/501100011033 and FSE “El FSE invierte en tu futuro”. Tamara Forbes-Hernández is supported by a JdC-I post-doctoral contract with grant reference IJC2020-043910-I, funded by NextGenerationEU.
The authors gratefully acknowledge the funding support of FEDER/Junta de Andalucía-Consejería de Economía y Conocimiento, Grant B-AGR-193-UGR18.
References
- P. Maiti, J. Manna, Z. N. Burch, D. B. Flaherty, J. D. Larkin and G. L. Dunbar, Ameliorative Properties of Boronic Compounds in In Vitro and In Vivo Models of Alzheimer's Disease, Int. J. Mol. Sci., 2020, 21(18), 6664, DOI:10.3390/ijms21186664.
- Z. Wang, P. Zheng, Y. Xie, X. Chen, N. Solowij, K. Green, Y. L. Chew and X.-F. Huang, Cannabidiol Regulates CB1-PSTAT3 Signaling for Neurite Outgrowth, Prolongs Lifespan, and Improves Health Span in Caenorhabditis Elegans of Aβ Pathology Models, FASEB J., 2021, 35(5), e21537, DOI:10.1096/fj.202002724R.
- Y. Chen, Y. Wang, Q. Qin, Y. Zhang, L. Xie, J. Xiao, Y. Cao, Z. Su and Y. Chen, Carnosic Acid Ameliorated Aβ-Mediated (Amyloid-β Peptide) Toxicity, Cholinergic Dysfunction and Mitochondrial Defect in Caenorhabditis Elegans of Alzheimer's Model, Food Funct., 2022, 13(8), 4624–4640, 10.1039/D1FO02965G.
- H. Li, X. Yu, C. Li, L. Ma, Z. Zhao, S. Guan and L. Wang, Caffeic Acid Protects against Aβ Toxicity and Prolongs Lifespan in Caenorhabditis Elegans Models, Food Funct., 2021, 12(3), 1219–1231, 10.1039/D0FO02784G.
- Alzheimer's Association, 2022 Alzheimer's Disease Facts and Figures, Alzheimer's Dementia, 2022, 18(4), 700–789, DOI:10.1002/alz.12638.
- Y. Luo, Alzheimer's Disease, the Nematode Caenorhabditis Elegans, and Ginkgo Biloba Leaf Extract, Life Sci., 2006, 78(18), 2066–2072, DOI:10.1016/j.lfs.2005.12.004.
- F. Meng, J. Li, Y. Rao, W. Wang and Y. Fu, A Chinese Herbal Formula, Gengnianchun, Ameliorates β-Amyloid Peptide Toxicity in a Caenorhabditis Elegans Model of Alzheimer's Disease, Evid.-Based Complement. Altern. Med., 2017, 2017, 7480980, DOI:10.1155/2017/7480980.
- J. M. Alvarez-Suarez, M. Gasparrini, T. Y. Forbes-Hernández, L. Mazzoni and F. Giampieri, The Composition and Biological Activity of Honey: A Focus on Manuka Honey, Foods, 2014, 3(3), 420–432, DOI:10.3390/foods3030420.
- J. M. Alvarez-Suarez, F. Giampieri, M. Cordero, M. Gasparrini, T. Y. Forbes-Hernández, L. Mazzoni, S. Afrin, P. Beltrán-Ayala, A. M. González-Paramás, C. Santos-Buelga, A. Varela-Lopez, J. L. Quiles and M. Battino, Activation of AMPK/Nrf2 Signalling by Manuka Honey Protects Human Dermal Fibroblasts against Oxidative Damage by Improving Antioxidant Response and Mitochondrial Function Promoting Wound Healing, J. Funct. Foods, 2016, 25, 38–49, DOI:10.1016/j.jff.2016.05.008.
