DOI:
10.1039/D1FO03145G
(Paper)
Food Funct., 2022,
13, 425-436
Chestnut polysaccharides restore impaired spermatogenesis by adjusting gut microbiota and the intestinal structure†
Received
19th September 2021
, Accepted 28th November 2021
First published on 29th November 2021
Abstract
Our previous study confirmed the beneficial effects of chestnut polysaccharides (CPs) on the spermatogenesis process, but the exact mechanism is not clear. Several studies have demonstrated the importance of balanced gut microbiota in maintaining normal reproductive function. In this study, we investigated the biological functions of CPs from the perspective of gut microbiota function, expecting to find out the specific mechanism of CPs in restoring impaired spermatogenesis. Compared with the control group, the mice treated with busulfan showed a reduced number of germ cells, structural changes in the small intestine and composition alteration in the gut microbiota at several levels, including the phylum and genus. In contrast, the number of germ cells in seminiferous tubules was significantly increased, and the structure of the small intestine and the composition of the gut microbiota were altered in the busulfan-treated mice after the CPs treatment. The 16s rRNA analysis results showed that the Firmicutes was the predominant phylum in all groups followed by Proteobacteria, Bacteroidetes, Actinobacteria, Tenericutes, Cyanobacteria and unidentified bacteria. Interestingly, the subsequent functional analysis implied that the steroid hormone biosynthesis process is the major metabolic pathway in the CPs-mediated restoration process and the experimental results confirmed this speculation. In conclusion, this study confirmed that CPs can restore the impaired spermatogenesis process by adjusting the gut microbiota and intestinal structure, which will also provide technical support and a theoretical basis for the subsequent treatment of male infertility.
Introduction
Polysaccharides are complex compounds including different carbohydrates with various bioactivities and have attracted much attention in recent years.1,2 Numerous studies have revealed that polysaccharides have the ability to regulate the immunity of the intestinal mucosal barrier, alter the composition of gut microbiota, inhibit cancer cell proliferation and improve spermatogenesis.3–10 Polysaccharides can be used as health care products because of these biological functions in regulating body health. Chestnut polysaccharides (CPs) are bioactive substances with multiple nutrients extracted from chestnut fruits and possess several health benefits.11 CPs are compounds containing many monosaccharides in different proportions including glucose, galactose, arabinose, mannose, xylose, rhamnose, and fructose.11,12
The link between gut microorganisms and the reproductive system has attracted the attention of scientists in recent years. Studies have demonstrated that changes in the gut microbiota are related to body health maintenance because the microbiota forms a network regulating the immune system homeostasis, the metabolome, and the reproductive system.13–22 So microbiota derived from the gut can help us to understand the health status of an individual. It was revealed that gut microbiota can act as protectors or invaders in the female reproductive system.23,24 There are many studies about the role of gut microbe disturbance in inducing the polycystic ovary syndrome (PCOS) pathogenesis. Researchers have found that the gut microbiota of healthy women differs from that of the patients with PCOS,25–27 including changes in the bacterial composition or global diversity (α-diversity or β-diversity).25,28–32 The aberrant behavior of gut microbes also disrupts normal physiological processes, causing reproductive problems in the host such as gestational diabetes mellitus (GDM) and early preeclampsia (PE).24,33,34 Researchers also found that gut microbiota is not only involved in the induction of reproductive problems, but also able to protect against reproductive problems in PCOS mice by transplanting microbes from healthy mice.23 Studies on the effect of gut microbiota on the male reproductive system were also conducted by researchers. Ding et al. revealed the mechanism of gut microbiota facilitating male reproduction, and that gut microbiota alters the expression of important genes in the testes.35 Recent studies also suggested that gut microbiota changes can restore male infertility following the administration of alginate oligosaccharides (AOS). The authors explored the sperm quality and spermatogenesis in AOS-dosed animals after fecal microbiota transplantation into busulfan-treated mice and found that microbiota transplantation from healthy mice can improve spermatogenesis by altering bacterial species.36,37
Studies have shown that CPs play a profound role in inhibiting tyrosinase and oxidative activity and benefiting the spermatogenesis of mice exposed to busulfan by improving the expression of important genes,11,12,38 but whether the restoration is caused by the gut microbiota alteration is unknown. 16S rRNA sequencing is a method to detect the microbiota landscape, which can indicate changes in the microbiota distribution and predict the functional pathways of microbiota.39 In this study, we used 16S rRNA sequencing technology to investigate the biological functions of CPs from the perspective of gut microbiota function, expecting to find out the exact mechanism of CPs in restoring impaired spermatogenesis.
Materials and methods
Experimental design of the study
The preliminary work of this study revealed that chestnut polysaccharides could restore the impaired spermatogenesis process. Considering the oral administration of chestnut polysaccharides and the important role of the gut–reproductive axis in the spermatogenesis process, this study mainly used 16S rRNA sequencing to detect the differences in gut microbiota between different model mice. Combined with immunohistochemical detection and other biological means, we expected to find out the correlation between the main components of chestnut polysaccharides, the gut microbiota composition and the intestinal structure and further clarify the important role of the gut microbiota composition in the spermatogenesis process (Fig. S1†).
Breeding environment of mice
Male CD1 mice were purchased from Vital River Laboratory Animal Technology Co., Ltd (Beijing, China). The mice were kept in a house with a 12-hour cycle of light and a constant temperature (22–23 °C) and had free access to food and water during the experimental phase. The Animal Care and Ethics Committee of Qingdao Agricultural University approved the study,40 which was conducted in accordance with the National Institutes of Health guidelines for the care and use of laboratory animals (NIH Publications no. 8023).
Information of chestnut polysaccharides
The CPs used in this study were brought from Wo Te Lai Si biotechnology co., Ltd (Lan Zhou, China). For the specific details on CPs, please refer to ref.41.
Treatment of mice
Busulfan was used to establish an animal model of male sterility.42–48 Treatment groups (10 mice per group) are as follows: (1) vehicle control (Ctrl); (2) chestnut polysaccharides (CPs); (3) busulfan (Bus); (4) busulfan + chestnut polysaccharides (Bus + CPs). 20 mice were treated with busulfan at the concentration of 40 mg per kg body weight and divided into two groups randomly. Fresh CPs solutions were prepared daily and the gavage volume was 0.10 ml per mouse per day. 0.10 mg CPs per kg body weight were given by gavage exposure on mice38and oral gavage was performed on three-week aged mice every day for five weeks as described previously.38
Collection of samples
After five-week treatment, mice were slaughtered in accordance with animal welfare requirements, and the tissues and intestinal contents were collected for further analysis. Small intestine and testis tissues were placed in paraformaldehyde solution for fixation. Intestinal contents were quickly collected from the gut in a sterile environment and stored at −80 °C.
