DOI:
10.1039/D1FO04091J
(Paper)
Food Funct., 2022,
13, 4469-4477
Investigating the effects of supercritical antisolvent process and food models on antioxidant capacity, bioaccessibility and transepithelial transport of quercetin and rutin
Received
1st December 2021
, Accepted 11th March 2022
First published on 14th March 2022
Abstract
In the present study, the effects of the Supercritical Anti-Solvent (SAS) process and food models on the antioxidant capacity, bioaccessibility and transport dynamics of flavonol-loaded polyvinylpyrrolidone (PVP) based microparticles were investigated using a combined in vitro gastrointestinal digestion/Caco-2 cell culture model. SAS-processed and unprocessed flavonols were supplied in two different food models: 10% ethanol for an aqueous hydrophilic food simulant and 3% acetic acid for an acidic food simulant. The SAS processing of quercetin and rutin resulted in a much higher recovery of these bioactives as well as greater retention of antioxidant capacity after gastrointestinal digestion in both hydrophilic and acidic food models. The present study also demonstrates that SAS coprecipitation has a positive effect on the stability and transport of bioactives across the epithelial cell layer. It can be deduced from the results that the SAS process can be a useful method in pharmaceutical and nutraceutical applications with high stability, bioaccessibility, bioavailability and thus enhanced nutritional value.
1. Introduction
Flavonoids are polyphenolic compounds, which are present in a wide variety of foods such as fruits, vegetables, flowers and leaves of plants.1 Quercetin and its glycoside form, namely rutin, have been reported to exhibit a wide range of biological activities, including anticancer, antioxidant, anti-inflammatory and antiviral activities, as well as prevention of cardiovascular, pancreatic and liver diseases.2–5 However, these flavonoids undergo several chemical changes during food processing and storage. Besides, there are also other drawbacks to consider before the incorporation of quercetin and rutin into functional foods, cosmetics and pharmaceuticals; e.g., their crystalline structure at both ambient and body temperatures, poor water solubility, chemical instability in the gastrointestinal tract (GI) and, thus, reduced bioavailability.6–8
Therefore, a wide variety of delivery systems have been investigated to improve the solubility, stability, bioaccessibility and bioavailability of phenolic compounds, including lipid-based formulations (i.e., emulsions/nanoemulsions, solid lipid nanoparticles, nanostructured lipid carriers, liposomes, etc.)9 as well as techniques assisted by supercritical fluids (e.g., RESS, rapid expansion from supercritical solutions; SAS, supercritical antisolvent process; SAA, supercritical assisted atomization process, etc.).10
Considering the above, the purpose of this study was to determine whether quercetin and/or rutin could be successfully protected and released by PVP-based microparticles obtained by the SAS process. Specifically, we have investigated (i) the retention and antioxidant capacity of pure or SAS-processed quercetin and rutin; (ii) the influence of SAS processing on the apical and basolateral recovery as well as the transport efficiency of these flavonols; and (iii) the effects of acidic and hydrophilic food conditions on the stability and bioavailability of the flavonols of interest, using in vitro simulated gastrointestinal digestion, coupled with the human intestinal Caco-2 epithelial cell system as a model. To the best of our knowledge, this is the first in vitro study with simulated human intestinal epithelial cells performed on the flavonols (quercetin and its glycosylated form rutin) embedded into a PVP-based matrix by SAS coprecipitation.
2. Materials and methods
2.1. Materials
Quercetin-polyvinylpyrrolidone (Q-PVP) and rutin-polyvinylpyrrolidone (R-PVP) coprecipitates were fabricated by the SAS process at the University of Salerno (Italy). The Q-PVP and R-PVP microparticles employed in this study were produced using the optimum process conditions described by Ozkan et al.,11 which were as follows: 90 bar, 40 °C, 40 mg mL−1 as overall concentration of solutes (Q/R and PVP) in dimethyl sulfoxide (DMSO), and flavonol/PVP weight ratios equal to 1/20 for Q/PVP and 1/10 for R/PVP.
Polyvinylpyrrolidone (PVP, average molecular weight: 10
000 g mol−1), quercetin dihydrate (purity: 98%), rutin hydrate (purity: 95%), pepsin (EC 3.4.23.1, from porcine gastric mucosa), pancreatin (8× USP, EC 232.468.9, from porcine pancreas, containing trypsin, amylase and lipase) and bile salt were purchased from Sigma-Aldrich (Steinheim, Germany). The Caco-2 human colon adenocarcinoma cell line was obtained from the German Collection of Microorganisms and Cell Cultures (DSMZ, Braunschweig, Germany). Dulbecco's modified Eagle's medium (DMEM), fetal bovine serum (FBS), penicillin and streptomycin, non-essential amino acids (MEM NEAA 100×), and trypsin/EDTA (10×) were purchased from Pan Biotech (Aidenbach, Germany) and trypan blue was obtained from Carl Roth (Karlsruhe, Germany). Ultrapure water was used (Purelab flex 3; Veolia Water Technologies, Celle/Germany). All other reagents were of analytical or HPLC grade.
2.2. Food models
The in vitro digestion experiments were carried out for selected unprocessed flavonols or flavonol-loaded microparticles in two different media, 3% acetic acid and 10% ethanol, which are considered as food simulants for acidic and hydrophilic food products, respectively, according to the Commission Regulation 10/2011 EU (10/2011/EC).12
2.3.
