A comparative study of the degradation efficiency of chlorinated organic compounds by bimetallic zero-valent iron nanoparticles†
Received
29th October 2021
, Accepted 19th November 2021
First published on 23rd November 2021
Abstract
The efficacy of zero-valent iron nanoparticles (nZVI) in degrading aquatic pollutants could be enhanced by the deposition of other metals on their surface. This article describes the synthesis of bimetallic nZVI with palladium (nZVI/Pd), nickel (nZVI/Ni), silver (nZVI/Ag), and copper (nZVI/Cu). The obtained bimetallic nZVIs were used in the degradation of chlorinated volatile organic compounds (CVOC) such as vinyl chloride (VC), 1,2-dichloroethylene (DCE), trichloroethylene (TCE), and perchloroethylene (PCE) in spiked water and real groundwater. Bimetallic nZVIs demonstrated superior degradation efficiencies compared to pristine nanoparticles. While pristine nZVI degraded ∼6%, ∼57%, and ∼26% of DCE, TCE, and PCE (25 mg L−1, spiked water), respectively, nZVI/Pd and nZVI/Ni degraded all the contaminants completely within 24 h. In addition, nZVI/Pd and nZVI/Ni demonstrated better degradation efficiencies as compared to nZVI/Ag and nZVI/Cu. This efficiency of nZVI/Pd and nZVI/Ni was further observed in the contaminant mixture (total CVOC concentration 25 mg L−1, 24 h of degradation time frame) as well as real groundwater (taken from Novy Bydzov locality). These bimetallic nZVIs demonstrated a significant increase in the degradation efficiency (from ∼10% using pristine nZVI to 99.9% using nZVI/Pd or nZVI/Ni). Even in groundwater, which contains numerous interferants such as organic acids and sulfate anions, bimetallic nZVI/Pd, and nZVI/Ni showed excellent degradation efficiency towards PCE [complete degradation when using nZVI/Pd or nZVI/Ni (24 h)]. Both nZVI/Ag and nZVI/Cu showed only a minor improvement in degradation efficiency.
Water impact
Deposition of secondary metals on the nZVI surface can improve its aquatic contaminants degradation efficiency. However, since wastewater treatment must be done on a large scale, it is necessary to consider the efficiency to cost ratio of the nanoparticles. Therefore, despite Pd's superior efficiency, Ni might be a cost-effective option in degrading common volatile organics.
|
1. Introduction
Volatile organic compounds (VOCs) comprise many large groups of compounds, including alkanes, alkenes, and halogenated organic compounds. Modernization, coupled with industrialization, has caused an increase in the content of VOCs in the environment. This may be attributed to the generation of effluents containing VOCs by industries as well as by our daily activities. Petroleum fluids (propane, butane, pentane), hydraulic fluids (acetone, chloromethane, hexachlorobenzene), paints (dichloromethane, tert-butyl peracetate), dry cleaning agents (perchloroethylene, glycol ethers) and inks (pentane, hexane) are some of the examples of sources that could include VOCs, as are the chemicals released from the chemical industries and refineries. Further, industrial solvents like benzene, toluene, chloroform, methane, and chlorohydrocarbons also constitute VOCs.1 These chemicals are toxic, carcinogenic, mutagenic and harmful to the ecosystem and the environment. Yang et al., analyzed the tap water from Wuhan, China and identified 75-200 VOCs comprising mainly industrial and pharmaceutical compounds.2 Im et al., identified 17 VOCs including 10 halogenated VOCs at Han River basin, which is one of the important drinking water sources of South Korea.3 Analyzing the tap and bottled water from Central Missouri, Ikem et al., found trihalomethanes in concentrations exceeding the standard limits set by World Health Organization.4 These VOCs often end up in the environment as a result of improper disposal. Chlorinated volatile organic compounds (CVOC), such as vinyl chloride (VC), 1,2-dichloroethylene (DCE), trichloroethylene (TCE), and perchloroethylene (PCE), have been extensively used as solvents in cleaning, extraction, metal degreasing, pesticide production, foams, sprays and many more applications.5–7 Because of their extensive use, the presence of these compounds in air, soil, rivers, lakes, and even in groundwater is almost ubiquitous.8,9 These typically recalcitrant compounds have high density, low water solubility (Table S1†) and represent a significant danger to the environment due to their persistence in the environment because these compounds are degraded relatively slowly in environmental systems.5,8,10–12
Over the past three decades, an array of oxidative,13–16 reductive17–19 and biodegradative approaches20–22 have been developed for in situ remediation to treat CVOC in soil and water. Among them, zero-valent iron nanoparticles (nZVI) have been found to be very effective in the reduction of CVOC.11,23–33 The high reactivity of nZVI and their ability to degrade a wide range of pollutants can be attributed to their large surface area34,35 and rapid oxidation. Several factors influence the pathways and processes that occur on the nZVI's surface, including its structure and properties, the presence of different contaminants, and the hydrochemistry of the polluted water.36–38 Reductive elimination of CVOC by nZVI has been widely studied, as nZVI has the ability to degrade CVOC via hydrogenolysis and dihaloelimination. Hydrogenolysis involves a carbon–carbon or carbon–heteroatom bond cleavage, and in the case of CVOC, the replacement of the chlorine anion by hydrogen cation along with an addition of two electrons.39,40 Hydrogenolysis of higher chlorinated compounds can produce toxic by-products. On the other hand, dihaloelimination involves eliminating pairs of Cl atoms without the addition of hydrogen or electrons. This takes place on vicinal carbons by forming an additional C–C bond often termed as β-elimination. Dihaloelimination and hydrogenolysis of CVOC lead to the formation of much less toxic acetylene, which in turn may be converted to ethene and ethane.