- S. Afrin, M. Gasparrini, T. Y. Forbes-Hernández, D. Cianciosi, P. Reboredo-Rodriguez, P. P. Manna, M. Battino and F. Giampieri, Protective Effects of Manuka Honey on LPS-Treated RAW 264.7 Macrophages. Part 1: Enhancement of Cellular Viability, Regulation of Cellular Apoptosis and Improvement of Mitochondrial Functionality, Food Chem. Toxicol., 2018, 121, 203–213, DOI:10.1016/j.fct.2018.09.001.
- S. Afrin, F. Giampieri, M. Gasparrini, T. Y. Forbes-Hernández, D. Cianciosi, P. Reboredo-Rodriguez, A. Amici, J. L. Quiles and M. Battino, The Inhibitory Effect of Manuka Honey on Human Colon Cancer HCT-116 and LoVo Cell Growth. Part 1: The Suppression of Cell Proliferation, Promotion of Apoptosis and Arrest of the Cell Cycle, Food Funct., 2018, 9(4), 2145–2157, 10.1039/c8fo00164b.
- S. Afrin, F. Giampieri, T. Forbes-Hernández, M. Gasparrini, A. Amici, D. Cianciosi, J. Quiles and M. Battino, Manuka Honey Synergistically Enhances the Chemopreventive Effect of 5-fluorouracil on Human Colon Cancer Cells by Inducing Oxidative Stress and Apoptosis, Altering Metabolic Phenotypes and Suppressing Metastasis Ability, Free Radicals Biol. Med., 2018, 126, 41–54, DOI:10.1016/j.freeradbiomed.2018.07.014.
- A. Lublin and C. Link, Alzheimer's Disease Drug Discovery: In vivo Screening Using C. Elegans as a Model for β-Amyloid Peptide-Induced Toxicity, Drug Discovery Today: Technol., 2013, 10(1), e115–e119, DOI:10.1016/j.ddtec.2012.02.002.
- K. Slinkard and V. L. Singleton, Total Phenol Analysis: Automation and Comparison with Manual Methods, Am. J. Enol. Vitic., 1977, 28(1), 49–55 CAS.
- M. D. Navarro-Hortal, J. M. Romero-Márquez, A. Esteban-Muñoz, C. Sánchez-González, L. Rivas-García, J. Llopis, D. Cianciosi, F. Giampieri, S. Sumalla-Cano, M. Battino and J. L. Quiles, Strawberry (Fragaria × Ananassa Cv. Romina) Methanolic Extract Attenuates Alzheimer's Beta Amyloid Production and Oxidative Stress by SKN-1/NRF and DAF-16/FOXO Mediated Mechanisms in C. Elegans, Food Chem., 2022, 372, 131272, DOI:10.1016/j.foodchem.2021.131272.
- J. M. Romero-Márquez, M. D. Navarro-Hortal, V. Jiménez-Trigo, P. Muñoz-Ollero, T. Y. Forbes-Hernández, A. Esteban-Muñoz, F. Giampieri, I. Delgado Noya, P. Bullón, L. Vera-Ramírez, M. Battino, C. Sánchez-González and J. L. Quiles, An Olive-Derived Extract 20% Rich in Hydroxytyrosol Prevents β-Amyloid Aggregation and Oxidative Stress, Two Features of Alzheimer Disease, via SKN-1/NRF2 and HSP-16.2 in Caenorhabditis Elegans, Antioxidants, 2022, 11(4), 629, DOI:10.3390/antiox11040629.
- J. M. Romero-Márquez, M. D. Navarro-Hortal, V. Jiménez-Trigo, L. Vera-Ramírez, T. J. Forbes-Hernández, A. Esteban-Muñoz, F. Giampieri, P. Bullón, M. Battino, C. Sánchez-González and J. L. Quiles, An Oleuropein Rich-Olive (Olea Europaea L.) Leaf Extract Reduces β-Amyloid and Tau Proteotoxicity through Regulation of Oxidative- and Heat Shock-Stress Responses in Caenorhabditis Elegans, Food Chem. Toxicol., 2022, 162, 112914, DOI:10.1016/j.fct.2022.112914.