16S rRNA sequencing and analysis
After collecting the intestinal contents, 16S rRNA sequencing was used to detect the gut microbiota landscape. First, the amplified V3–V4 region sequences were mixed and purified using the GeneJET Gel Extraction Kit (Thermo Scientific, United States) to generate the sequencing libraries. Then the NovaSeq PE250 platform (Novogene, Beijing, China) was used to finish the sequencing. Subsequently, we used FLASH (V1.2.71) software and QIIME (V1.7.02) software to merge paired-end reads and conducted the quality control of the tags, respectively. After that, the classification was completed based on the Greengenes database 3, and then these sequences (similarity ≥97%) were assigned to the same operation taxonomic units (OTUs).
Then the QIIME (version 1.7.0) software was used to calculate the alpha diversity index and Unifrac distance. PCoA (principal coordinate analysis) was conducted using Vegan (version 2.5.4) and ade4 package (version 1.7.13). Differences in abundance were determined by the linear discriminate analysis effect size (LEfSe) and the threshold for the LDA score was 4.0. After that, Tax4Fun (version 0.3.1) software was used to conduct functional prediction based on the KEGG database and the SILVA database.49–51
H&E and IHC staining
Testicular and intestinal tissues were placed in fixation solution overnight in a refrigerator at 4 °C. And these tissues were embedded in paraffin and 5 μm sections were prepared following a standard procedure of gradient rehydration and antigen retrieval.52 Subsequently, the sections were used for hematoxylin eosin (HE) and immunohistochemistry (IHC) staining as described previously.53 Therein, HE staining was used to detect the condition of germ cells in seminiferous tubules and the structure of intestinal microvilli; IHC was used to detect the alteration of intestinal tight junctions and the number of testicular germ cells. The images of the sections were obtained using an Olympus fluorescence microscope (BX51, Japan) and antibodies used in this study are listed in Table S1.†
RNA extraction and real-time fluorescence quantitative PCR
Total RNA was extracted from intestinal tissue using the SPARKscript II RT Plus Kit (HAOSAIL, AG0304-A, Qingdao, China) according to the manufacturer's instructions. The TransScript One-Step gDNA Removal Kit and cDNA Synthesis Kit (TransGen Biotech, AT311, Beijing, China) were used to perform reverse transcription. Then, gene expression was detected using a Light Cycler real-time PCR instrument (Roche LC96; Basel, Switzerland). GAPDH was used to complete standard normalization. 2−ΔΔCT was used to calculate the relative transcript abundance according to the SYBR Green user manual (Takara, RR820A, Japan). In this part, the expression of related genes (Claudin1, Claudin3, Claudin5 and Pmp22) was used to detect the alterations of intestinal tight junctions, and the corresponding primer sequences used in this study are listed in Table S2.†
Statistical analysis
All experiments were repeated at least 3 times and the results were expressed as the mean ± SD. Data were statistically tested by Student's t-test or one-way analysis of variance (ANOVA) followed by Tukey's multiple comparison test and we defined p < 0.05 as a significant difference (*), while p < 0.01 was a highly significant difference (**).
Results
CPs treatment restored busulfan-impaired spermatogenesis
Consistent with our previous study,38 busulfan could damage the germ cells in seminiferous tubes, and it was found that CPs treatment restored busulfan-impaired spermatogenesis, as evidenced by the increased number of germ cells, reduced vacuoles in seminiferous tubules and improved sperm quality in the busulfan-exposed mice (Fig. 1).
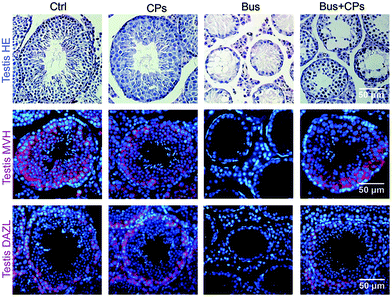 |
| Fig. 1 Chestnut polysaccharides (CPs) increased the germ cell quantity. Histopathology photos of HE staining and MVH and DAZL staining of mouse testes. | |
CPs treatment altered the gut microbiota composition
According to the experimental design, we next detected the gut microbiota composition. Three-dimensional principal coordinate analysis (PCoA) (Fig. 2A) and random forest classification (Fig. S3B†) revealed that the four groups were well separated from each group. The richness of bacterial species in different groups is shown (Fig. S2A and S3A†). The ecological diversity of the microbial community was determined using the α-diversity index including Observed, Chao1, Shannon, and Simpson. The Shannon, Chao1, and Simpson indices showed lower species richness and evenness in the Bus group than in the other groups, and this phenomenon suggests that the busulfan treatment disturbed the balance of the gut microbiota (Fig. 2B).
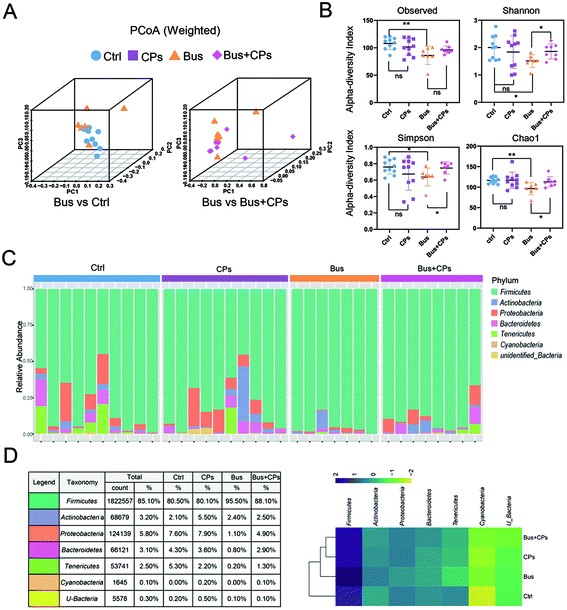 |
| Fig. 2 The changes of gut microbiota in different treatments. (A) The three-dimensional PCoA of unweighted UniFrac distances for the Ctrl vs. Bus group and Bus vs. Bus + CPs 0.1 group. (B) Alpha diversity indexes of gut microbiota, observed, Shannon, Simpson, and Chao1. (C) The relative abundance of microbiota at the phylum level. (D) The table and heatmap of the relative richness of microbiota at the phylum level. | |
The phylum and genus information was used to illustrate the differences in the levels of abundant taxa between treatments, indicating differences in the composition between different treatments. Fig. 2C and Fig. S2B† show the phylum and genus level details respectively and the relative abundance of OUT is shown in Fig. 2D. From these figures, it can be seen that the pattern of intestinal bacterial diversity was changed and the diversity was declined after busulfan treatment. Among the four groups, the Firmicutes was the dominant phylum, followed by Proteobacteria, Bacteroidetes, Actinobacteria, Tenericutes, Cyanobacteria, and unidentified bacteria. However, the busulfan treatment group had lower contents of Proteobacteria, Bacteroidetes and Tenericutes and a higher content of Firmicutes than the other groups (Fig. 2D and Fig. S3C†). At the genus level, the heatmap of the genus showed differences among the different treatment groups and Lactobacillus was the predominant genus existing in all groups (Fig. 3).