In vitro simulated gastrointestinal digestion
The in vitro digestion procedure was adapted from Sessa et al.13 with some modifications. Due to the daily intake of quercetin being in the range of 0–30 mg,14,15 5 mg of the active compound was selected as the initial dose. Briefly, 5 mg of unprocessed quercetin/rutin or 5 mg of quercetin/rutin equivalent microparticles (105 mg for Q/PVP and 55 mg for R/PVP) were dissolved in 5 mL media (acidic or hydrophilic), individually. A sample of 5 mL from each was placed in a test tube and incubated in a water bath (GFL 1092, Burgwedel, Germany) at 37 °C for 15 min. Due to the fact that the analyzed samples did not contain any starch, we did not perform the oral phase test by using α-amylase. To simulate gastric digestion, the pH was adjusted to 2 using 1 M HCl and porcine pepsin was then added to a final concentration of 1.3 mg mL−1. The samples were incubated at 37 °C in a shaking water bath (GFL 1092, Burgwedel, Germany) at 100 rpm for 30 min. To simulate intestinal digestion, the pH of the gastric chyme was raised to pH 5.8 with 1 M NaHCO3 dropwise, and 2.5 mL of a pancreatin and bile salt mixture was added to final concentrations of 0.175 and 1.10 mg mL−1, respectively. Afterwards, the pH was adjusted to pH 6.5 with 1 M NaHCO3, and the samples were incubated at 37 °C in a shaking water bath at 100 rpm for 2 h. A blank without flavonol was incubated under the same conditions for correcting the interferences from the digestive enzymes and buffers. After gastrointestinal digestion, the samples were cooled by immersing in an ice bath and then centrifuged at 10
000 rpm for 30 min at 4 °C (Megafuge 8R; Thermo Scientific, Darmstadt, Germany) to separate the soluble or bioaccessible fraction and the residual fraction. Bioaccessible fractions of the digests were kept at −80 °C until further analysis. All experiments were performed in triplicate.
2.4. Antioxidant capacity during in vitro digestion
All spectrophotometric assays were performed using an Infinite M200 UV–visible spectrophotometer (Tecan, Crailsheim, Germany). The antioxidant capacity was estimated by using the cupric ion reducing antioxidant capacity (CUPRAC)16 and 2,2-diphenyl-1-picrylhydrazyl (DPPH)17 assays. In both assays, 6-hydroxy-2,5,7,8-tetramethylchroman-2-carboxylic acid (Trolox) was used as a standard. All samples (quercetin or rutin in a free or microparticle form) were measured in triplicate and the results were expressed as mmol Trolox equivalents (TE) per g of sample.
2.5.
In vitro bioaccessibility calculations
The in vitro bioaccessibility of quercetin or rutin was defined as the amount of bioactive released into the mixed micelles formed by bile salts after the intestinal phase.18 The bioaccessibility was calculated using eqn (1) and expressed as percentage. | Bioaccessibility (%) = (BCdigested/BCinitial) × 100 | (1) |
where BCdigested was the amount of bioactive compounds (quercetin or rutin) recovered in the supernatants of the centrifuged final digests (BF; bioaccessible fraction) and BCinitial was the initial amount of bioactive compounds in the stock solution.
2.6. Cell culture
Caco-2 cells were cultured in Dulbecco's modified Eagle's medium including 4.5 g L−1 glucose and stable glutamine supplemented with 20% fetal bovine serum, 1% non-essential amino acids, 100 U mL−1 penicillin and 100 μg mL−1 streptomycin. Cells were incubated at 37 °C under a humidified atmosphere of 5% CO2 in air. The cells were subcultured at 70–80% confluence using trypsin. For the transport experiment, Caco-2 cells were seeded in inserts of 6-well transwell plates (0.4 μm pore diameter, 24 mm insert, Sarstedt, Nümbrecht, Germany) at a density of 4 × 104 cell per well, with 2 mL of medium in the apical side and 2.5 mL of medium in the basolateral side. The cells were allowed to grow and differentiate to confluent monolayers for 21 days post-seeding. The cell monolayer integrity during differentiation of Caco-2 cells was monitored by measuring the transepithelial electrical resistance, TEER value, using a Millicell© ERS-2 Volt-Ohm Meter (Millipore, Bedford, MA, USA). Only differentiated enterocytes displaying unchanged TEER values were used in the experiment. The medium was changed three times per week. The passage numbers of the cells used in this study were less than 30.
2.7. Cytotoxicity test
Caco-2 cells were seeded in 96 well plates, allowed to differentiate for 14 days after confluency and treated with digests at dilutions of 1/2, 1/5 and 1/10 (v/v) in HBSS. After 4 h of treatment, the cytotoxic effect of the samples on Caco-2 cells was evaluated using the sulforhodamine B (SRB) assay as described by Longo-Sorbello et al.19 For the SRB assay, differentiated cells were first fixed by the addition of 50 μL of 50% TCA ((trichloroacetic acid) in Ultrapure water) and kept at 4 °C for 45 min. The plates were rinsed with tap water and air-dried. Afterward, the cells were stained with 70 μL SRB solution (0.4% in 1% glacial acetic acid) and left for 15 min. The plates were then rinsed with 1% glacial acetic acid in Ultrapure water and air-dried and the stain was re-suspended in 200 μL of 10 mM Tris-buffer. Thereafter, the absorbance was determined at 490 nm, subtracting the background measurement at 620 nm.
2.8. Transport experiment in Caco-2 cells
The transport experiment was carried out according to Wu et al.20 Briefly, the medium in the transwells was changed to HBSS and preincubated for 1 h and then removed. The BF used for the Caco-2 experiments was diluted 1
:
5 (v
:
v) with HBSS, since the cell viability after 4 h of exposure to the samples under such conditions, assessed by SRB assay, was higher than 85–90%. Incubation was performed at 37 °C with 5% CO2 for 4 h. During incubation, 200 μL of samples was collected from both the apical and basolateral compartments every 2 h, after which 200 μL of HBSS was added to adjust the volume of the media. The TEER value of the cells was detected before treatment and immediately after the last sampling to ensure the integrity of the monolayer. Then the HBSS was replaced with growth medium, and the monolayers were incubated for another 20 h to measure the irreversible damage to the cell monolayer. Therefore, after 24 h, monolayer integrity was tested using TEER measurements. The samples were stored at −80 °C until further analysis.