Despite its many advantages, nZVI suffers from certain drawbacks, including rapid passivation and low reactivity.11,41 To overcome these drawbacks and improve the efficiency of nZVI, various modification techniques have been reported.42–44 Bimetallic nZVI containing traces of other metals in order to enhance their reactivity in environmental remediation is one of the most studied methods. Metal elements, including palladium (Pd), platinum (Pt), nickel (Ni), silver (Ag), and copper (Cu), have been deposited in trace amounts onto the nZVI surface to improve their efficiency in pollutant degradation.45–49 Wang and Zhang observed that bimetallic nZVI/Pd significantly improved their efficiency by completely degrading TCE (20 mg L−1) in 0.25 h compared to 1.7 h for pure nZVI (the concentration of nZVI was 20 g L−1).46 Similarly, bimetallic nZVI/Ni,50 nZVI/Pd,45 and nZVI/Cu51 demonstrated increased efficiency in comparison to pristine nZVI in the reduction of other chlorinated and organic compounds. In addition to an improvement in efficiency, the presence of these elements on the nZVI's surface alleviated their passivation while inhibiting the accumulation of corrosion products on their surface.48 Despite these advantages, the cause of their improved reaction rate is a matter of debate among researchers. Over the years, many researchers have proposed several different mechanisms. Elliott et al.52 suggested that the improvement in reaction rate was due to the formation of galvanic cells on the surface of the nZVI, whereby Fe acts as an anode and the second metal acts as a cathode. While Lowry et al.53 reported that the deposited metal acts as a catalyst in the dehalogenation reaction, enabling complete degradation of CVOC without intermediate toxic by-products. Schrick et al.54 reported the transfer of electrons onto the second metal surface, which cleaves the C–Cl bond, resulting in hydrogenolysis. Lien et al.55 and Tee et al.56 proposed the formation of atomic hydrogen on the second metal surface that acts as a reducing agent for CVOC.
Despite numerous reports suggesting the higher efficiency of bimetallic nZVI in CVOC degradation,45–51 to the best of our knowledge, there are only a few comparative studies on the efficiency of different bimetallic nZVI in synthetic and actual groundwater.57–60 Herein we report the synthesis, characterization, and comparison of bimetallic nZVI (nZVI/X, X being the additional metal) with palladium (nZVI/Pd), nickel (nZVI/Ni), silver (nZVI/Ag), and cooper (nZVI/Cu). nZVI/X were characterized by transmission electron microscopy (TEM), scanning electron microscopy (SEM), electron dispersive X-ray (EDS) mapping, X-ray diffraction (XRD), and a vibrating-sample magnetometer (VSM) and were compared for their efficiency in DCE, TCE, and PCE degradation. Furthermore, CVOC contaminated groundwater was obtained from a contaminated site (Novy Bydzov, Czech Republic) and was used to evaluate performance in the field environment which includes variables as anions, cations, and organic matter.
2. Material and methods
2.1. Reagents and solution
Iron(III) chloride hexahydrate, sodium borohydride (98%), potassium tetrachloropalladate(II) (98%), nickel(II) chloride (98%), silver nitrate (≥99%), copper(II) chloride (97%), 1,2 dichloroethylene (DCE 98%), perchloroethylene (PCE, ≥99%) and trichloroethylene (TCE, ≥99.5%) were obtained from Sigma-Aldrich. Hydrochloric acid (35%) was purchased from Lach-Ner (Czech Republic). Deionized water [DI; ELGA, Veolia Water, Marlow, UK (18.2 MΩ cm−1)] was used in all of the tests.
2.2. Preparation of nZVI and bimetallic nZVI/X
Nano zero-valent iron particles were synthesized following a modified procedure, reported previously by Wang and Zhang46 (eqn (1)). Briefly, 0.2 M of iron(III) chloride hexahydrate solution was reduced under a nitrogen atmosphere by dosing 0.5 M of sodium borohydride solution at a rate of 1.5 mL min−1 using a peristaltic pump. At the same time, the reaction mixture was mechanically stirred for 10 min (200 RPM). The synthesized nZVI particles were washed three times with ethanol and then freeze-dried for further use. | 2FeCl3 + 6NaBH4 + 18H2O → 2Fe0 + 6NaCl + 6B(OH)3 + 21H2 | (1) |
nZVI/X bimetallic nanoparticles were synthesized using a modified version of the method reported by Al-Shamsi.47 This method is one of the standard techniques to synthesize bimetallic nZVI particles.48,61 The nanoparticles were activated by acidification with hydrochloric acid (1 M) for 20 s, to which 1% (wt/wt of nZVI) Pd/Ni/Ag/Cu metal precursors were added separately and stirred for 10 min. The synthesized bimetallic nanoparticles were washed three times with DI water. An example of the deposition of a second metal on the surface of the nZVI is shown in eqn (2).46 Nanoparticles were used immediately after synthesis. | Fe0 + Pd2+ → Fe2+ + Pd0 | (2) |
2.3. Characterization
A field-emission transmission electron microscope (FEI Titan 80–300 TEM/STEM, FEI) with a super twin-lens powered at 300 kV and fitted with an annular dark-field detector was used for the TEM analysis. A scanning electron microscope (UHR FE-SEM Carl Zeiss ULTRA Plus, Germany) equipped with an energy-dispersive X-ray spectroscope (EDS) was used for the determination of the morphology/elemental composition of all nZVI samples. It was operated at an acceleration voltage of 0.5–2.5 kV. A diffractometer (Philips PW 3710/3020, Netherlands) was used for obtaining X-ray powder diffraction (XRD) patterns. For this, Cu-Kα radiation and a graphite monochromator were employed. The measurements were performed using a step-scanning program with 0.02° per step and an acquisition time of 5 s per step. X'Pert High Score software (PANanlytical BV Almelo, Netherlands) was used for analysis of the XRD data. A vibrating sample magnetometer (LAKESHORE 7407) with a maximum applied field of 20 kOe was used for performing room temperature magnetic measurements.