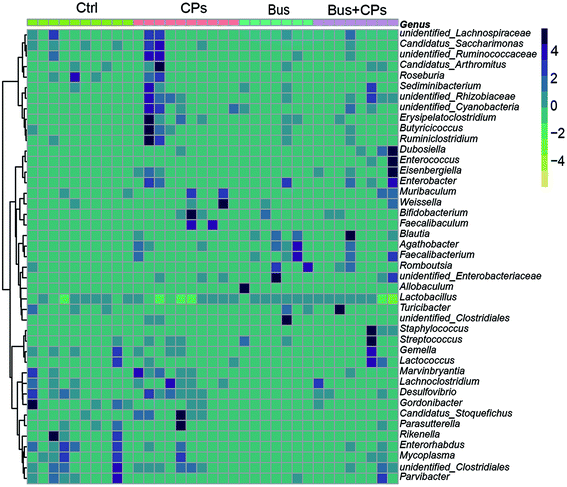 |
| Fig. 3 The heatmap of microbiota at the genus level. | |
LEfSe was used to compare the effect of CPs on the gut microbiota and to evaluate the bacterial composition between different treatment groups at different taxonomic levels (LDA value of 4) (Fig. 4A–D). The gut microbiota composition changes in the intestine were great. There were many differences in taxa in Ctrl vs. Bus groups and Bus vs. Bus + CPs groups. The abundance of Lactobacillalaceae and Anaeroplasmatales was higher in Ctrl and Bus + CPs groups, and the abundance of Pseudomonadales and Mycoplasmatales in Ctrl and Bus + CPs groups was higher than that in the Bus group.
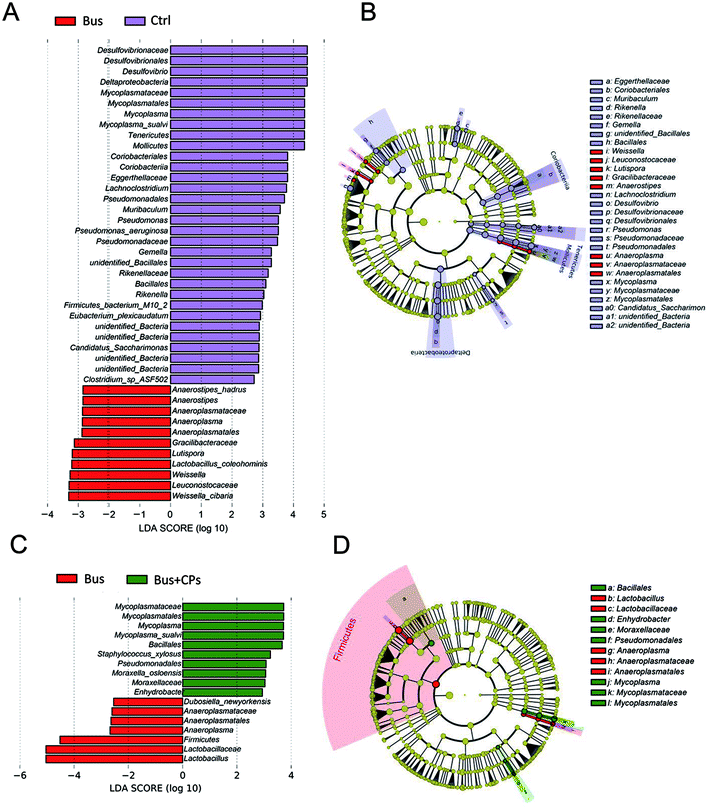 |
| Fig. 4 Differences in the bacterial abundance between different groups: (A) LDA distribution of the Ctrl group vs. CPs group with the LDA score threshold of 4.0. (B) Cladogram of the Ctrl group vs. CPs group. The linear discriminant analysis effect size (LEfSe) was used to determine the differences in microbiota abundance; the same color represents the same microbial taxon, and the dot size reflects the relative abundance at the same microbial taxonomic level; (C) LDA distribution of the Bus group vs. Bus + CPs 0.1 group with the LDA score threshold of 4.0. (D) Cladogram of the Bus group vs. Bus + CPs group. Linear discriminant analysis effect size (LEfSe) was used to determine the differences in microbiota abundance; the same color represents the same microbial taxon, and the dot size reflects the relative abundance at the same microbial taxonomic level. | |
CPs treatment influenced the intestinal structure
It is well known that the integrity of the intestinal epithelial barrier is critical for maintaining a healthy symbiosis between the epithelial cell layer and intestinal microbes, and tight junction proteins play a key role in maintaining the integrity of the epithelial barrier. In the course of the experiment, we unexpectedly found changes in the intestinal structure of the four groups (Fig. 5A), which was confirmed by the differential expression of several tight junction markers (claudin 1, Zo-1, occludin, and desmoglein 2). As shown in Fig. 5A and B, the expression of these proteins decreased after exposure to busulfan, and CPs could reverse this trend and increase the expression of these representative proteins. The results of RT-qPCR also confirmed the trend that busulfan decreased tight junctions in the intestine and CPs could reverse this trend (Fig. 5C).