- A. Esteban-Muñoz, S. Sánchez-Hernández, C. Samaniego-Sánchez, R. Giménez-Martínez and M. Olalla-Herrera, Differences in the Phenolic Profile by UPLC Coupled to High Resolution Mass Spectrometry and Antioxidant Capacity of Two Diospyros Kaki Varieties, Antioxidants, 2021, 10(1), 31, DOI:10.3390/antiox10010031.
- S. Sánchez-Hernández, A. Esteban-Muñoz, C. Samaniego-Sánchez, R. Giménez-Martínez, B. Miralles and M. Olalla-Herrera, Study of the Phenolic Compound Profile and Antioxidant Activity of Human Milk from Spanish Women at Different Stages of Lactation: A Comparison with Infant Formulas, Food Res. Int., 2021, 141, 110149, DOI:10.1016/j.foodres.2021.110149.
- B. d. Silva, L. Valdomiro Gonzaga, R. Fett and A. C. Oliveira Costa, Simplex-Centroid Design and Derringer's Desirability Function Approach for Simultaneous Separation of Phenolic Compounds from Mimosa Scabrella Bentham Honeydew Honeys by HPLC/DAD, J. Chromatogr. A, 2019, 1585, 182–191, DOI:10.1016/j.chroma.2018.11.072.
- N. Stanek, P. Kafarski and I. Jasicka-Misiak, Development of a High Performance Thin Layer Chromatography Method for the Rapid Qualification and Quantification of Phenolic Compounds and Abscisic Acid in Honeys, J. Chromatogr. A, 2019, 1598, 209–215, DOI:10.1016/j.chroma.2019.04.052.
- M. Biesaga and K. Pyrzynska, Liquid Chromatography/Tandem Mass Spectrometry Studies of the Phenolic Compounds in Honey, J. Chromatogr. A, 2009, 1216(38), 6620–6626, DOI:10.1016/j.chroma.2009.07.066.
- M. Nešović, U. Gašić, T. Tosti, J. Trifković, R. Baošić, S. Blagojević, L. Ignjatović and Ž Tešić, Physicochemical Analysis and Phenolic Profile of Polyfloral and Honeydew Honey from Montenegro, RSC Adv., 2020, 10(5), 2462–2471, 10.1039/C9RA08783D.
- Z. Zhu, Y. Zhang, J. Wang, X. Li, W. Wang and Z. Huang, Sugaring-out Assisted Liquid-Liquid Extraction Coupled with High Performance Liquid Chromatography-Electrochemical Detection for the Determination of 17 Phenolic Compounds in Honey, J. Chromatogr. A, 2019, 1601, 104–114, DOI:10.1016/j.chroma.2019.06.023.
- S. Kečkeš, U. Gašić, T.Ć Veličković, D. Milojković-Opsenica, M. Natić and Ž Tešić, The Determination of Phenolic Profiles of Serbian Unifloral Honeys Using Ultra-High-Performance Liquid Chromatography/High Resolution Accurate Mass Spectrometry, Food Chem., 2013, 138(1), 32–40, DOI:10.1016/j.foodchem.2012.10.025.
- C. T. Ciucure and E.-I. Geană, Phenolic Compounds Profile and Biochemical Properties of Honeys in Relationship to the Honey Floral Sources, Phytochem. Anal., 2019, 30(4), 481–492, DOI:10.1002/pca.2831.
- J. Rusko, P. Vainovska, B. Vilne and V. Bartkevics, Phenolic Profiles of Raw Mono- and Polyfloral Honeys from Latvia, J. Food Compos. Anal., 2021, 98, 103813, DOI:10.1016/j.jfca.2021.103813.