2.4. Analytical
Gas chromatography/ion trap MS instrument [GC–MS; Varian Saturn 3800, (CTC Analytics AG, Switzerland)], equipped with a VF-624 ms column, 60 m in length, 0.32 mm in diameter, and 1.8 μm in film thickness, was used for determination of VOCs that were searched for in the full scan mode. Helium was employed as a carrier gas with a flow rate of 1 mL min−1. At the beginning, the temperature was maintained at 45 °C for 4 min, followed by an increase of temperature until 220 °C at a rate of 9 °C min−1; the temperature was then held at 220 °C for 3 min. The pH, ORP and conductivity was determined by a WTW multimeter (TMultiLine® Multi 3430 IDS, Weilheim, Germany). The parameters listed in Table 1 were determined according to standard procedures described in the ESI† (Text S1).
Table 1 Physical and chemical parameters of groundwater from Novy Bydzov
Parameter |
Value |
Oxidation-reduction potential.
Chemical oxygen demand.
|
pH |
6.3 |
ORPa |
−106 mV |
Conductivity |
1789 μS cm−1 |
PCE |
1302 μg L−1 |
TCE |
187 μg L−1 |
DCE |
1679 μg L−1 |
VC |
179 μg L−1 |
NH4+ |
2.4 mg L−1 |
Cl− |
71 mg L−1 |
NO3− |
8.0 mg L−1 |
SO42− |
194 mg L−1 |
CODb |
5.2 mg L−1 |
Fe2+ |
5.3 mg L−1 |
2.5. VOC reduction test
Reduction tests of nZVI on CVOC were performed in a batch set-up (in 250 mL vials without headspace) in three different media. The reduction of the individual compounds DCE, TCE, and PCE was first performed in water, then in a synthetic mixture of all three CVOC (test conditions are reported below), and finally in real groundwater collected from a contaminated site (Novy Bydzov, Czech Republic).
Prior to the addition of freshly prepared nanoparticles (1 g L−1), blank solutions of the contaminants (each or all compounds together depending on the experiment at a concentration of 25 mg L−1) were stabilized for 24 h in order to observe any changes in the initial concentration. The experiments were performed at initial pH of ∼7 (no buffer), stirred at 200 rpm for 24 h at room temperature, and in triplicates (the error bars represent the standard deviation of a data set). At regular intervals, the samples for GC–MS analysis were extracted through the rubber cork covering the vials using a Hamilton syringe. The degradation (%) of the contaminants was calculated using the following equation:
| 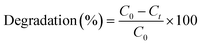 | (3) |
where
C0 is the initial concentration of contaminant,
Ct is the concentration at time
t.
2.6. Kinetic model
The kinetics of the reductive CVOC dechlorination by nZVI were estimated by a pseudo-first-order kinetic model based on the literature (eqn (4)).62,63 | 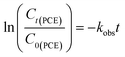 | (4) |
2.7. Groundwater parameters
The contaminated groundwater was obtained from the Novy Bydzov contaminated site (Czech Republic). The town of Novy Bydzov is located in the northeast of the Czech Republic. The site is located in the Hradec Kralove Region, Czech Republic. Improper handling of toxic chemicals therein such as chlorinated hydrocarbons, mineral oils, and other chemicals resulted in uncontrolled contamination of the quaternary aquifer. Despite having municipal drinking water supply to the majority of the households, these quaternary aquifers are sometimes used as a source of drinking water and irrigation for gardens. As a result of consuming contaminated water from such an aquifer, a person suffered a severe health condition in 2007. This site was found to be contaminated by numerous chlorinated solvents and is being remediated via bioremediation (cheese whey injected as a carbon source). The contaminated water was collected from the most contaminated well during the recent periodic sampling, and its parameters are tabulated in Table 1.
3. Results and discussions
3.1. Morphology of the nanoparticles
To obtain information on the surface morphology, particle structure/aggregation and to analyze the elemental composition of the synthesized bimetallic nanoparticles, TEM, SEM, and EDS mapping studies were performed. From the TEM images and SEM images (Fig. 1), it is observed that all nanoparticles have similar chain-like structures, which is in accordance with literature.64 This may be attributed to the magnetic effects between the small particles causing a physical attraction and to remain in the thermodynamically stable state. The EDS mapping (Fig. 1) of nZVI/X confirmed the presence of different metals on the nanoparticle surface. In addition to the presence of Fe and O, the EDS mapping revealed the presence of Pd, Ni, Ag, and Cu in nZVI/Pd, nZVI/Ni, nZVI/Ag, and nZVI/Cu, respectively. EDS (Fig. S1†) and EDS mapping further confirmed the uniform distribution of second metal over the entire nZVI/X surface.