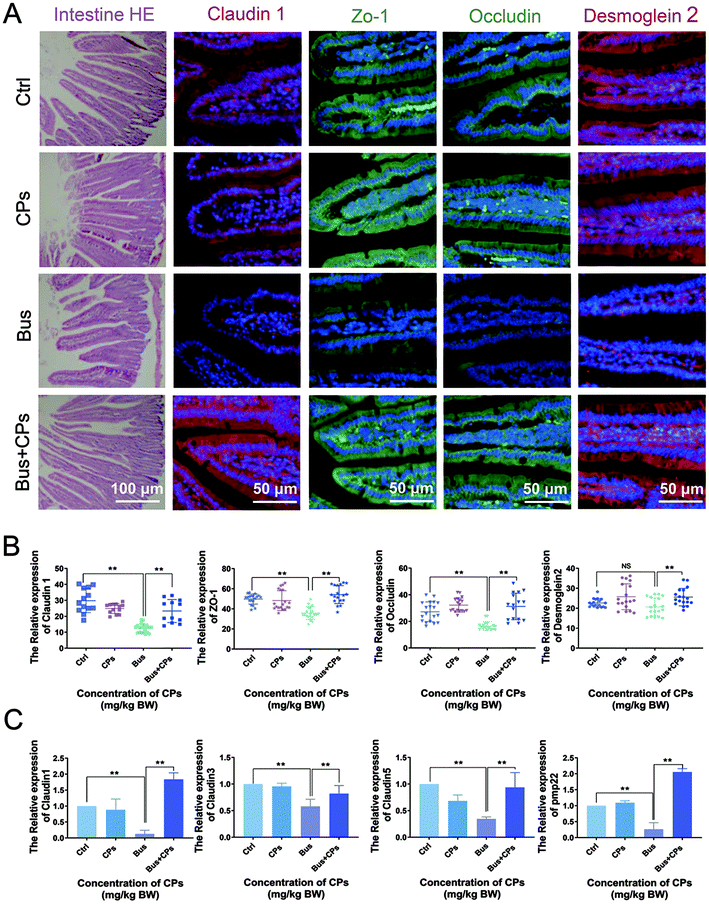 |
| Fig. 5 CPs effects on the expression of intestine tight junction markers in mice. (A) The representative images of HE staining, claudin1, Zo-1, occludin1 and desmoglein 2 in the Ctrl, CPs, Bus, and Bus + CPs groups. (B) Analysis of the fluorescence intensity of claudin1 (Ctrl: 29.82 ± 7.52, CPs: 24.94 ± 3.11, Bus: 12.59 ± 2.74, Bus + CPs: 23.34 ± 8.57), Zo-1 (Ctrl: 49.73 ± 5.03, CPs: 48.20 ± 9.91, Bus: 35.77 ± 6.23, Bus + CPs: 54.19 ± 8.93), occludin1 (Ctrl: 27.16 ± 7.45, CPs: 32.24 ± 5.33, Bus: 16.51 ± 3.20, Bus + CPs: 31.22 ± 9.96) and desmoglein 2 (Ctrl: 22.64 ± 2.14, CPs: 25.76 ± 6.47, Bus: 20.70 ± 4.47, Bus + CPs: 25.57 ± 4.36) in different groups. (C) The expression of Cldn1, Cldn3, Cldn5, and Pmp22 by RT-qPCR. The results are presented as mean ± SD. **P < 0.01. | |
CPs treatment restored impaired spermatogenesis via the steroid hormone synthesis process
Subsequently, we used functional analysis to explore this process in depth and the results showed that gut microbiota alterations are related to many functional pathways such as cellular processes, diseases, and organismal systems (Fig. 6A). Then based on the Second Level KEGG pathway analysis, we found that there are many common pathways between the Ctrl vs. Bus group and the Bus vs. Bus + CPs group (Fig. 6B–D and Fig. S4A and S4B†). After that, the steroid hormone biosynthesis function was greatly altered in the Bus group compared to the Bus + CPs group by Third Level KEGG pathway analysis, which is similar to the theory in our previous study, where CPs restored busulfan-impaired spermatogenesis by increasing the levels of proteins associated with hormone production. Thereafter, the expression of several hormone biosynthesis-related proteins, including CYP17A1 and HSD17β1, was detected by IHC. From the results, it was shown that the expression of CYP17A1 and HSD17β1 decreased in the busulfan-exposed group, while CPs alleviated this trend and increased the protein expression (Fig. 7A and B). These results indicated that gut microbiota participated in regulating spermatogenesis via steroid hormone biosynthesis.
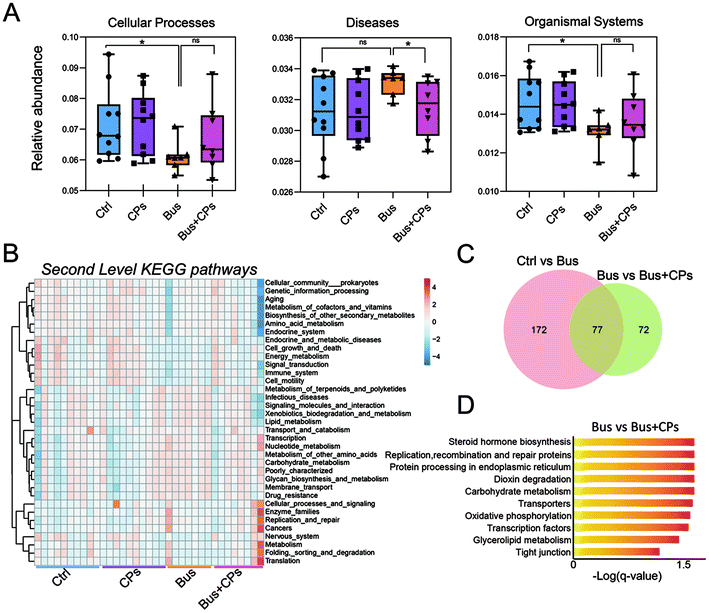 |
| Fig. 6 The analysis of the functional pathway. (A) The representative KEGG pathways at the first classification level. (B) The heat map of annotated KEGG pathways at the second classification level. (C) The Venn diagram in the Ctrl group vs. CPs group and the Bus group vs. Bus + CPs group. (D) Functional pathways at the third classification level in the Bus group vs. Bus + CPs group. | |
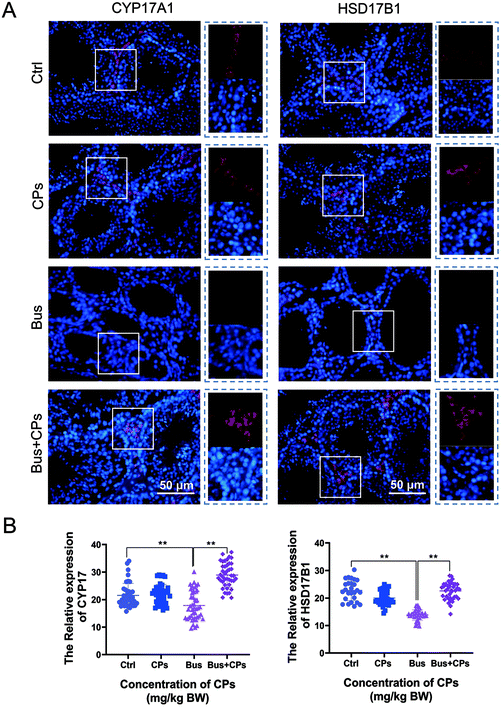 |
| Fig. 7 The changes of hormone synthesis proteins in the testes. (A) The images of HSD17β1 and CYP17 in the Ctrl, CPs, Bus, and Bus + CPs groups. (B) Analysis of the fluorescence intensity of CYP17 (Ctrl: 21.53 ± 4.33, CPs: 21.98 ± 3.52, Bus: 17.88 ± 5.22, Bus + CPs: 28.95 ± 4.47) and HSD17β1 (Ctrl: 22.77 ± 3.71, CPs: 19.99 ± 2.59, Bus: 13.85 ± 1.89, Bus + CPs: 22.60 ± 3.12) in different groups. The results are presented as mean ± SD. **P < 0.01. | |
Discussion
In recent years, research related to gut microbiota has attracted worldwide attention, and accumulating studies have shown that gut microbiota is closely related to reproductive health. For example, there is a study suggesting that the change of B. vulgatus results in increased disruption of ovarian functions.54 Our previous study confirmed the beneficial effects of CPs on the spermatogenesis process, but the exact mechanism is not clear. So we designed this experiment as shown in Fig. S1† to find out the specific mechanism of CPs in restoring impaired spermatogenesis from the gut microbiota perspective. In the present study, we speculated that CPs restore impaired spermatogenesis by adjusting gut microbiota and the results also confirmed our speculation. Interestingly, in addition to the change of gut microbiota, we also found that CPs treatment influenced the intestinal structure, especially tight junctions. Finally, by combining the functional analysis and corresponding experiments, we proved that the steroid hormone biosynthesis process is the major metabolic pathway in the CPs-mediated restoration process.