2.9. Identification and quantification of flavonols by UHPLC
The method for the determination of flavonoids by UHPLC was adapted from Ozkan et al.21 with minor modifications. Briefly, samples (unprocessed flavonols and microparticles) were passed through 0.20 μm membrane filters and injected into a UHPLC system (Agilent 1290 Infinity II, Germany). The UHPLC system was equipped with a binary pump with a vacuum degasser, an autosampler, a photodiode array detector and a column oven. A Phenomenex Luna C18(2) analytical column (250 × 4.6 mm, 5 μm) with a C18 guard column (Phenomenex, Aschaffenburg, Germany) was used for the separation. A mobile phase consisting of TFA/MQ water (trifluoroacetic acid in Ultrapure water) (1 mL L−1; eluent A) and TFA/acetonitrile (1 mL L−1; eluent B) was used. The linear gradient was as follows: 0 min, 5% B; 0–6.48 min, 35% B; 6.48–6.77 min, 100% B; 6.77–8.00 min, 100% B; 8.00–8.10 min, 5% B. The injection volume was 10 μL, the flow rate was 0.6 mL min−1 and spectral measurements were performed at 369 and 257 nm for quercetin and rutin, respectively. For calibration curves, the chromatographic peak area of the standards versus nominal concentrations was plotted. All samples were measured in triplicate and the results were expressed as milligrams per g sample.
2.10. Statistical analysis
Experiments were performed at least in triplicate. The results were reported as mean ± standard deviation. Error bars in figures represented standard deviations. Statistical analysis was applied using SPSS software (version 20.0, SPSS Inc. Chicago, IL, USA). Treatments were compared using one-way analysis of variance (ANOVA) followed by a Tukey post hoc test (p < 0.05).
3. Results and discussion
3.1.
In vitro bioaccessibility of flavonols
The use of polyphenols is currently limited due to its relatively low water solubility, chemical instability in the GI tract, and thus, reduced oral bioavailability (2–20% absorption).22–24 Therefore, it is essential to investigate the effects of digestion on their stability to reveal the mechanism of absorption and metabolism. It was assumed that the bioaccessible fraction of a compound incorporated within the micelles would be available for adsorption by epithelial cells in the small intestine.25 For these reasons, effects of the precipitation process, the physical state of quercetin/rutin and different food models on the bioaccessibility were studied. Bioaccessibility was quantified by measuring the amount of quercetin/rutin within the micelle phase collected at the end of the simulated small intestine phase.
Quercetin had a low bioaccessibility value of 0.21 ± 0.09% when simply suspended in a hydrophilic food simulant, which can be related to its low solubility and crystalline state in water (Fig. 1). Moreover, there was no triacylglycerol present that could be digested and form mixed micelles to solubilize the hydrophobic component. According to the literature, quercetin is chemically unstable because of hydroxyl groups and the unstable pyrone structure.26 The degradation of quercetin was found to be sensitive to medium pH, especially at alkaline pH values (pH > 7). Increasing the medium pH value from 6.0 to 6.8 or 7.5 increased the k values by 2- and 12-fold, respectively.27 On the other hand, the bioaccessibility of Q-PVP microparticles was found to be significantly higher (19.8 ± 2.02%) than that of the unprocessed one (0.21 ± 0.09%) (p < 0.05). These results suggest that the use of PVP could help achieve amorphization and increased dissolution rate, thus enhanced bioaccessibility.
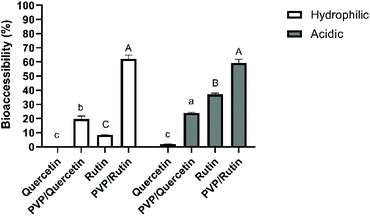 |
| Fig. 1 The bioaccessibility of unprocessed and supercritical antisolvent (SAS) processed quercetin or rutin using polyvinylpyrrolidone (PVP) in two different food stimulants. The data presented in this figure consist of average values ± standard deviation of three independent batches. Different small letters on the bars represent statistically significant differences between quercetin samples (p < 0.05). Different capital letters on the bars indicate significant differences (p < 0.05) between rutin samples. | |
As for the acidic food model, while a lower bioaccessibility value (1.91 ± 0.13%) was obtained with unprocessed quercetin, there was a significant improvement (p < 0.05) in quercetin within Q-PVP microparticles (24.03 ± 0.44%). Strongly acidic conditions may result in the degradation of quercetin to phenolic acids (e.g., protocatechuic acid) and the loss of the skeletal structure of quercetin.8 It can be deduced from the results that the PVP matrix could protect the bioactive compound under acidic conditions which was selected as the worst-case scenario in which the release was expected to be faster due to the quick swelling or even dissolution of the polymeric matrices under acidic conditions.12
For increasing quercetin bioaccessibility, different attempts have been made. Aditya et al.28 achieved 60% bioaccessibility of quercetin within nanostructured lipid carriers and lipid nanoemulsions, and 35% with solid lipid nanoparticles, which were much higher than that of free quercetin solution (∼7%). Similarly, the quercetin bioaccessibility was increased from <5% in bulk water to 53% within the nanoemulsion.25 Ni et al.29 reported a bioaccessibility of 33.6% for quercetin in nanostructured lipid carriers, which was higher than that obtained in water (<2%). Additionally, a Eudragit, an anionic copolymer based on methacrylic acid and ethyl acrylate, based delivery system was also used to increase the stability and solubility of quercetin in the GI tract. After the simulated GI digestion, quercetin release was enhanced from 7% (for free quercetin dispersed in water) to 22% for quercetin-loaded polymeric nanoparticles.30 Besides, Chen et al.18 reported the bioaccessibility of quercetin as 12.7% by incorporating it into rice bran protein-stabilized nanoemulsions, whereas the bioaccessibility of free quercetin was found to be 1.40%.
The bioaccessibility of rutin in a hydrophilic food model was obtained as 8.38 ± 0.10%. It has been reported that phenolics are highly sensitive to alkaline conditions and may degrade during gastrointestinal digestion.31 On the other hand, the recovery of rutin was statistically enhanced (p < 0.05) to 62.1 ± 2.66% when it was coprecipitated with PVP. These results are in agreement with those of Bermúdez-Soto et al.31 who showed that rutin exhibited a high bioaccessibility (3% loss) after pancreatic digestion. In line with the results of rutin bioaccessibility in an acidic food model, the recovery of rutin was increased from 37.1 ± 0.84 to 59.4 ± 2.45% by SAS processing. The results indicated a protective effect of the PVP matrix on rutin under both hydrophilic and acidic conditions during in vitro digestion due to its pH-stable properties.32 It can also be deduced from the results that the stability of rutin in both unprocessed and microparticle forms was found to be higher than that of quercetin ones. Indeed, previous studies have noticed a stronger stability of rutin rather than quercetin under hydrophilic conditions, indicating a stabilization effect of glycoside linkages present in rutin.33
3.2. Changes in the in vitro antioxidant capacity during digestion
Changes in the antioxidant capacity of the samples in different food models, measured by both CUPRAC and DPPH assays, during the in vitro GI digestion are shown in Table 1.