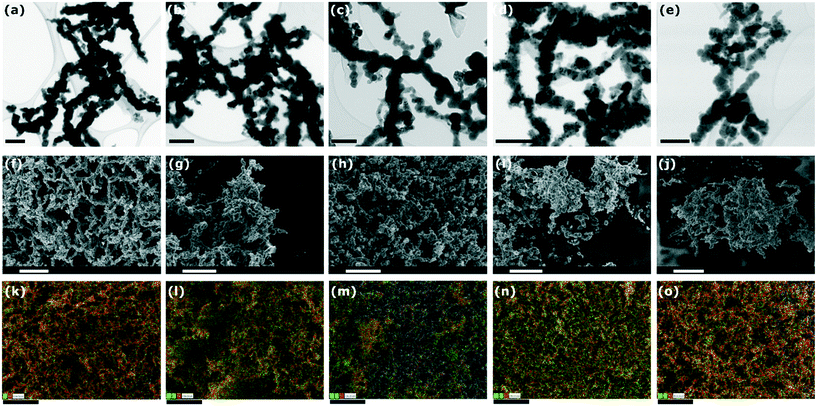 |
| Fig. 1 TEM images of (a) nZVI, b) nZVI/Pd, (c) nZVI/Ni, d) nZVI/Ag and (e) nZVI/Cu (scale bar of all the images is 200 nm). SEM images of (f) nZVI, (g) nZVI/Pd, (h) nZVI/Ni, (i) nZVI/Ag and (j) nZVI/Cu (scale bar of all the images is 1 μm). EDS mapping of k) nZVI, l) nZVI/Pd, m) nZVI/Ni, n) nZVI/Ag and o) nZVI/Cu (scale bar of all the images is 2.5 μm). In EDS mapping the red, green and turquoise color denote iron, oxygen and the second metal, respectively. | |
3.2. X-ray diffraction (XRD) analysis
The crystalline nature of nZVI was confirmed by XRD analysis (Fig. S2†). In all cases, the sharp peak located at 44.7° corresponded to bcc of Fe0. The intensity of this peak was higher for the bimetallic nZVI/X. No additional peak for nZVI/X (Ni, Cu, Ag, and Pd) was observed due to their small weight percentages present in the nZVI samples. Similar findings have been reported in the past, in which the nZVI peaks obscured the peaks of other metals present at low concentrations.48,59
3.3. Vibrating sample magnetometer (VSM)
The magnetic properties of the nZVI were examined with a VSM in an applied field sweeping from −20 to 20 kO. The relatively high magnetization may be due to the high crystallinity of the nZVI and the small layer of oxide on the nZVI surface.65 To inhibit the oxidation process that normally occurs on the nZVI surface, the samples were synthesized, collected, and stored under a nitrogen atmosphere. The obtained hysteresis loops are provided in Fig. S3.† It can be seen that all samples exhibit ferromagnetic behavior with similar magnetic saturation (Ms) and coercivity (Hc) values. A maximum Ms value of 143.9 emu g−1 and a minimum Hc value of 293 Oe were obtained for nZVI/Pd, while for the pristine nZVI nanoparticles, the Ms and Hc values were 132.9 emu g−1 and 318 Oe, respectively. All nZVI/X samples exhibit lower Hc values; this decrease in the Hc values may be due to the decrease in the content of Fe0.66–68 The slight changes could be attributed to the presence of iron oxide on the surface of the nanoparticles, which could affect the magnetic properties of the nanoparticles.69 Furthermore, the Ms values of all nZVI/X samples suggest that they can be simply separated from the mixture employing an external magnet.66,70
3.4. Degradation of CVOC
The degradation of the individual CVOC was tested in the first experimental step and the results are shown in Fig. 2. When testing the pristine nZVI, ∼6%, ∼57%, and ∼26% degradation was observed after 24 h for DCE, TCE, and PCE, respectively. With nZVI/Pd, which caused the fastest degradation, TCE was completely degraded within 30 min and DCE within 2 h. Degradation of PCE was ∼99.9% after 24 h. The second-best efficiency was observed for the nZVI/Ni nanoparticles with complete degradation of DCE within 4 h. However, for TCE and PCE, ∼74% and ∼63% degradation was observed at the 4 h mark, respectively, with complete degradation after 24 h. The degradation efficiencies of nZVI/Ag and nZVI/Cu were marginally better than those of pristine nZVI. DCE, TCE, and PCE degradation by nZVI/Ag was found to be ∼9%, ∼72%, and ∼78%, and by nZVI/Cu ∼82%, ∼69%, and ∼32%, respectively.
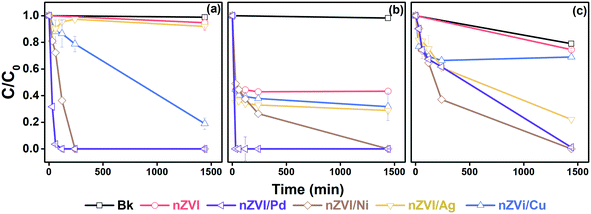 |
| Fig. 2 Comparison of different nZVI/X on the degradation of (a) DCE, (b) TCE, and (c) PCE, (nZVI/X 1 g L−1, DCE, TCE, and PCE 25 mg L−1, Bk = sample without nZVI addition). | |
Table S2† shows a comparison of the pseudo-first-order kinetic constants for the degradation of PCE by nZVI/X. As can be seen from Table S2,† nZVI/Ni shows the highest degradation rate constant (kobs = 0.245 h−1), followed by nZVI/Pd (0.184 h−1), nZVI/Ag (0.065 h−1) and nZVI/Cu (0.016 h−1) (Fig. S4†).
The test of simultaneous CVOC decontamination by different nanoparticles was evaluated and the results are shown in Fig. 3. Pristine nZVI showed an insignificant degradation efficiency (∼10%) and no subsequent changes were observed in the concentration of the pollutants even after 24 h. This may indicate the insufficient reductive efficiency of pristine nZVI under these conditions. Bimetallic nZVI/Pd drastically improved the degradation efficiencies of the nanoparticles, and the total reduction of all CVOC was observed within 60 min of the reaction. This result agrees with previous studies, where Pd demonstrated remarkable results compared to other metals.71,72 In the case of nZVI/Ni, complete degradation of TCE and DCE (under the detection limit, <1 μg L−1) and ∼92% degradation of PCE was observed within 4 h; however, PCE was completely degraded within 24 h. These results suggest that Ni may be a good alternative to Pd to be used along with nZVI for dechlorination of CVOC due to the lower price compared with Pd (Table S3†). The probable release of Ni ions from nZVI/Ni might pose an environmental issue, but the Ni in the nZVI/Ni is galvanically protected while the nZVI is oxidized.73 Schrick et al. observed that the electronic contact between the nZVI and Ni inhibits the Ni oxidation, making it stable for a longer time.54 Bimetallic nZVI/Cu demonstrated a better degradation efficiency than nZVI; however, only ∼42%, ∼73%, and ∼39% degradation of PCE, DCE, and TCE, respectively, were observed even after 24 h. nZVI/Ag completely degraded PCE within 4 h, but the DCE and TCE concentrations were reduced by only ∼14% and ∼15%, respectively, after 24 h.