Several studies have demonstrated the importance of balanced gut microbiota in maintaining normal reproductive function. This study further validated the importance of gut microbiota in ensuring the normal spermatogenesis process. As a continuation of our previous research, in this study, we adopted the high-throughput 16S rRNA sequencing method to study the effects of CPs on restoring the impaired spermatogenesis by adjusting the imbalanced gut microbiota in busulfan-treated mice. The results of 16S rRNA sequencing showed that although Firmicutes, Bacteroidetes, Proteobacteria and Actinobacteria were common in all groups, the composition of these microbes was different. Compared with the control group, busulfan disrupted the homeostasis of gut microbiota and resulted in significant changes in the gut microbiota composition at the phylum and genus levels. This phenomenon is consistent with previous reports.36 Furthermore, the composition of the gut microbiota was altered after CPs treatment in the busulfan-treated mice, and the mice treated with the combination of Bus + CPs had bacterial abundance similar to that in the control group. For example, both the Bacteroidetes and Proteobacteria proportions decreased in the busulfan-treated mice, but increased in the Bus + CPs mice compared to the busulfan-treated group. These results proved that CPs may restore the impaired spermatogenesis by adjusting the imbalanced gut microbiota in busulfan-treated mice. Besides, at the bacteria genus level, we found that the predominant genus in all treated groups was Lactobacillus, which is consistent with the previous study55 and proved the accuracy of our analysis.
Interestingly, in addition to the changes in gut microbiota, we unexpectedly found that CPs treatment had an effect on the intestinal structure, especially tight junctions. As we all know, the integrity of the intestinal epithelial barrier is critical for maintaining a healthy symbiosis between the epithelial cell layer and intestinal microbes,56 and tight junction proteins play a key role in maintaining the integrity of the epithelial barrier.57 Besides, it was reported that tight junctions in the intestine are related to microbiota transplantation.58 In this study, we found that busulfan damaged the intestinal tight-junction function and CPs reversed this phenomenon to increase the expression of tight-junction related proteins. Combined with the CPs-mediated gut microbiota alteration phenomenon, it might indicate that the effect of CPs on the intestinal tight-junction function is closely associated with the CPs-mediated gut microbiota alteration. Similarly, many previous studies revealed that gut microbiota fluctuation is accompanied by tight-junction marker changes,34 which also proved the reliability of our results.
As described previously, CPs treatment may reverse the busulfan-induced disturbances in the spermatogenesis process and improve the sperm quality through steroid hormone biosynthesis.38 Based on the third level functional analysis, the present study further confirmed that CPs may restore impaired spermatogenesis via steroid hormone biosynthesis after the alteration of gut microbiota. Besides, we found that steroid hormone synthesis protein levels were elevated in the Bus + CPs group compared to those of the busulfan-treated group, which is in good agreement with our previous findings.38 Some studies have revealed that the alteration of the gut microbiota composition not only disturbs the reproductive function, but also regulates the steroid hormone production in men or women.59 Abnormal hormone production can lead to the obstruction of spermatogenesis.60–62 The results of the above studies prove the validity of our results to some extent, but further validation is needed to accurately prove the conclusion, such as through metabolome determination,63 which will also be the focus of our later studies.
Availability of data materials
The microbiota raw sequencing data generated in this study have been uploaded to the Genome Sequence Archive (GSA) with the accession number CRA004367 that are publicly accessible at https://ngdc.cncb.ac.cn/gsa.64,65
Author contributions
Zhong-Yi Sun and Wei Shen designed the study methods, Shuai Yu wrote the manuscript, and Yu Tian analyzed the data. Bao-Quan Han, Yu-Jiang Sun and Yong Zhao collected the samples. Ya-Qi Li and Yan Wang prepared the figures.
Abbreviations
IHC | Immunochemistry |
RT-qPCR | Real-time fluorescence quantitative PCR |
CPs | Chestnut polysaccharides |
Busulfan | Bus |
PCOS | Polycystic ovary syndrome |
GDM | Gestational diabetes mellitus |
PE | Early preeclampsia |
AOS | Alginate oligosaccharides |
OTUs | Operational taxonomic units |
PCoA | Principal coordinate analysis |
LEfSe | Linear discriminate analysis effect size. |
Conflicts of interest
There are no conflicts to declare.
Acknowledgements
This work was supported by the Shenzhen Sanming Project (SZSM201612066), the Agricultural Breeding Engineering and Major Agricultural Application Technology Innovation Project (SD2019XM008) and the Taishan Scholar Construction Foundation (ts20190946) of Shandong Province of China.
References
- X. Chen, Y. Fang, K. Nishinari, H. We, C. Sun, J. Li and Y. Jiang, Physicochemical characteristics of polysaccharide conjugates prepared from fresh tea leaves and their improving impaired glucose tolerance, Carbohydr. Polym., 2014, 112, 77–84 CrossRef CAS PubMed.
- X. Q. Chen, Z. F. Zhang, Z. M. Gao, Y. Huang and Z. Q. Wu, Physicochemical properties and cell-based bioactivity of Pu'erh tea polysaccharide conjugates, Int. J. Biol. Macromol., 2017, 104, 1294–1301 CrossRef CAS PubMed.
- Y. Jiang, X. Qi, K. Gao, W. Liu, N. Li, N. Cheng, G. Ding, W. Huang, Z. Wang and W. Xiao, Relationship between molecular weight, monosaccharide composition and immunobiologic activity of Astragalus polysaccharides, Glycoconjugate J., 2016, 33, 755–761 CrossRef CAS PubMed.
- Q. Peng, M. Li, F. Xue and H. Liu, Structure and immunobiological activity of a new polysaccharide from Bletilla striata, Carbohydr. Polym., 2014, 107, 119–123 CrossRef CAS PubMed.
- B. A. Duerkop, S. Vaishnava and L. V. Hooper, Immune responses to the microbiota at the intestinal mucosal surface, Immunity, 2009, 31, 368–376 CrossRef CAS PubMed.
- N. T. Porter and E. C. Martens, The Critical Roles of Polysaccharides in Gut Microbial Ecology and Physiology, Annu. Rev. Microbiol., 2017, 71, 349–369 CrossRef CAS PubMed.