Table 1 Changes in the antioxidant activities of the samples during gastrointestinal (GI) digestiona
Sample |
Before digestion |
Hydrophilic |
Acidic |
After digestion |
Increase (fold) |
After digestion |
Increase (fold) |
The data presented in this table consist of average values ± standard deviation of three independent batches. Different small letters in the same rows represent statistically significant differences (p < 0.05) between quercetin and rutin samples, individually. Total antioxidant capacity is expressed as mmol Trolox equivalents per g sample.
|
Cupric ion reducing antioxidant capacity (CUPRAC)
|
Quercetin |
Q |
17.2 ± 1.6a |
0.10 ± 0.02c |
— |
4.63 ± 0.63b |
0.27 |
Q-PVP |
321 ± 9.0a |
205 ± 17.0b |
0.64 |
206 ± 24.0b |
0.64 |
Rutin |
R |
11.3 ± 1.3a |
0.66 ± 0.12c |
— |
4.32 ± 0.28b |
0.38 |
R-PVP |
147 ± 3.0a |
61.8 ± 3.6b |
0.42 |
59.3 ± 6.6b |
0.40 |
2,2-Diphenyl-1-picrylhydrazyl (DPPH)
|
Quercetin |
Q |
6.28 ± 0.07a |
0.10 ± 0.0c |
— |
0.76 ± 0.32b |
0.12 |
Q-PVP |
6.40 ± 0.27c |
58.6 ± 5.2a |
9.16 |
37.1 ± 4.8b |
5.80 |
Rutin |
R |
2.41 ± 0.16a |
0.60 ± 0.05c |
0.25 |
1.10 ± 0.31b |
0.46 |
R-PVP |
2.59 ± 0.26c |
18.1 ± 0.6a |
6.99 |
12.3 ± 2.0b |
4.75 |
The results revealed that flavonol-free microparticles (PVP matrix) showed almost no antioxidant capacity (data not shown) under all conditions. In line with the antioxidant capacity results obtained for unprocessed flavonols after in vitro digestion, the values of CUPRAC antioxidant capacity were found to be quite lower than those of PVP microparticles in all food models due to the crystalline structures of these compounds under these conditions. Therefore, the findings indicated preservative and supportive effects of the SAS process on the antioxidant capacities of bioactive compounds. In the CUPRAC assay, Cu(II) is reduced to Cu(I) through the action of electron-donating (ET) antioxidants.16 It could be stated that the use of PVP as a carrier favored the electron-donating ability of the flavonol loaded PVP-based microparticles (higher reducing power) when compared with unprocessed ones. Samborska et al.34 indicated that the CUPRAC antioxidant activities of spray-dried honey powders increased with the use of maltodextrin as a carrier. The CUPRAC method is superior to other ET-based assays in terms of working pH.35 Thus, it could be deduced from the results that the decrease in CUPRAC values in hydrophilic and acidic food simulants may arise from the reduced stability of flavonols under these conditions.
Similarly, the DPPH radical scavenging capacity of flavonols within the PVP matrix was found to be much higher than those of unprocessed ones. In addition to this, the antioxidant activities of unprocessed quercetin and rutin decreased after digestion (p < 0.05), whereas it increased for SAS processed Q-PVP and R-PVP microparticles in all food models (p < 0.05). The DPPH assay is a mixed mode assay which is attributed to the scavenging of a radical by antioxidants with both hydrogen atom transfer and electron transfer based mechanisms.36 It is well known that the radical scavenging capacity of polyphenols is strongly pH-dependent. Higher pH values lead to an enhancement in this capacity. The increase in the radical scavenging capacity is based on the deprotonation of the hydroxyl moieties present on the aromatic rings of the phenolic compounds.37,38 The transition from the stomach to the intestinal environment may induce structural changes in the phenolic molecules by ionization of the hydroxyl groups.39 Similar results were also obtained by Tagliazucchi et al.39 It was found that the radical scavenging activities of gallic acid, caffeic acid, catechin, quercetin and resveratrol increased after pancreatic digestion.
Regarding the effect of food models on the antioxidant capacity of the samples after in vitro digestion, there was a significant change (p < 0.05) in the DPPH antiradical scavenging abilities of the samples. The results show that a statistically higher antioxidant capacity of flavonols in the PVP matrix is achieved in hydrophilic media. Similar to the results obtained in the present work, Pekal & Pyrzynska40 found that there was a significant variation in the DPPH inhibition of tea infusions in the measuring system in buffered (methanol with acetate buffer at pH 5.5) and non-buffered reagents (methanol). Indeed, higher antioxidant capacity of tea infusions was determined in less acidic media.
3.3. Determination of the cytotoxicity in Caco-2 cells
Before transport experiments, it is necessary to determine the maximum non-toxic sample concentration by a cytotoxicity test. Thus, SRB assay was performed to determine the cellular viability based on the cellular protein content. Cells were allowed to differentiate for 14 days after confluency. Then, Caco-2 cells were treated with flavonol-free and flavonol-containing digests at different dilution ratios. 1/5 dilution ratios of the digests with Q, Q/PVP, R or R/PVP did not result in a major cytotoxic effect (cell viability was never <85%), indicating no cell release from the monolayer. Therefore, a 1/5 dilution was used in further Caco-2 transport experiments.
3.4. Transport experiments
The TEER values before and after 4 and 24 h of treatment for all tested digests were monitored. Before treatment, the TEER values of all conditions were found to be higher than 300 Ω cm2. The TEER values did not change by more than 10% of the original values (not shown) after 4 and 24 h, and are considerably above the recommended minimum level (200 Ω cm2) needed for monolayer integrity.41 Therefore, we conclude that the monolayer integrity was not irreversibly damaged during transport experiments in the presence of a 1/5 diluted digestive matrix and paracellular transport was not affected, which could influence bioavailability calculations.