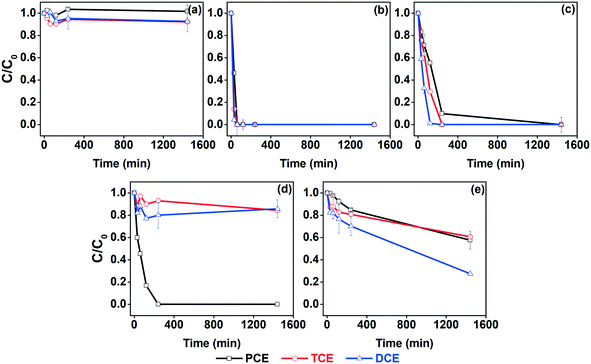 |
| Fig. 3 Degradation of CVOC mixtures by (a) nZVI, (b) nZVI/Pd, (c) nZVI/Ni, (d) nZVI/Ag and (e) NZVI/Cu (nZVI/X 1 g L−1, sum of CVOC 25 mg L−1). | |
To study the degradation efficiency in real applications, batch studies were performed with real groundwater from a contaminated site (Novy Bydzov, Czech Republic) containing various interferants (Fig. 4). The groundwater contained VC, DCE, TCE, and PCE (Table 1). A blank experiment without nZVI showed a slight decrease in the concentration of CVOC due to their high volatility. The addition of nZVI caused degradation of VC, DCE, TCE, and PCE with ∼19%, ∼27%, ∼21%, and ∼10% efficiency, respectively, after 24 h. The efficiency of nZVI/Pd and nZVI/Ni was significantly higher. Complete degradation of TCE, DCE, and VC was observed within 2 h, while ∼97% and ∼89% degradation of PCE was observed at 4 h and completely at the 24 h mark for both nZVI/Pd and nZVI/Ni. These results are comparable to the above-presented ones. Furthermore, bimetallic nZVI with Ag and Cu marginally improved the degradation efficiency.
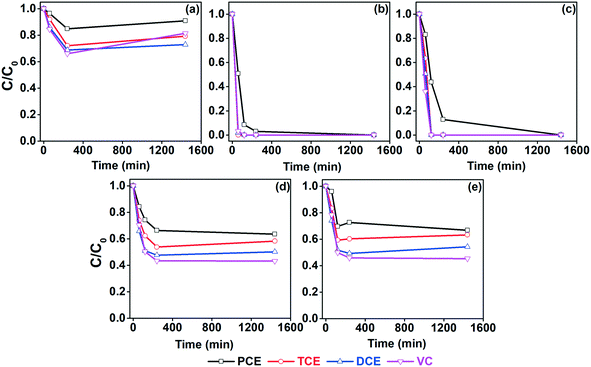 |
| Fig. 4 Degradation of CVOC in groundwater from Novy Bydzov by (a) nZVI, (b) nZVI/Pd, (c) nZVI/Ni, (d) nZVI/Ag, and (e) nZVI/Cu (nZVI/X 1 g L−1, initial CVOC concentrations are reported in Table 1). | |
In order to understand better the dechlorination process in real groundwater using the bimetallic nanoparticles, the PCE degradation rate constants of nZVI/X were compared (Fig. S5†). Fig. 5 shows the difference between the various metals used. These results further suggest that Pd and Ni may potentially improve the reactivity of nZVI. The results clearly indicated that nZVI/Pd exhibited a higher efficiency (kobs = 0.93 h−1) followed by nZVI/Ni (kobs = 0.48 h−1), nZVI/Ag (kobs = 0.11 h−1) and nZVI/Cu (kobs = 0.02 h−1). This further emphasizes the superiority of bimetallic nZVI/Pd and nZVI/Ni in the degradation of CVOC. The difference in results of rate constants between synthetic water and groundwater can be due to the poisoning of the metal catalyst surface. Because of the complexity and presence of numerous interfering ions in groundwater (especially sulfate ions at a concentration of 194 mg L−1), the surface of nanoparticles is prone to poisoning.74 This effect is more prominent in the case of nickel as compared to Pd. Due to the possible presence of Ni2+, ions can interact with the surface of the nanoparticles, as reported previously by Stumm and Morgan75 (eqn (5)).
In terms of the toxicology effect of bimetallic nZVI particles, Kim
et al.76 presented the effect of the presence of different metals on the viability of
E. coli. They reported that both bimetallic nZVI/Pd and nZVI/Ni are toxic at concentrations of 500 mg L
−1 while decreasing the concentration to 100 mg L
−1 showed a significant decrease in the toxicity. Bimetallic nZVI/Pd exhibited potentially the lowest toxicity among the other bimetallic nZVI.