- D. Sohretoglu and S. Huang, Ganoderma lucidum Polysaccharides as An Anti-cancer Agent, Adv. Anticancer Agents Med. Chem., 2018, 18, 667–674 CrossRef CAS PubMed.
- Y. M. Zhao, J. Wang, Z. G. Wu, J. M. Yang, W. Li and L. X. Shen, Extraction, purification and anti-proliferative activities of polysaccharides from Lentinus edodes, Int. J. Biol. Macromol., 2016, 93, 136–144 CrossRef CAS PubMed.
- L. Qian, W. Wang, J. Song and D. Chen, Peach gum polysaccharides improve the spermatogenesis of KKAy mice with impaired reproduction system, Am. J. Reprod. Immunol., 2017, 77 DOI:10.1111/aji.12627.
- Q. Luo, J. Li, X. Cui, J. Yan, Q. Zhao and C. Xiang, The effect of Lycium barbarum polysaccharides on the male rat's reproductive system and spermatogenic cell apoptosis exposed to low-dose ionizing irradiation, J. Ethnopharmacol., 2014, 154, 249–258 CrossRef CAS PubMed.
- M. Tang, F. Hou, Y. Wu, Y. Liu and J. Ouyang, Purification, characterization and tyrosinase inhibition activity of polysaccharides from chestnut (Castanea mollissima Bl.) kernel, Int. J. Biol. Macromol., 2019, 131, 309–314 CrossRef CAS PubMed.
- G. Chen and J. Kan, Ultrasound-assisted extraction, characterization, and antioxidant activity in vitro and in vivo of polysaccharides from Chestnut rose (Rosa roxburghii tratt) fruit, J. Food Sci. Technol., 2018, 55, 1083–1092 CrossRef CAS PubMed.
- A. Naseribafrouei, K. Hestad, E. Avershina, M. Sekelja, A. Linlokken, R. Wilson and K. Rudi, Correlation between the human fecal microbiota and depression, Neurogastroenterol. Motil., 2014, 26, 1155–1162 CrossRef CAS PubMed.
- H. Jiang, Z. Ling, Y. Zhang, H. Mao, Z. Ma, Y. Yin, W. Wang, W. Tang, Z. Tan, J. Shi, L. Li and B. Ruan, Altered fecal microbiota composition in patients with major depressive disorder, Brain, Behav., Immun., 2015, 48, 186–194 CrossRef PubMed.
- M. J. Claesson, I. B. Jeffery, S. Conde, S. E. Power, E. M. O'Connor, S. Cusack, H. M. Harris, M. Coakley, B. Lakshminarayanan, O. O'Sullivan, G. F. Fitzgerald, J. Deane, M. O'Connor, N. Harnedy, K. O'Connor, D. O'Mahony, D. van Sinderen, M. Wallace, L. Brennan, C. Stanton, J. R. Marchesi, A. P. Fitzgerald, F. Shanahan, C. Hill, R. P. Ross and P. W. O'Toole, Gut microbiota composition correlates with diet and health in the elderly, Nature, 2012, 488, 178–184 CrossRef CAS PubMed.
- P. W. O'Toole, Changes in the intestinal microbiota from adulthood through to old age, Clin. Microbiol. Infect., 2012, 18(Suppl 4), 44–46 CrossRef PubMed.
- J. M. Pickard, M. Y. Zeng, R. Caruso and G. Nunez, Gut microbiota: Role in pathogen colonization, immune responses, and inflammatory disease, Immunol. Rev., 2017, 279, 70–89 CrossRef CAS PubMed.
- B. O. Schroeder and F. Backhed, Signals from the gut microbiota to distant organs in physiology and disease, Nat. Med., 2016, 22, 1079–1089 CrossRef CAS PubMed.
- N. Garg, M. Wang, E. Hyde, R. R. da Silva, A. V. Melnik, I. Protsyuk, A. Bouslimani, Y. W. Lim, R. Wong, G. Humphrey, G. Ackermann, T. Spivey, S. S. Brouha, N. Bandeira, G. Y. Lin, F. Rohwer, D. J. Conrad, T. Alexandrov, R. Knight and P. C. Dorrestein, Three-Dimensional Microbiome and Metabolome Cartography of a Diseased Human Lung, Cell Host Microbe, 2017, 22, 705–716 CrossRef CAS PubMed.
- Y. Furusawa, Y. Obata, S. Fukuda, T. A. Endo, G. Nakato, D. Takahashi, Y. Nakanishi, C. Uetake, K. Kato, T. Kato, M. Takahashi, N. N. Fukuda, S. Murakami, E. Miyauchi, S. Hino, K. Atarashi, S. Onawa, Y. Fujimura, T. Lockett, J. M. Clarke, D. L. Topping, M. Tomita, S. Hori, O. Ohara, T. Morita, H. Koseki, J. Kikuchi, K. Honda, K. Hase and H. Ohno, Commensal microbe-derived butyrate induces the differentiation of colonic regulatory T cells, Nature, 2013, 504, 446–450 CrossRef CAS PubMed.
- E. A. Mayer, K. Tillisch and A. Gupta, Gut/brain axis and the microbiota, J. Clin. Invest., 2015, 125, 926–938 CrossRef PubMed.
- L. Rizzetto, F. Fava, K. M. Tuohy and C. Selmi, Connecting the immune system, systemic chronic inflammation and the gut microbiome: The role of sex, J. Autoimmun., 2018, 92, 12–34 CrossRef CAS PubMed.
- P. J. Torres, B. S. Ho, P. Arroyo, L. Sau, A. Chen, S. T. Kelley and V. G. Thackray, Exposure to a Healthy Gut Microbiome Protects Against Reproductive and Metabolic Dysregulation in a PCOS Mouse Model, Endocrinology, 2019, 160, 1193–1204 CrossRef CAS PubMed.
- M. K. W. Crusell, T. H. Hansen, T. Nielsen, K. H. Allin, M. C. Ruhlemann, P. Damm, H. Vestergaard, C. Rorbye, N. R. Jorgensen, O. B. Christiansen, F. A. Heinsen, A. Franke, T. Hansen, J. Lauenborg and O. Pedersen, Gestational diabetes is associated with changes in the gut microbiota composition in the third trimester of pregnancy and postpartum, Microbiome, 2018, 6, 89 CrossRef PubMed.
- L. Lindheim, M. Bashir, J. Munzker, C. Trummer, V. Zachhuber, B. Leber, A. Horvath, T. R. Pieber, G. Gorkiewicz, V. Stadlbauer and B. Obermayer-Pietsch, Alterations in Gut Microbiome Composition and Barrier Function Are Associated with Reproductive and Metabolic Defects in Women with Polycystic Ovary Syndrome (PCOS): A Pilot Study, PLoS One, 2017, 12, e0168390 CrossRef PubMed.