One of the aims of this study was to determine the effects of the SAS process and different food models on the transport dynamics of quercetin and rutin, in the form of free or flavonol-loaded PVP microparticles, in the human digestive system using a recognized cell-based assay. Although there have been a series of studies related to the absorption of bioactive compounds,9 the effects of food models with different pH values and the SAS process on the uptake of phenolic compounds are largely unknown.
In the present research, a combined in vitro digestion/Caco-2 cell culture system was used to screen the transport of flavonols – quercetin and rutin – across the gut epithelium. The digests of quercetin and rutin in the form of free or flavonol-loaded PVP microparticles from different food simulants were added to the apical (top) side of the Caco-2 cells growing in transwells. Then, the samples were collected from the apical compartment after 0, 2 and 4 h and from the basolateral (bottom) compartment after 2 and 4 h of incubation. At the end of 2 and 4 h exposure, recoveries on the apical and basolateral sides were quantified for flavonols, as a percentage of the amount of flavonols loaded to the apical side at 0 h (Table 2).
Table 2 Apical and basolateral side recovery and transport efficiency of flavonols in free and microparticle forms
Food modela |
t = 2 h |
t = 4 h |
Apical side recoveryb (%) |
Basolateral side recoveryc (%) |
Transport efficiencyd |
Apical side recoveryb (%) |
Basolateral side recoveryc (%) |
Transport efficiencyd |
The data presented in this table consist of average values ± standard deviation of three independent batches. Different small letters represent statistically significant differences (p < 0.05) between quercetin or rutin samples, individually. n.d.: not detected.
Apical side recovery percentages were calculated as (flavonol concentration at the apical side after transport)/(flavonol concentration at the apical side at 0 h of incubation) × 100.
Basolateral side recovery percentages were calculated as (flavonol concentration at the basolateral side after transport)/(flavonol concentration at the apical side at 0 h of incubation) × 100.
Transport efficiency was calculated as (basolateral side recovery, %)/(apical side recovery, %).
|
Quercetin
|
Hydrophilic |
n.d. |
n.d. |
—
|
n.d. |
n.d. |
—
|
Acidic |
n.d. |
n.d. |
—
|
n.d. |
n.d. |
—
|
Quercetin loaded PVP based microparticles
|
Hydrophilic |
66.0 ± 7.69b |
0.98 ± 0.03c |
0.015 ± 0.004c |
61.0 ± 9.96b |
1.93 ± 0.71b |
0.032 ± 0.008b |
Acidic |
81.0 ± 5.71a |
1.38 ± 0.36bc |
0.017 ± 0.005c |
65.0 ± 2.63b |
3.89 ± 0.38a |
0.060 ± 0.006a |
Rutin
|
Hydrophilic |
73.2 ± 3.21c |
1.17 ± 0.00c |
0.010 ± 0.000c |
69.0 ± 2.944c |
3.52 ± 0.77b |
0.011 ± 0.001c |
Acidic |
88.4 ± 4.10ab |
0.72 ± 0.01c |
0.013 ± 0.000c |
85.0 ± 5.00b |
0.74 ± 0.77c |
0.016 ± 0.000c |
Rutin loaded PVP based microparticles
|
Hydrophilic |
87.8 ± 2.91ab |
1.42 ± 0.03c |
0.014 ± 0.000c |
82.5 ± 3.49b |
4.65 ± 0.67a |
0.048 ± 0.006b |
Acidic |
89.0 ± 4.56a |
1.21 ± 0.17c |
0.017 ± 0.002c |
87.0 ± 2.26ab |
1.35 ± 0.67c |
0.058 ± 0.005a |
According to the results, free quercetin from hydrophilic or acidic food simulants could not be detected at the apical or basolateral sides. After in vitro gastrointestinal digestion, quite lower amount of unprocessed quercetin was detected in both hydrophilic and acidic food models as described above. Before the cell culture study, the digests were diluted with HBSS at a pH of 7.8; thereby intact quercetin in the digests may be degraded at this pH. The recovery of quercetin within the PVP matrix in the apical compartment after 2 h of incubation were found to be 66 and 81% for hydrophilic and acidic food simulants, respectively. Furthermore, after 4 h of treatment with the samples, apical recovery of quercetin was decreased to 61 and 65% for hydrophilic and acidic food simulants, respectively. While the basolateral side recoveries of intact quercetin were found to be 0.98 and 1.38% after 2 h of treatment, after 4 h, the amount of compounds transported to the basolateral compartment increased to 1.93 and 3.89%, for hydrophilic and acidic food models, respectively. The basolateral side recovery (indicating transport) was corrected for the apical side recovery (indicating stability), expressed as transport efficiency.42 The transport efficiency of quercetin in the acidic food model was greater than that of the hydrophilic food model, indicating higher retention. What has become evident from this research is that the basolateral recovery and transportation of quercetin are altered positively with SAS processing. While quercetin in its free form could not be detected in the basolateral compartment after 2 or 4 h exposure, relatively higher transportation could be achieved with quercetin in the form of PVP-based microparticles.
As for the transportation of rutin in both free and microparticle forms, while apical recoveries (t = 4 h) in hydrophilic food simulants were statistically higher than those of acidic food simulants, basolateral recoveries (t = 4 h) in acidic food simulants were statistically higher than those of hydrophilic ones. The major outcome of this work was that the transport of rutin was enhanced by SAS processing. In detail, after 4 h exposure, the transport efficiency (t = 4 h) was found to be 1.2 and 1.5 times higher for the rutin within the PVP matrix from hydrophilic and acidic food models, respectively, compared to the unprocessed ones.