76
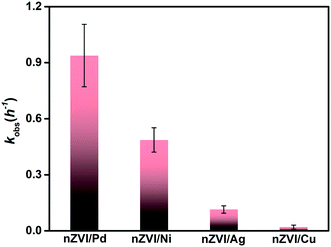 |
| Fig. 5
k
obs of PCE dechlorination by various bimetallic nZVI in real groundwater (the black error bars represent the slope error). | |
3.5. Mechanism of degradation
Herein, we elucidate the mechanism behind the CVOC degradation by bimetallic nZVI. The presence of second metal on the nZVI surface is known to reduce the activation energy of the CVOC reduction reaction.60 As a result, an increased number of interactions takes place between the CVOC and the nanoparticles, consequently increasing the rate of the reaction.77 In general, the second metal enhances the electron transfer between nZVI and the target compound,59 where nZVI acts as an electron donor and the second metal as electron collector.78 Thus, bimetallic nZVI particles effectively degrade chlorinated compounds that typically have a slower degradation rate while using nZVI. Despite this, binding strength (metal-VOC) may significantly impact VOC degradation.59 Consequently, different secondary metals exhibit different rates of CVOC degradation; Pd and Ni dehalogenate PCE quickly, while Ag and Cu have a negligible effect. It is reported that the adsorbed atomic hydrogen (Hads) on the second metal surface may also influence the reactivity of the nanoparticles.54 The Hads could be generated by H2 chemisorption or, as highlighted by Cwiertny et al.,79 Hads could also be produced as an intermediate.79 As a result, different metals' affinity for H2 chemisorption may be translated into their reactivity.31
Further, it is worth noting that degradation of PCE by nZVI/Pd and nZVI/Ni generates trace levels of TCE and DCE as intermediates (∼1 μg L−1). Due to the low activation barrier for H2 dissociation in nZVI/Pd and nZVI/Ni, atomic hydrogen is readily formed at the Pd and Ni surfaces with low cathodic hydrogen overpotentials. This atomic hydrogen reduces CVOC via a surface-mediated process (Fig. 6). CVOC is first adsorbed onto the surface of the bimetallic nanoparticles, upon which the C–Cl bond is broken, and the Cl is replaced by hydrogen, followed by the desorption of the newly dehalogenated compound.59 However, concerning nZVI/Ag, TCE and DCE were observed as intermediate by-products, while TCE was observed for nZVI/Cu. For these bimetallic nanoparticles, the surface-mediated process seems to be secondary. The primary process may be a direct reduction of CVOC on the nZVI surface. The poor catalytic ability of nZVI/Ag to degrade PCE is evident by the generation and accumulation of the by-products such as TCE and DCE (Fig. S6†). Similarly, nZVI/Cu seems to use a direct reduction process, but a catalytic reaction could also occur as evidenced by lower TCE concentration and no noticeable DCE in the reactor; nevertheless, 36% of the PCE remained in the reactor after 24 h mark suggesting its insufficient catalytic activity.
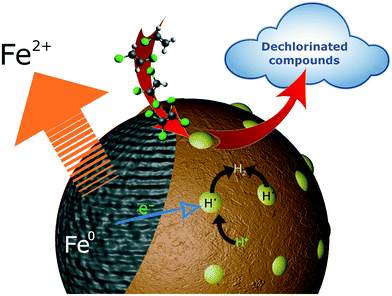 |
| Fig. 6 Schematic representation of the surface-mediated catalytic degradation of CVOC for nZVI/Pd and nZVI/Ni. | |
Furthermore, the difference in reactivity of nZVI/Pd, nZVI/Ni, nZVI/Ag, and nZVI/Cu could also arise from the surface passivation induced by iron corrosion. The surface passivation appears to have a more negligible effect on nZVI/Pd, nZVI/Ni, while nZVI/Ag and nZVI/Cu are more prone to surface passivation. Hu et al. observed that the deposition of Cu could increase the thickness of the oxide layer.80 Observations made by He et al.59 suggest that iron corrosion has a negligible effect on the reactivity of nZVI/Pd and nZVI/Ni, corroborating their higher efficiency.
The cost–benefit factor of the remediation process is also an important parameter to consider. For example, pump and treat methods and bioremediation are among the most widely used techniques for groundwater remediation. However, the pump and treat method is extremely energy-consuming, especially when treating groundwater with a minimal pollutant concentration. On the other hand, bioremediation requires specific physical–chemical conditions and is usually significantly slower. Depending on the site condition and characteristics, the use of nZVI can cut down the remediation cost by 80–90% and drastically reduce the timeframe of the remediation process.81 Using bimetallic nZVI could further reduce this timeframe, especially in the case of nZVI/Pd and nZVI/Ni (complete degradation of contaminants was observed within 24 h). Indeed, synthesis costs related to the bimetallic nZVI are higher compared to the bare nZVI (Table S3†). However, the costs associated with the number of injections, the amount of nanoparticles injected and the groundwater remediation timeframe are also considered to be significant. Due to the increased effectiveness of the bimetallic nZVI, these expenses may decrease. Moreover, the groundwater remediation time could be shortened. Therefore, considering the price of Ni (Table S3†), we propose that nZVI/Ni can serve as a very cost-effective in situ treatment of chlorinated hydrocarbons. However, further research (focused on a larger scale) is required to confirm this conclusion.
4. Conclusions
Herein, we report the efficiencies of bimetallic nZVI with various transition metals in the degradation of chlorinated organic compounds. The morphology of these nanoparticles was studied using TEM and SEM, which revealed typical nZVI morphology. The deposition of various transition metals was confirmed via EDS mapping, which showed the presence of transition metals on the nZVI surface. The efficacy of the bimetallic and pristine nZVI nanoparticles for the degradation of CVOC was further studied. The obtained results indicated that the bimetallic nZVI nanoparticles might overcome the drawbacks of pristine nZVI and delay the passivation of the nanoparticle surface, thereby significantly improving the degradation of DCE, TCE, and PCE. Bimetallic nZVI/Pd and nZVI/Ni exhibited a better degradation efficiency than nZVI incorporating Ag or Cu. In the case of both synthetic and real groundwater, nZVI/Pd and nZVI/Ni degraded the contaminants completely. Since Ni is comparatively cheaper than Pd, it may be more economically feasible to use nZVI/Ni for in situ groundwater treatment.