- R. Liu, C. Zhang, Y. Shi, F. Zhang, L. Li, X. Wang, Y. Ling, H. Fu, W. Dong, J. Shen, A. Reeves, A. S. Greenberg, L. Zhao, Y. Peng and X. Ding, Dysbiosis of Gut Microbiota Associated with Clinical Parameters in Polycystic Ovary Syndrome, Front. Microbiol., 2017, 8, 324 Search PubMed.
- P. J. Torres, M. Siakowska, B. Banaszewska, L. Pawelczyk, A. J. Duleba, S. T. Kelley and V. G. Thackray, Gut Microbial Diversity in Women With Polycystic Ovary Syndrome Correlates With Hyperandrogenism, J. Clin. Endocrinol. Metab., 2018, 103, 1502–1511 CrossRef PubMed.
- S. T. Kelley, D. V. Skarra, A. J. Rivera and V. G. Thackray, The Gut Microbiome Is Altered in a Letrozole-Induced Mouse Model of Polycystic Ovary Syndrome, PLoS One, 2016, 11, e0146509 CrossRef PubMed.
- Y. Guo, Y. Qi, X. Yang, L. Zhao, S. Wen, Y. Liu and L. Tang, Association between Polycystic Ovary Syndrome and Gut Microbiota, PLoS One, 2016, 11, e0153196 CrossRef PubMed.
- M. Insenser, M. Murri, R. Del Campo, M. A. Martinez-Garcia, E. Fernandez-Duran and H. F. Escobar-Morreale, Gut Microbiota and the Polycystic Ovary Syndrome: Influence of Sex, Sex Hormones, and Obesity, J. Clin. Endocrinol. Metab., 2018, 103, 2552–2562 CrossRef PubMed.
- V. G. Thackray, Sex, Microbes, and Polycystic Ovary Syndrome, Trends Endocrinol. Metab., 2019, 30, 54–65 CrossRef CAS PubMed.
- K. Tremellen and K. Pearce, Dysbiosis of Gut Microbiota (DOGMA)–a novel theory for the development of Polycystic Ovarian Syndrome, Med. Hypotheses, 2012, 79, 104–112 CrossRef PubMed.
- L. J. Lv, S. H. Li, S. C. Li, Z. C. Zhong, H. L. Duan, C. Tian, H. Li, W. He, M. C. Chen, T. W. He, Y. N. Wang, X. Zhou, L. Yao and A. H. Yin, Early-Onset Preeclampsia Is Associated With Gut Microbial Alterations in Antepartum and Postpartum Women, Front. Cell. Infect. Microbiol., 2019, 9, 224 CrossRef CAS PubMed.
- X. Chen, P. Li, M. Liu, H. Zheng, Y. He, M. X. Chen, W. Tang, X. Yue, Y. Huang, L. Zhuang, Z. Wang, M. Zhong, G. Ke, H. Hu, Y. Feng, Y. Chen, Y. Yu, H. Zhou and L. Huang, Gut dysbiosis induces the development of pre-eclampsia through bacterial translocation, Gut, 2020, 69, 513–522 CrossRef CAS PubMed.
- N. Ding, X. Zhang, X. D. Zhang, J. Jing, S. S. Liu, Y. P. Mu, L. L. Peng, Y. J. Yan, G. M. Xiao, X. Y. Bi, H. Chen, F. H. Li, B. Yao and A. Z. Zhao, Impairment of spermatogenesis and sperm motility by the high-fat diet-induced dysbiosis of gut microbes, Gut, 2020, 69, 1608–1619 CrossRef CAS PubMed.
- P. Zhang, Y. Feng, L. Li, W. Ge, S. Yu, Y. Hao, W. Shen, X. Han, D. Ma, S. Yin, Y. Tian, L. Min, Z. Sun, Q. Sun, H. Zhang and Y. Zhao, Improvement in sperm quality and spermatogenesis following faecal microbiota transplantation from alginate oligosaccharide dosed mice, Gut, 2021, 70, 222–225 CrossRef PubMed.
- C. Zhang, B. Xiong, L. Chen, W. Ge, S. Yin, Y. Feng, Z. Sun, Q. Sun, Y. Zhao, W. Shen and H. Zhang, Rescue of male fertility following faecal microbiota transplantation from alginate oligosaccharide-dosed mice, Gut, 2020, 70, 2213–2215 CrossRef PubMed.
- S. Yu, Y. Zhao, F. L. Zhang, Y. Q. Li, W. Shen and Z. Y. Sun, Chestnut polysaccharides benefit spermatogenesis through improvement in the expression of important genes, Aging, 2020, 12, 11431–11445 CrossRef CAS PubMed.
- Y. Feng, L. Min, W. Zhang, J. Liu, Z. Hou, M. Chu, L. Li, W. Shen, Y. Zhao and H. Zhang, Zinc Oxide Nanoparticles Influence Microflora in Ileal Digesta and Correlate Well with Blood Metabolites, Front. Microbiol., 2017, 8, 992 CrossRef PubMed.
- Y. Zhao, W. D. Zhang, X. Q. Liu, P. F. Zhang, Y. N. Hao, L. Li, L. Chen, W. Shen, X. F. Tang, L. J. Min, Q. S. Meng, S. K. Wang, B. Yi and H. F. Zhang, Hydrogen Sulfide and/or Ammonia Reduces Spermatozoa Motility through AMPK/AKT Related Pathways, Sci. Rep., 2016, 6, 37884 CrossRef CAS PubMed.
- F. Zeng, W. Chen, P. He, Q. Zhan, Q. Wang, H. Wu and M. Zhang, Structural characterization of polysaccharides with potential antioxidant and immunomodulatory activities from Chinese water chestnut peels, Carbohydr. Polym., 2020, 246, 116551 CrossRef CAS PubMed.
- M. Panahi, S. Keshavarz, F. Rahmanifar, A. Tamadon, D. Mehrabani, N. Karimaghai, M. Sepehrimanesh and H. Aqababa, Busulfan induced azoospermia: Stereological evaluation of testes in rat, Vet. Res. Forum, 2015, 6, 273–278 Search PubMed.
- G. I. Aboul Fotouh, M. M. Abdel-Dayem, D. I. Ismail and H. H. Mohamed, Histological Study on the Protective Effect of Endogenous Stem Cell Mobilization in Busulfan-Induced Testicular Injury in Albino Rats, J. Microsc. Ultrastruct., 2018, 6, 197–204 Search PubMed.
- X. Chen, M. Liang and D. Wang, Progress on the study of the mechanism of busulfan cytotoxicity, Cytotechnology, 2018, 70, 497–502 CrossRef CAS PubMed.
- M. Mirhoseini, G. Saki, M. Hemadi, A. Khodadadi and J. Mohammadi Asl, Melatonin and testicular damage in busulfan treated mice, Iran. Red Crescent Med. J., 2014, 16, e14463 Search PubMed.