The greater retention of quercetin and rutin in microparticle form is attributed to the SAS processing by using PVP as an excipient which causes an enhanced dissolution rate and, thus, increased bioavailability. It has been previously demonstrated that micrometric or sub-micrometric composite particles with an amorphous structure fabricated by SAS processing under proper operating conditions led to significantly increased dissolution rates of various active compounds.11 Indeed, since the particle size reduction caused an increase in the specific surface area and a higher contact between the compounds and the dissolution medium, the dissolution rate can be improved by the micronization of the active compounds.43 In addition to these, the improved recoveries of these flavonols are related to their strengthened stabilities by the use of PVP.32
The transepithelial transport of quercetin and rutin has been studied before using Caco-2 cells. In the small intestine, most flavonoid glycosides – like rutin – degraded to their corresponding aglycones by deglycosylation using luminal lactase phloridzin hydrolase (LPH) or β-glucosidase.44 Thereafter, flavonoid aglycones, such as quercetin, enter enterocytes and a transepithelial flux originates from passive diffusion followed by various conjugation reactions including sulphation and glucuronidation.45 Moreover, methylation was also found to be the main metabolism of quercetin in buckwheat samples after intestinal transport through Caco–2/HepG2 coculture models.46 Similar to the results obtained here, it was reported that 0.3–6.4% of quercetin and its conjugates was detected after the consumption of quercetin-rich foods such as apples, onions, buckwheat, tomato products and cranberrybush purée samples.47–49 The results of this study were in line with those of in vivo studies conducted with volunteers, in which the fate of flavonols in lightly fried onions was investigated.50 According to the results, about 4% recovery of the ingested flavonol glucoside metabolites was achieved from plasma and urine samples. Besides, rutin metabolites in urine collected from healthy human subjects were studied after the consumption of tomato juice fortified with rutin (quercetin-3-O-rutinoside). It was reported that the excretion of the metabolites ranged from 0.02% to 2.8% of intake.51
4. Conclusion
The aim of this study was to investigate the effect of SAS processing on the absorption of flavonoids, namely quercetin and rutin, in the human digestive system using a recognized combined gastrointestinal digestion/cell-based assay. Moreover, aqueous hydrophilic and acidic conditions were simulated to analyze food-related factors that could have an impact on the transport of these compounds across the gut epithelium. SAS processing enhanced the recovery of quercetin (94 and 13 times under hydrophilic and acidic conditions, respectively) and rutin (7 and 2 times under hydrophilic and acidic conditions, respectively) after in vitro digestion. Besides, transepithelial transportation of these flavonoids was also affected positively. Thereby, it can be deduced from the results that SAS processing can be a useful method in pharmaceutical and nutraceutical applications due to its positive effects on the stability, bioaccessibility, bioavailability and thus nutritional value of bioactive compounds. On the other hand, current studies could be enriched with colonic fermentation to examine the fate of bioactive compounds in an entire approach; since the majority of the phenolic substances pass through the colon and they are metabolized by the colonic microbiota. Furthermore, owing to the significant effects on the transportation of the bioactive compounds across the gut epithelium, food-related factors like the matrix effect could be also included in future studies.
Author contributions
Iolanda De Marco and Esra Capanoglu contributed to the supervision, conceptualization and methodology. Tuba Esatbeyoglu contributed to the supervision, conceptualization, methodology and funding acquisition. Gulay Ozkan contributed to the conceptualization, methodology, formal analysis, investigation, resources, writing – original draft and visualization. Paola Franco contributed to the investigation and methodology. All the authors critically reviewed and contributed to the editing.
Conflicts of interest
There are no conflicts to declare.
Acknowledgements
This work was supported by the Istanbul Technical University, Scientific Research Projects (BAP) Unit [project number MDK-2018-41359].
References
- L. H. Yao, Y. M. Jiang, J. Shi, F. A. Tomás-Barberán, N. Datta, R. Singanusong and S. S. Chen, Flavonoids in food and their health benefits, Plant Foods Hum. Nutr., 2004, 59, 113–122 CrossRef CAS PubMed.
- Ž. B. Milanović, M. R. Antonijević, A. D. Amić, E. H. Avdović, D. S. Dimić, D. A. Milenković and Z. S. Marković, Inhibitory activity of quercetin, its metabolite, and standard antiviral drugs towards enzymes essential for SARS-CoV-2: The role of acid-base equilibria, RSC Adv., 2021, 11, 2838–2847 RSC.
- M. M. Rashad, A. E. Mahmoud, M. M. Ali, M. U. Nooman and A. S. Al-Kashef, Antioxidant and anticancer agents produced from pineapple waste by solid state fermentation, Int. J. Toxicol. Pharmacol. Res., 2015, 7, 287–296 Search PubMed.
- M. Russo, C. Spagnuolo, I. Tedesco, S. Bilotto and G. L. Russo, The flavonoid quercetin in disease prevention and therapy: Facts and fancies, Biochem. Pharmacol., 2012, 83, 6–15 CrossRef CAS PubMed.
- J. Huang, Q. Wang, T. Li, N. Xia and Q. Xia, Nanostructured lipid carrier (NLC) as a strategy for encapsulation of quercetin and linseed oil: Preparation and in vitro characterization studies, J. Food Eng., 2017, 215, 1–12 CrossRef CAS.
- H. G. Ulusoy and N. Sanlier, A minireview of quercetin: from its metabolism to possible mechanisms of its biological activities, Crit. Rev. Food Sci. Nutr., 2020, 60, 3290–3303 CrossRef CAS PubMed.
- I. R. Record and J. M. Lane, Simulated intestinal digestion of green and black teas, Food Chem., 2001, 73, 481–486 CrossRef CAS.
- W. Wang, C. Sun, L. Mao, P. Ma, F. Liu, J. Yang and Y. Gao, The biological activities, chemical stability, metabolism and delivery systems of quercetin: A review, Trends Food Sci. Technol., 2016, 56, 21–38 CrossRef CAS.
- G. Ozkan, T. Kostka, T. Esatbeyoglu and E. Capanoglu, Effects of lipid-based encapsulation on the bioaccessibility and bioavailability of phenolic compounds, Molecules, 2020, 25, 5545 CrossRef CAS PubMed.
- H. H. Peng, D. X. Hong, Y. X. Guan and S. J. Yao, Preparation of pH-responsive DOX-loaded chitosan nanoparticles using supercritical assisted atomization with an enhanced mixer, Int. J. Pharm., 2018, 558, 82–90 CrossRef PubMed.
- G. Ozkan, P. Franco, E. Capanoglu and I. De Marco, PVP/flavonoid coprecipitation by supercritical antisolvent process, Chem. Eng. Process. – Process Intensif., 2019, 146, 107689 CrossRef CAS.