Conflicts of interest
There are no conflicts to declare.
Acknowledgements
The authors would like to acknowledge help of the Ministry of Education, Youth and Sports in the Czech Republic under the Research Infrastructures NanoEnviCz (Project No. LM2018124) and “Inter Excellence – Action programme” within the framework of project “Exploring the role of ferrates and modified nano zero-valent iron in the activation process of persulfates” (reg. nr: LTAUSA18078). Stanisław Wacławek also gratefully thanks for the support provided by Grant Agency of the Czech Republic (GA ČR) GJ20-17028Y nano zero-valent iron and cyclodextrins – their synergistic action for water purification. This work was also partly supported by the Student Grant Scheme at the Technical University of Liberec through project number SGS-2021-3038. D. D. Dionysiou also acknowledges support from the University of Cincinnati through the Herman Schneider Professorship in the College of Engineering and Applied Sciences.
References
- M. Meena, P. Sonigra and G. Yadav, Biological-based methods for the removal of volatile organic compounds (VOCs) and heavy metals, Environ. Sci. Pollut. Res., 2020, 28, 2485–2508 CrossRef PubMed.
- X. Yang, C. Wang, H. Shao and Q. Zheng, Non-targeted screening and analysis of volatile organic compounds in drinking water by DLLME with GC–MS, Sci. Total Environ., 2019, 694, 133494 CrossRef CAS PubMed.
- J. K. Im, S. J. Yu, S. Kim, S. H. Kim, H. R. Noh and M. K. Kim, Occurrence, Potential Sources, and Risk Assessment of Volatile Organic Compounds in the Han River Basin, South Korea, Int. J. Environ. Res. Public Health, 2021, 18, 3727 CrossRef CAS PubMed.
- A. Ikem, Measurement of volatile organic compounds in bottled and tap waters by purge and trap GC–MS: Are drinking water types different?, J. Food Compos. Anal., 2010, 23, 70–77 CrossRef CAS.
- B. Huang, C. Lei, C. Wei and G. Zeng, Chlorinated volatile organic compounds (Cl-VOCs) in environment – sources, potential human health impacts, and current remediation technologies, Environ. Int., 2014, 71, 118–138 CrossRef CAS PubMed.
- R. E. Doherty, A History of the Production and Use of Carbon Tetrachloride, Tetrachloroethylene, Trichloroethylene and 1,1,1-Trichloroethane in the United States: Part 1--Historical Background; Carbon Tetrachloride and Tetrachloroethylene, Environ. Forensics, 2000, 1, 69–81 CrossRef CAS.
- R. E. Doherty, A History of the Production and Use of Carbon Tetrachloride, Tetrachloroethylene, Trichloroethylene and 1,1,1-Trichloroethane in the United States: Part 2 – Trichloroethylene and 1,1,1-Trichloroethane, Environ. Forensics, 2000, 1, 83–93 CrossRef CAS.
- K. S. Lin, N. V. Mdlovu, C. Y. Chen, C. L. Chiang and K. Dehvari, Degradation of TCE, PCE, and 1,2–DCE DNAPLs in contaminated groundwater using polyethylenimine-modified zero-valent iron nanoparticles, J. Cleaner Prod., 2018, 175, 456–466 CrossRef CAS.
- M. J. Moran, J. S. Zogorski and P. J. Squillace, Chlorinated solvents in groundwater of the United States, Environ. Sci. Technol., 2007, 41, 74–81 CrossRef CAS PubMed.
- Y. H. Guan, J. Ma, Y. M. Ren, Y. L. Liu, J. Y. Xiao, L. Q. Lin and C. Zhang, Efficient degradation of atrazine by magnetic porous copper ferrite catalyzed peroxymonosulfate oxidation via the formation of hydroxyl and sulfate radicals, Water Res., 2013, 47, 5431–5438 CrossRef CAS PubMed.
- F. Fu, D. D. Dionysiou and H. Liu, The use of zero-valent iron for groundwater remediation and wastewater treatment: a review, J. Hazard. Mater., 2014, 267, 194–205 CrossRef CAS PubMed.
- S. Tang, X. M. Wang, Y. Q. Mao, Y. Zhao, H. W. Yang and Y. F. Xie, Effect of dissolved oxygen concentration on iron efficiency: Removal of three chloroacetic acids, Water Res., 2015, 73, 342–352 CrossRef CAS PubMed.
- M. Sunder and D. C. Hempel, Oxidation of tri- and perchloroethene in aqueous solution with ozone and hydrogen peroxide in a tube reactor, Water Res., 1997, 31, 33–40 CrossRef CAS.
- K. C. Huang, G. E. Hoag, P. Chheda, B. A. Woody and G. M. Dobbs, Oxidation of chlorinated ethenes by potassium permanganate: a kinetics study, J. Hazard. Mater., 2001, 87, 155–169 CrossRef CAS PubMed.
- N. Kang, I. Hua and P. S. C. Rao, Enhanced Fenton's destruction of non-aqueous phase perchloroethylene in soil systems, Chemosphere, 2006, 63, 1685–1698 CrossRef CAS PubMed.
- X. Gu, S. Lu, L. Li, Z. Qiu, Q. Sui, K. Lin and Q. Luo, Oxidation of 1,1,1-Trichloroethane Stimulated by Thermally Activated Persulfate, Ind. Eng. Chem. Res., 2011, 50, 11029–11036 CrossRef CAS.
-
R. Rodrigues, S. Betelu, S. Colombano, T. Tzedakis, G. Masselot and I. Ignatiadis, in Environmental Soil Remediation and Rehabilitation, Springer, 2020, pp. 283–398 Search PubMed.