- F. J. Leyva-Jimenez, J. Lozano-Sanchez, I. Borras-Linares, M. d. l. L. Cadiz-Gurrea and E. Mahmoodi-Khaledi, Potential Antimicrobial Activity of Honey Phenolic Compounds against Gram positive and Gram negative Bacteria, LWT–Food Sci. Technol., 2019, 101, 236–245, DOI:10.1016/j.lwt.2018.11.015.
- M. A. Boukhris, É. Destandau, A. El Hakmaoui, L. El Rhaffari and C. Elfakir, A Dereplication Strategy for the Identification of New Phenolic Compounds from Anvillea Radiata (Coss. & Durieu), C. R. Chim., 2016, 19(9), 1124–1132, DOI:10.1016/j.crci.2016.05.019.
- Y. Cheung, M. Meenu, X. Yu and B. Xu, Phenolic Acids and Flavonoids Profiles of Commercial Honey from Different Floral Sources and Geographic Sources, Int. J. Food Prop., 2019, 22(1), 290–308, DOI:10.1080/10942912.2019.1579835.
- A. Juric, U. Gasic, I. Brcic-Karaconji, K. Jurica and D. Milojkovic-Opsenica, The Phenolic Profile of Strawberry Tree (Arbutus Unedo L.) Honey, J. Serb. Chem. Soc., 2020, 85(8), 1011–1019, DOI:10.2298/JSC191217018J.
- F. Braghini, F. C. Biluca, L. V. Gonzaga, A. S. Kracik, C. R. W. Vieira, L. Vitali, G. A. Micke, A. C. O. Costa and R. Fett, Impact of Short-Term Thermal Treatment on Stingless Bee Honey (Meliponinae): Quality, Phenolic Compounds and Antioxidant Capacity, J. Food Process. Preserv., 2019, 43(7), e13954, DOI:10.1111/jfpp.13954.
- K. Kozłowicz, R. Różyło, B. Gładyszewska, A. Matwijczuk, G. Gładyszewski, D. Chocyk, K. Samborska, J. Piekut and M. Smolewska, Identification of Sugars and Phenolic Compounds in Honey Powders with the Use of GC-MS, FTIR Spectroscopy, and X-Ray Diffraction, Sci. Rep., 2020, 10(1), 16269, DOI:10.1038/s41598-020-73306-7.
- V. Vasić, U. Gašić, D. Stanković, D. Lušić, D. Vukić-Lušić, D. Milojković-Opsenica, Ž Tešić and J. Trifković, Towards Better Quality Criteria of European Honeydew Honey: Phenolic Profile and Antioxidant Capacity, Food Chem., 2019, 274, 629–641, DOI:10.1016/j.foodchem.2018.09.045.
- I. Sergiel, P. Pohl and M. Biesaga, Characterisation of Honeys According to Their Content of Phenolic Compounds Using High Performance Liquid Chromatography/Tandem Mass Spectrometry, Food Chem., 2014, 145, 404–408, DOI:10.1016/j.foodchem.2013.08.068.
- D. Cianciosi, T. Y. Forbes-Hernández, L. Regolo, J. M. Alvarez-Suarez, D. Quinzi, A. Sargenti, W. Bai, L. Tian, F. Giampieri and M. Battino, Manuka Honey in Combination with 5-Fluorouracil Decreases Physical Parameters of Colonspheres Enriched with Cancer Stem-like Cells and Reduces Their Resistance to Apoptosis, Food Chem., 2022, 374, 131753, DOI:10.1016/j.foodchem.2021.131753.
- G. Hayes, N. Wright, S. L. Gardner, C. L. Telzrow, A. J. Wommack and P. A. Vigueira, Manuka Honey and Methylglyoxal Increase the Sensitivity of Staphylococcus Aureus to Linezolid, Lett. Appl. Microbiol., 2018, 66(6), 491–495, DOI:10.1111/lam.12880.