- E. Atay, M. J. Fabra, M. Martínez-Sanz, L. G. Gomez-Mascaraque, A. Altan and A. Lopez-Rubio, Development and characterization of chitosan/gelatin electrosprayed microparticles as food grade delivery vehicles for anthocyanin extracts, Food Hydrocolloids, 2018, 77, 699–710 CrossRef CAS.
- M. Sessa, R. Tsao, R. Liu, G. Ferrari and F. Donsì, Evaluation of the stability and antioxidant activity of nanoencapsulated resveratrol during in vitro digestion, J. Agric. Food Chem., 2011, 59, 12352–12360 CrossRef CAS PubMed.
- P. Knekt, J. Kumpulainen, R. Järvinen, H. Rissanen, M. Heliövaara, A. Reunanen, T. Hakulinen and A. Aromaa, Flavonoid intake and risk of chronic diseases, Am. J. Clin. Nutr., 2002, 76, 560–568 CrossRef CAS PubMed.
- H. Böhm, H. Boeing, J. Hempel, B. Raab and A. Kroke, Flavonols, flavone and anthocyanins as natural antioxidants of food and their possible role in the prevention of chronic diseases, Z. Ernahrungswiss., 1998, 37, 147–163 Search PubMed.
- R. Apak, K. Güçlü, M. Özyürek and S. E. Karademir, Novel total antioxidant capacity index for dietary polyphenols and vitamins C and E, using their cupric ion reducing capability in the presence of neocuproine: CUPRAC method, J. Agric. Food Chem., 2004, 52, 7970–7981 CrossRef CAS PubMed.
- P. Molyneux, The use of the stable free radical diphenylpicryl-hydrazyl (DPPH) for estimating antioxidant activity, Songklanakarin J. Sci. Technol., 2004, 26, 211–219 CAS.
- W. Chen, X. Ju, R. E. Aluko, Y. Zou, Z. Wang, M. Liu and R. He, Rice bran protein-based nanoemulsion carrier for improving stability and bioavailability of quercetin, Food Hydrocolloids, 2020, 108, 106042 CrossRef CAS.
- G. S. A. Longo-Sorbello, G. Saydam, D. Banerjee and J. R. Bertino, Cytotoxicity and cell growth assays, Cell Biol., 2006, 1, 315–324 Search PubMed.
- T. Wu, C. Grootaert, S. Voorspoels, G. Jacobs, J. Pitart, S. Kamiloglu, S. Possemiers, M. Heinonen, N. Kardum, M. Glibetic, G. Smagghe, K. Raes and J. Van Camp, Aronia (Aronia melanocarpa) phenolics bioavailability in a combined in vitro digestion/Caco-2 cell model is structure and colon region dependent, J. Funct. Foods, 2017, 38, 128–139 CrossRef CAS.
- G. Ozkan, A. S. Stübler, K. Aganovic, G. Dräger, T. Esatbeyoglu and E. Capanoglu, Retention of polyphenols and vitamin C in cranberrybush purée (Viburnum opulus) by means of non-thermal treatments, Food Chem., 2021, 360, 129918 CrossRef CAS PubMed.
- L. Q. Zou, S. F. Peng, W. Liu, L. Gan, W. L. Liu, R. H. Liang, C. M. Liu, Y. L. Cao, Z. Liu and X. Chen, Improved in vitro digestion stability of (-)-epigallocatechin gallate through nanoliposome encapsulation, Food Res. Int., 2014, 64, 492–499 CrossRef CAS PubMed.
- X. Chen, L. Zou, W. Liu and D. J. McClements, Potential of excipient emulsions for improving quercetin bioaccessibility and antioxidant activity: an in vitro study, J. Agric. Food Chem., 2016, 64, 3653–3660 CrossRef CAS PubMed.
- M. Hu, Commentary: Bioavailability of flavonoids and polyphenols: Call to arms, Mol. Pharm., 2007, 4, 803–806 CrossRef CAS PubMed.
- H. Pool, S. Mendoza, H. Xiao and D. J. McClements, Encapsulation and release of hydrophobic bioactive components in nanoemulsion-based delivery systems: Impact of physical form on quercetin bioaccessibility, Food Funct., 2013, 4, 162–174 RSC.
- X. Lv, T. Liu, H. Ma, Y. Tian, L. Li, Z. Li, M. Gao, J. Zhang and Z. Tang, Preparation of essential oil-based microemulsions for improving the solubility, ph stability, photostability, and skin permeation of quercetin, AAPS PharmSciTech, 2017, 18, 3097–3104 CrossRef CAS PubMed.
- J. Wang and Xi. H. Zhao, Degradation kinetics of fisetin and quercetin in solutions affected by medium pH, temperature and co-existing proteins, J. Serb. Chem. Soc., 2016, 81, 243–253 CrossRef CAS.
- N. P. Aditya, A. S. Macedo, S. Doktorovova, E. B. Souto, S. Kim, P. S. Chang and S. Ko, Development and evaluation of lipid nanocarriers for quercetin delivery: A comparative study of solid lipid nanoparticles (SLN), nanostructured lipid carriers (NLC), and lipid nanoemulsions (LNE), LWT – Food Sci. Technol., 2014, 59, 115–121 CrossRef CAS.
- S. Ni, R. Sun, G. Zhao and Q. Xia, Quercetin loaded nanostructured lipid carrier for food fortification: Preparation, characterization and in vitro study, J. Food Process Eng., 2015, 38, 93–106 CrossRef CAS.
- H. Pool, D. Quintanar, J. de D. Figueroa, J. E. H. Bechara, D. J. McClements and S. Mendoza, Polymeric nanoparticles as oral delivery systems for encapsulation and release of polyphenolic compounds: Impact on quercetin antioxidant activity & bioaccessibility, Food Biophys., 2012, 7, 276–288 CrossRef.
- M. J. Bermúdez-Soto, F. A. Tomás-Barberán and M. T. García-Conesa, Stability of polyphenols in chokeberry (Aronia melanocarpa) subjected to in vitro gastric and pancreatic digestion, Food Chem., 2007, 102, 865–874 CrossRef.