- Y. J. Choi, D. W. Ok, D. N. Kwon, J. I. Chung, H. C. Kim, S. M. Yeo, T. Kim, H. G. Seo and J. H. Kim, Murine male germ cell apoptosis induced by busulfan treatment correlates with loss of c-kit-expression in a Fas/FasL- and p53-independent manner, FEBS Lett., 2004, 575, 41–51 CrossRef CAS PubMed.
- R. L. Brinster and J. W. Zimmermann, Spermatogenesis following male germ-cell transplantation, Proc. Natl. Acad. Sci. U. S. A., 1994, 91, 11298–11302 CrossRef CAS PubMed.
- S. R. Vasiliausha, F. L. Beltrame, F. de Santi, P. S. Cerri, B. H. Caneguim and E. Sasso-Cerri, Seminiferous epithelium damage after short period of busulphan treatment in adult rats and vitamin B12 efficacy in the recovery of spermatogonial germ cells, Int. J. Exp. Pathol., 2016, 97, 317–328 CrossRef CAS PubMed.
- J. G. Caporaso, J. Kuczynski, J. Stombaugh, K. Bittinger, F. D. Bushman, E. K. Costello, N. Fierer, A. G. Pena, J. K. Goodrich, J. I. Gordon, G. A. Huttley, S. T. Kelley, D. Knights, J. E. Koenig, R. E. Ley, C. A. Lozupone, D. McDonald, B. D. Muegge, M. Pirrung, J. Reeder, J. R. Sevinsky, P. J. Turnbaugh, W. A. Walters, J. Widmann, T. Yatsunenko, J. Zaneveld and R. Knight, QIIME allows analysis of high-throughput community sequencing data, Nat. Methods, 2010, 7, 335–336 CrossRef CAS PubMed.
- K. P. Asshauer, B. Wemheuer, R. Daniel and P. Meinicke, Tax4Fun: predicting functional profiles from metagenomic 16S rRNA data, Bioinformatics, 2015, 31, 2882–2884 CrossRef CAS PubMed.
- T. Magoc and S. L. Salzberg, FLASH: fast length adjustment of short reads to improve genome assemblies, Bioinformatics, 2011, 27, 2957–2963 CrossRef CAS PubMed.
- H. Liu, R. Zhu, C. Liu, R. Ma, L. Wang, B. Chen, L. Li, J. Niu, D. Zhao, F. Mo, M. Fu, D. Bromme, D. Zhang and S. Gao, Evaluation of Decalcification Techniques for Rat Femurs Using HE and Immunohistochemical Staining, BioMed Res. Int., 2017, 2017, 9050754 Search PubMed.
- P. Zhang, H. Chao, X. Sun, L. Li, Q. Shi and W. Shen, Murine folliculogenesis in vitro is stage-specifically regulated by insulin via the Akt signaling pathway, Histochem. Cell Biol., 2010, 134, 75–82 CrossRef CAS PubMed.
- X. Qi, C. Yun, L. Sun, J. Xia, Q. Wu, Y. Wang, L. Wang, Y. Zhang, X. Liang, L. Wang, F. J. Gonzalez, A. D. Patterson, H. Liu, L. Mu, Z. Zhou, Y. Zhao, R. Li, P. Liu, C. Zhong, Y. Pang, C. Jiang and J. Qiao, Gut microbiota-bile acid-interleukin-22 axis orchestrates polycystic ovary syndrome, Nat. Med., 2019, 25, 1225–1233 CrossRef CAS PubMed.
- S. Tan, W. Ge, J. Wang, W. Liu, Y. Zhao, W. Shen and L. Li, Zearalenone-induced aberration in the composition of the gut microbiome and function impacts the ovary reserve, Chemosphere, 2020, 244, 125493 CrossRef CAS PubMed.
- R. Okumura and K. Takeda, Roles of intestinal epithelial cells in the maintenance of gut homeostasis, Exp. Mol. Med., 2017, 49, e338 CrossRef CAS PubMed.
- T. Otani and M. Furuse, Tight Junction Structure and Function Revisited, Trends Cell Biol., 2020, 30, 805–817 CrossRef CAS PubMed.
- L. Ma, Y. Ni, Z. Wang, W. Tu, L. Ni, F. Zhuge, A. Zheng, L. Hu, Y. Zhao, L. Zheng and Z. Fu, Spermidine improves gut barrier integrity and gut microbiota function in diet-induced obese mice, Gut Microbes, 2020, 12, 1–19 Search PubMed.
- J. H. Shin, Y. H. Park, M. Sim, S. A. Kim, H. Joung and D. M. Shin, Serum level of sex steroid hormone is associated with diversity and profiles of human gut microbiome, Res. Microbiol., 2019, 170, 192–201 CrossRef CAS PubMed.
- L. O'Donnell, R. I. McLachlan, N. G. Wreford and D. M, de Kretser and D. M. Robertson, Testosterone withdrawal promotes stage-specific detachment of round spermatids from the rat seminiferous epithelium, Biol. Reprod., 1996, 55, 895–901 CrossRef PubMed.
- N. Sofikitis, K. Ono, Y. Yamamoto, H. Papadopoulos and I. Miyagawa, Influence of the male reproductive tract on the reproductive potential of round spermatids abnormally released from the seminiferous epithelium, Hum. Reprod., 1999, 14, 1998–2006 CrossRef CAS PubMed.
- B. R. Zirkin, Spermatogenesis: its regulation by testosterone and FSH, Semin. Cell Dev. Biol., 1998, 9, 417–421 CrossRef CAS PubMed.
- Y. Yang, B. B. Misra, L. Liang, D. Bi, W. Weng, W. Wu, S. Cai, H. Qin, A. Goel, X. Li and Y. Ma, Integrated microbiome and metabolome analysis reveals a novel interplay between commensal bacteria and metabolites in colorectal cancer, Theranostics, 2019, 9, 4101–4114 CrossRef CAS PubMed.
- Y. Wang, F. Song, J. Zhu, S. Zhang, Y. Yang, T. Chen, B. Tang, L. Dong, N. Ding, Q. Zhang, Z. Bai, X. Dong, H. Chen, M. Sun, S. Zhai, Y. Sun, L. Yu, L. Lan, J. Xiao, X. Fang, H. Lei, Z. Zhang and W. Zhao, GSA: Genome Sequence Archive, Genomics, Proteomics Bioinf., 2017, 15, 14–18 CrossRef PubMed.
- C.-N. Members and Partners, Database Resources of the National Genomics Data Center, China National Center for Bioinformation in 2021, Nucleic Acids Res., 2021, 49, D18–D28 CrossRef PubMed.
Footnotes |
† Electronic supplementary information (ESI) available. See DOI: 10.1039/d1fo03145g |
‡ Co-first authors. |
|
This journal is © The Royal Society of Chemistry 2022 |