- H. R. Al Refaey, A.-S. A. Newairy, M. M. Wahby, C. Albanese, M. Elkewedi, M. U. Choudhry and A. S. Sultan, Manuka Honey Enhanced Sensitivity of HepG2, Hepatocellular Carcinoma Cells, for Doxorubicin and Induced Apoptosis through Inhibition of Wnt/β-Catenin and ERK1/2, Biol. Res., 2021, 54(1), 16, DOI:10.1186/s40659-021-00339-1.
- Z. Jubri, N. B. A. Rahim and G. J. Aan, Manuka Honey Protects Middle-Aged Rats from Oxidative Damage, Clinics, 2013, 68(11), 1446–1454, DOI:10.6061/clinics/2013(11)11.
- S. B. Almasaudi, A. T. Abbas, R. R. Al-Hindi, N. A. El-Shitany, U. A. Abdel-Dayem, S. S. Ali, R. M. Saleh, S. K. Al Jaouni, M. A. Kamal and S. M. Harakeh, Manuka Honey Exerts Antioxidant and Anti-Inflammatory Activities That Promote Healing of Acetic Acid-Induced Gastric Ulcer in Rats, Evid.-Based Complement. Altern. Med., 2017, 2017, 5413917, DOI:10.1155/2017/5413917.
-
M. Senanayake, A Chemical Investigation of New Zealand Unifloral Honeys, Thesis, The University of Waikato, 2006 Search PubMed.
- S. Oelschlaegel, M. Gruner, P.-N. Wang, A. Boettcher, I. Koelling-Speer and K. Speer, Classification and Characterization of Manuka Honeys Based on Phenolic Compounds and Methylglyoxal, J. Agric. Food Chem., 2012, 60(29), 7229–7237, DOI:10.1021/jf300888q.
- Y.-Z. Zhang, J.-J. Si, S.-S. Li, G.-Z. Zhang, S. Wang, H.-Q. Zheng and F.-L. Hu, Chemical Analyses and Antimicrobial Activity of Nine Kinds of Unifloral Chinese Honeys Compared to Manuka Honey (12+ and 20+), Molecules, 2021, 26(9), 2778, DOI:10.3390/molecules26092778.
- M. Gośliński, D. Nowak and L. Kłębukowska, Antioxidant Properties and Antimicrobial Activity of Manuka Honey versus Polish Honeys, J. Food Sci. Technol., 2020, 57(4), 1269–1277, DOI:10.1007/s13197-019-04159-w.
- S. M. Marshall, K. R. Schneider, K. V. Cisneros and L. Gu, Determination of Antioxidant Capacities, α-Dicarbonyls, and Phenolic Phytochemicals in Florida Varietal Honeys Using HPLC-DAD-ESI-MSn, J. Agric. Food Chem., 2014, 62(34), 8623–8631, DOI:10.1021/jf501329y.
- S. Anand, E. Pang, G. Livanos and N. Mantri, Characterization of Physico-Chemical Properties and Antioxidant Capacities of Bioactive Honey Produced from Australian Grown Agastache Rugosa and Its Correlation with Colour and Poly-Phenol Content, Molecules, 2018, 23(1), 108, DOI:10.3390/molecules23010108.
- W. Chen, H.-R. Lin, C.-M. Wei, X.-H. Luo, M.-L. Sun, Z.-Z. Yang, X.-Y. Chen and H.-B. Wang, Echinacoside, a Phenylethanoid Glycoside from Cistanche Deserticola, Extends Lifespan of Caenorhabditis Elegans and Protects from Aβ-Induced Toxicity, Biogerontology, 2018, 19(1), 47–65, DOI:10.1007/s10522-017-9738-0.
- Y. Zhang, H. Li, S. Jin, Y. Lu, Y. Peng, L. Zhao and X. Wang, Cannabidiol Protects against Alzheimer's Disease in C. Elegans via ROS Scavenging Activity of Its Phenolic Hydroxyl Groups, Eur. J. Pharmacol., 2022, 919, 174829, DOI:10.1016/j.ejphar.2022.174829.