- P. Franco and I. De Marco, The use of poly(N-vinyl pyrrolidone) in the delivery of drugs: A review, Polymers, 2020, 12, 18–21 Search PubMed.
- E. B. Dechene, The relative stability of rutin and quercetin in alkaline solution, J. Am. Pharm. Assoc., Sci. Ed., 1951, 40, 495–497 CrossRef CAS PubMed.
- K. Samborska, A. Jedlińska, A. Wiktor, D. Derewiaka, R. Wołosiak, A. Matwijczuk, W. Jamróz, K. Skwarczyńska-Maj, D. Kiełczewski, L. Błażowski, M. Tułodziecki and D. Witrowa-Rajchert, The effect of low-temperature spray drying with dehumidified air on phenolic compounds, antioxidant activity, and aroma compounds of rapeseed honey powders, Food Bioprocess Technol., 2019, 12, 919–932 CrossRef CAS.
- R. Apak, K. Güçlü, B. Demirata, M. Özyürek, S. E. Çelik, B. Bektaşoǧlu, K. I. Berker and D. Özyurt, Comparative evaluation of various total antioxidant capacity assays applied to phenolic compounds with the CUPRAC assay, Molecules, 2007, 12, 1496–1547 CrossRef CAS PubMed.
-
E. Capanoglu, S. Kamiloglu, G. Ozkan and R. Apak, Evaluation of antioxidant activity/capacity measurement methods for food products, in Measurement of Antioxidant Activity and Capacity: Recent Trends and Applications, ed. R. Apak, E. Capanoglu and F. Shahidi, Wiley, UK, 2018, pp. 273–286 Search PubMed.
- K. Mukai, W. Oka, K. Watanabe, Y. Egawa, S. I. Nagaoka and J. Terao, Kinetic study of free-radical-scavenging action of flavonoids in homogeneous and aqueous triton X-100 micellar solutions, J. Phys. Chem. A, 1997, 101, 3746–3753 CrossRef CAS.
- B. Tyrakowska, A. E. M. F. Soffers, H. Szymusiak, S. Boeren, M. G. Boersma, K. Lemańska, J. Vervoort and I. M. C. M. Rietjens, TEAC antioxidant activity of 4-hydroxybenzoates, Free Radicals Biol. Med., 1999, 27, 11–12 CrossRef.
- D. Tagliazucchi, E. Verzelloni, D. Bertolini and A. Conte,
In vitro bioaccessibility and antioxidant activity of grape polyphenols, Food Chem., 2010, 120, 599–606 CrossRef CAS.
- A. Pekal and K. Pyrzynska, Effect of pH and metal ions on DPPH radical scavenging activity of tea, Int. J. Food Sci. Nutr., 2015, 66, 58–62 CrossRef CAS PubMed.
- K. Palm, K. Luthman, A. L. Ungell, G. Strandlund and P. Artursson, Correlation of drug absorption with molecular surface properties, J. Pharm. Sci., 1996, 85, 32–39 CrossRef CAS PubMed.
- G. Toydemir, D. Boyacioglu, E. Capanoglu, I. M. Van Der Meer, M. M. M. Tomassen, R. D. Hall, J. J. Mes and J. Beekwilder, Investigating the transport dynamics of anthocyanins from unprocessed fruit and processed fruit juice from sour cherry (Prunus cerasus L.) across intestinal epithelial cells, J. Agric. Food Chem., 2013, 61, 11434–11441 CrossRef CAS PubMed.
- K. T. Savjani, A. K. Gajjar and J. K. Savjani, Drug solubility: Importance and enhancement techniques, ISRN Pharmacol., 2012, 2012, 1–10 CrossRef PubMed.
- K. Németh, G. W. Plumb, J. G. Berrin, N. Juge, R. Jacob, H. Y. Naim, G. Williamson, D. M. Swallow and P. A. Kroon, Deglycosylation by small intestinal epithelial cell β-glucosidases is a critical step in the absorption and metabolism of dietary flavonoid glycosides in humans, Eur. J. Nutr., 2003, 42, 29–42 CrossRef PubMed.
- J. Erdman, J. Wills and D. Finley, Chocolate: Modern science investigates an ancient medicine. Foreword, J. Nutr., 2000, 130, 2073–2085 CrossRef PubMed.
- Y. Yao, F. Xu, X. Ju, Z. Li and L. Wang, Lipid-lowering effects and intestinal transport of polyphenol extract from digested buckwheat in Caco-2/HepG2 coculture models, J. Agric. Food Chem., 2020, 68, 4205–4214 CrossRef CAS PubMed.
- C. Manach, G. Williamson, C. Morand, A. Scalbert and C. Rémésy, Bioavailability and bioefficacy of polyphenols in humans. I. Review of 97 bioavailability studies, Am. J. Clin. Nutr., 2005, 81, 230–242 CrossRef PubMed.
- K. Kahle, M. Kempf, P. Schreier, W. Scheppach, D. Schrenk, T. Kautenburger, D. Hecker, W. Huemmer, M. Ackermann and E. Richling, Intestinal transit and systemic metabolism of apple polyphenols, Eur. J. Nutr., 2011, 50, 507–522 CrossRef CAS PubMed.
- G. Ozkan, T. Kostka, G. Dräger, E. Capanoglu and T. Esatbeyoglu, Bioaccessibility and transepithelial transportation of cranberrybush (Viburnum opulus) phenolics: Effects of non-thermal processing and food matrix, Food Chem., 2022, 380, 132036 CrossRef CAS PubMed.
- W. Mullen, C. A. Edwards and A. Crozier, Absorption, excretion and metabolite profiling of methyl-, glucuronyl-, glucosyl- and sulpho-conjugates of quercetin in human plasma and urine after ingestion of onions, Br. J. Nutr., 2006, 96, 107–116 CrossRef CAS PubMed.
- I. B. Jaganath, W. Mullen, C. A. Edwards and A. Crozier, The relative contribution of the small and large intestine to the absorption and metabolism of rutin in man, Free Radic. Res., 2006, 40, 1035–1046 CrossRef CAS PubMed.
|
This journal is © The Royal Society of Chemistry 2022 |