- A. Leiteritz, B. Dilberger, U. Wenzel and E. Fitzenberger, Betaine Reduces β-Amyloid-Induced Paralysis through Activation of Cystathionine-β-Synthase in an Alzheimer Model of Caenorhabditis Elegans, Genes Nutr., 2018, 13, 21, DOI:10.1186/s12263-018-0611-9.
- M. G. Chaubey, S. N. Patel, R. P. Rastogi, D. Madamwar and N. K. Singh, Cyanobacterial Pigment Protein Allophycocyanin Exhibits Longevity and Reduces Aβ-Mediated Paralysis in C. Elegans: Complicity of FOXO and NRF2 Ortholog DAF-16 and SKN-1, 3 Biotech, 2020, 10(8), 332, DOI:10.1007/s13205-020-02314-1.
- X. Zhang, C. Ma, L. Sun, Z. He, Y. Feng, X. Li, J. Gan and X. Chen, Effect of Policosanol from Insect Wax on Amyloid β-Peptide-Induced Toxicity in a Transgenic Caenorhabditis Elegans Model of Alzheimer's Disease, BMC Complement. Med. Ther., 2021, 21, 103, DOI:10.1186/s12906-021-03278-2.
- D. Zhi, D. Wang, W. Yang, Z. Duan, S. Zhu, J. Dong, N. Wang, N. Wang, D. Fei, Z. Zhang, X. Wang, M. Wang and H. Li, Dianxianning Improved Amyloid β-Induced Pathological Characteristics Partially through DAF-2/DAF-16 Insulin like Pathway in Transgenic C. Elegans, Sci. Rep., 2017, 7, 11408, DOI:10.1038/s41598-017-11628-9.
- F. Heiner, B. Feistel and M. Wink, Sideritis Scardica Extracts Inhibit Aggregation and Toxicity of Amyloid-β in Caenorhabditis Elegans Used as a Model for Alzheimer's Disease, PeerJ, 2018, 6, e4683, DOI:10.7717/peerj.4683.
- X. Wang, K. Yi and Y. Zhao, Fucoidan Inhibits Amyloid-β-Induced Toxicity in Transgenic Caenorhabditis Elegans by Reducing the Accumulation of Amyloid-β and Decreasing the Production of Reactive Oxygen Species, Food Funct., 2018, 9(1), 552–560, 10.1039/C7FO00662D.
- I. K. Cheah, L.-T. Ng, L.-F. Ng, V. Y. Lam, J. Gruber, C. Y. W. Huang, F.-Q. Goh, K. H. C. Lim and B. Halliwell, Inhibition of Amyloid-Induced Toxicity by Ergothioneine in a Transgenic Caenorhabditis Elegans Model, FEBS Lett., 2019, 593(16), 2139–2150, DOI:10.1002/1873-3468.13497.
- T. Tangrodchanapong, P. Sobhon and K. Meemon, Frondoside A Attenuates Amyloid-β Proteotoxicity in Transgenic Caenorhabditis Elegans by Suppressing Its Formation, Front. Pharmacol., 2020, 11, 553579, DOI:10.3389/fphar.2020.553579.
- X. Yang, M. Zhang, Y. Dai, Y. Sun, Y. Aman, Y. Xu, P. Yu, Y. Zheng, J. Yang and X. Zhu, Spermidine Inhibits Neurodegeneration and Delays Aging via the PINK1-PDR1-Dependent Mitophagy Pathway in C. Elegans, Aging, 2020, 12(17), 16852–16866, DOI:10.18632/aging.103578.
- X. Chen, D. Liao, M. Sun, X. Cui and H. Wang, Essential Oil of Acorus Tatarinowii Schott Ameliorates Aβ-Induced Toxicity in Caenorhabditis Elegans through an Autophagy Pathway, Oxid. Med. Cell. Longevity, 2020, 2020, 3515609, DOI:10.1155/2020/3515609.
- A. Gea-González, S. Hernández-García, P. Henarejos-Escudero, P. Martínez-Rodríguez, F. García-Carmona and F. Gandía-Herrero, Polyphenols from Traditional Chinese Medicine and Mediterranean Diet Are Effective against Aβ Toxicity in Vitro and in Vivo in Caenorhabditis Elegans, Food Funct., 2022, 13(3), 1206–1217, 10.1039/D1FO02147H.
- T. Imamura, N. Isozumi, Y. Higashimura, H. Koga, T. Segawa, N. Desaka, H. Takagi, K. Matsumoto, S. Ohki and M. Mori, Red-Beet Betalain Pigments Inhibit Amyloid-β Aggregation and Toxicity in Amyloid-β Expressing Caenorhabditis Elegans, Plant Foods Hum. Nutr., 2022, 77(1), 90–97, DOI:10.1007/s11130-022-00951-w.
- H. A. Tissenbaum, DAF-16: FOXO in the Context of C. Elegans, Curr. Top. Dev. Biol., 2018, 127, 1–21, DOI:10.1016/bs.ctdb.2017.11.007.
- A. Zečić and B. P. Braeckman, DAF-16/FoxO in Caenorhabditis Elegans and Its Role in Metabolic Remodeling, Cells, 2020, 9(1), E109, DOI:10.3390/cells9010109.
- C. T. Murphy, S. A. McCarroll, C. I. Bargmann, A. Fraser, R. S. Kamath, J. Ahringer, H. Li and C. Kenyon, Genes That Act Downstream of DAF-16 to Influence the Lifespan of Caenorhabditis Elegans, Nature, 2003, 424(6946), 277–283, DOI:10.1038/nature01789.
- S.-H. Kim, B.-K. Kim, S. Park and S.-K. Park, Phosphatidylcholine Extends Lifespan via DAF-16 and Reduces Amyloid-Beta-Induced Toxicity in Caenorhabditis Elegans, Oxid. Med. Cell. Longevity, 2019, 2019, 2860642, DOI:10.1155/2019/2860642.
- X. Wang, M. Cao and Y. Dong, Royal Jelly Promotes DAF-16-Mediated Proteostasis to Tolerate β-Amyloid Toxicity in C. Elegans Model of Alzheimer's Disease, Oncotarget, 2016, 7(34), 54183–54193, DOI:10.18632/oncotarget.10857.
- Y.-C. Shi, T.-M. Pan and V. H.-C. Liao, Monascin from Monascus-Fermented Products Reduces Oxidative Stress and Amyloid-β Toxicity via DAF-16/FOXO in Caenorhabditis Elegans, J. Agric. Food Chem., 2016, 64(38), 7114–7120, DOI:10.1021/acs.jafc.6b02779.
- F. Du, L. Zhou, Y. Jiao, S. Bai, L. Wang, J. Ma and X. Fu, Ingredients in Zijuan Pu'er Tea Extract Alleviate β-Amyloid Peptide Toxicity in a Caenorhabditis Elegans Model of Alzheimer's Disease Likely through DAF-16, Molecules, 2019, 24(4), E729, DOI:10.3390/molecules24040729.
- K. Koch, N. Weldle, S. Baier, C. Büchter and W. Wätjen, Hibiscus Sabdariffa L. Extract Prolongs Lifespan and Protects against Amyloid-β Toxicity in Caenorhabditis Elegans: Involvement of the FoxO and Nrf2 Orthologues DAF-16 and SKN-1, Eur. J. Nutr., 2020, 59(1), 137–150, DOI:10.1007/s00394-019-01894-w.
- A. P. Chauhan, M. G. Chaubey, S. N. Patel, D. Madamwar and N. K. Singh, Extension of Life Span and Stress Tolerance Modulated by DAF-16 in Caenorhabditis Elegans under the Treatment of Moringa Oleifera Extract, 3 Biotech, 2020, 10(12), 504, DOI:10.1007/s13205-020-02485-x.
- S. Zhu, H. Li, J. Dong, W. Yang, T. Liu, Y. Wang, X. Wang, M. Wang and D. Zhi, Rose Essential Oil Delayed Alzheimer's Disease-Like Symptoms by SKN-1 Pathway in C. Elegans, J. Agric. Food Chem., 2017, 65(40), 8855–8865, DOI:10.1021/acs.jafc.7b03224.
- L. Zhou, P.-P. Huang, L.-L. Chen and P. Wang, Panax Notoginseng Saponins Ameliorate Aβ-Mediated Neurotoxicity in C. Elegans through Antioxidant Activities, Evid.-Based Complement. Altern. Med., 2019, 2019, 7621043, DOI:10.1155/2019/7621043.
- R. Keowkase and N. Weerapreeyakul, Cratoxylum Formosum Extract Protects against Amyloid-Beta Toxicity in a Caenorhabditis Elegans Model of Alzheimer's Disease, Planta Med., 2016, 82(06), 516–523, DOI:10.1055/s-0041-111621.
- D. C. Zamberlan, L. P. Arantes, M. L. Machado, T. L. da Silveira, A. F. da Silva, I. B. M. da Cruz, C. P. Figueiredo and F. A. A. Soares, Guarana (Paullinia Cupana Mart.) Protects against Amyloid-β Toxicity in Caenorhabditis Elegans through Heat Shock Protein Response Activation, Nutr. Neurosci., 2020, 23(6), 444–454, DOI:10.1080/1028415X.2018.1517473.
- W. Jia, Q. Su, Q. Cheng, Q. Peng, A. Qiao, X. Luo, J. Zhang and Y. Wang, Neuroprotective Effects of Palmatine via the Enhancement of Antioxidant Defense and Small Heat Shock Protein Expression in Aβ-Transgenic Caenorhabditis Elegans, Oxid. Med. Cell. Longevity, 2021, 2021, 9966223, DOI:10.1155/2021/9966223.
- S. J. Benbow, T. J. Strovas, M. Darvas, A. Saxton and B. C. Kraemer, Synergistic Toxicity between Tau and Amyloid Drives Neuronal Dysfunction and Neurodegeneration in Transgenic C. Elegans, Hum. Mol. Genet., 2020, 29(3), 495–505, DOI:10.1093/hmg/ddz319.
- T. Miyasaka, C. Xie, S. Yoshimura, Y. Shinzaki, S. Yoshina, E. Kage-Nakadai, S. Mitani and Y. Ihara, Curcumin Improves Tau-Induced Neuronal Dysfunction of Nematodes, Neurobiol. Aging, 2016, 39, 69–81, DOI:10.1016/j.neurobiolaging.2015.11.004.
- G. J. Pir, B. Choudhary and E. Mandelkow, Caenorhabditis Elegans Models of Tauopathy, FASEB J., 2017, 31(12), 5137–5148, DOI:10.1096/fj.201701007.
- D. Paul, S. Chipurupalli, A. Justin, K. Raja and S. K. Mohankumar, Caenorhabditis Elegans as a Possible Model to Screen Anti-Alzheimer's Therapeutics, J. Pharmacol. Toxicol. Methods, 2020, 106, 106932, DOI:10.1016/j.vascn.2020.106932.
- M. Zhen and A. D. T. C. Samuel, Elegans Locomotion: Small Circuits, Complex Functions, Curr. Opin. Neurobiol., 2015, 33, 117–126, DOI:10.1016/j.conb.2015.03.009.
Footnote |
† These authors contributed equally to this manuscript. |
|
This journal is © The Royal Society of Chemistry 2022 |