- J. D. Culpepper, M. M. Scherer, T. C. Robinson, A. Neumann, D. Cwiertny and D. E. Latta, Reduction of PCE and TCE by magnetite revisited, Environ. Sci.: Processes Impacts, 2018, 20, 1340–1349 RSC.
- Y.-H. Kim and E. R. Carraway, Reductive Dechlorination of PCE and TCE by Vitamin B12 and ZVMs, Environ. Technol., 2010, 23, 1135–1145 CrossRef PubMed.
-
M. H. van Agteren, S. Keuning and D. B. Janssen, in Handbook on Biodegradation and Biological Treatment of Hazardous Organic Compounds, Springer, 1998, pp. 351–471 Search PubMed.
- G. R. Kassenga, J. H. Pardue, S. Blair and T. Ferraro, Treatment of chlorinated volatile organic compounds in upflow wetland mesocosms, Ecol. Eng., 2003, 19, 305–323 CrossRef.
-
M. H. van Agteren, S. Keuning and D. B. Janssen, in Handbook on Biodegradation and Biological Treatment of Hazardous Organic Compounds, Springer, 1998, pp. 77–187 Search PubMed.
- Y. N. Vodyanitskii, Effect of reduced iron on the degradation of chlorinated hydrocarbons in contaminated soil and ground water: A review of publications, Eurasian Soil Sci., 2014, 47, 119–133 CrossRef CAS.
- M. M. Krol, A. J. Oleniuk, C. M. Kocur, B. E. Sleep, P. Bennett, Z. Xiong and D. M. O'Carroll, A field-validated model for in situ transport of polymer-stabilized nZVI and implications for subsurface injection, Environ. Sci. Technol., 2013, 47, 7332–7340 CrossRef CAS PubMed.
- H. Tian, Y. Liang, T. Zhu, X. Zeng and Y. Sun, Surfactant-enhanced PEG-4000-NZVI for remediating trichloroethylene-contaminated soil, Chemosphere, 2018, 195, 585–593 CrossRef CAS PubMed.
- W. Xu, Z. Li, S. Shi, J. Qi, S. Cai, Y. Yu, D. M. O'Carroll and F. He, Carboxymethyl cellulose stabilized and sulfidated nanoscale zero-valent iron: Characterization and trichloroethene dechlorination, Appl. Catal., B, 2020, 262, 118303 CrossRef CAS.
- J. Farrell, M. Kason, N. Melitas and T. Li, Investigation of the Long-Term Performance of Zero-Valent Iron for Reductive Dechlorination of Trichloroethylene, Environ. Sci. Technol., 2000, 34, 514–521 CrossRef CAS.
- W. S. Orth and R. W. Gillham, Dechlorination of Trichloroethene in Aqueous Solution Using Fe0, Environ. Sci. Technol., 1995, 30, 66–71 CrossRef.
- R. W. Gillham and S. F. O'Hannesin, Enhanced Degradation of Halogenated Aliphatics by Zero-Valent Iron, Groundwater, 1994, 32, 958–967 CrossRef CAS.
- H. Song and E. R. Carraway, Reduction of Chlorinated Ethanes by Nanosized Zero-Valent Iron: Kinetics, Pathways, and Effects of Reaction Conditions, Environ. Sci. Technol., 2005, 39, 6237–6245 CrossRef CAS PubMed.
- W. Zhang, Nanoscale Iron Particles for Environmental Remediation: An Overview, J. Nanopart. Res., 2003, 5, 323–332 CrossRef CAS.
- C.-B. Wang and W. Zhang, Synthesizing Nanoscale Iron Particles for Rapid and Complete Dechlorination of TCE and PCBs, Environ. Sci. Technol., 1997, 31, 2154–2156 CrossRef CAS.
- J. Quinn, D. Elliott, S. O'Hara and A. Billow, Use of nanoscale iron and bimetallic particles for environmental remediation: A review of field-scale applications, ACS Symp. Ser., 2009, 1027, 263–283 CrossRef CAS.
- D. Fan, D. M. O'Carroll, D. W. Elliott, Z. Xiong, P. G. Tratnyek, R. L. Johnson and A. N. Garcia, Selectivity of Nano Zerovalent Iron in In Situ Chemical Reduction: Challenges and Improvements, Remediation Journal, 2016, 26, 27–40 CrossRef.
- X. Li, D. W. Elliott and W. Zhang, Zero-Valent Iron Nanoparticles for Abatement of Environmental Pollutants: Materials and Engineering Aspects, Crit. Rev. Solid State Mater. Sci., 2006, 31, 111–122 CrossRef CAS.
- W. Yan, H. L. Lien, B. E. Koel and W. X. Zhang, Iron nanoparticles for environmental clean-up: recent developments and future outlook, Environ. Sci.: Processes Impacts, 2013, 15, 63–77 RSC.
- D. O'Carroll, B. Sleep, M. Krol, H. Boparai and C. Kocur, Nanoscale zero valent iron and bimetallic particles for contaminated site remediation, Adv. Water Resour., 2013, 51, 104–122 CrossRef.
- S. Song, Y. Su, A. S. Adeleye, Y. Zhang and X. Zhou, Optimal design and characterization of sulfide-modified nanoscale zerovalent iron for diclofenac removal, Appl. Catal., B, 2017, 201, 211–220 CrossRef CAS.
- L. J. Matheson and P. G. Tratnyek, Reductive Dehalogenation of Chlorinated Methanes by Iron Metal, Environ. Sci. Technol., 1994, 28, 2045–2053 CrossRef CAS PubMed.
- W. A. Arnold and A. L. Roberts, Pathways and kinetics of chlorinated ethylene and chlorinated acetylene reaction with Fe(0) particles, Environ. Sci. Technol., 2000, 34, 1794–1805 CrossRef CAS.
- Y. Yang, L. Xu, W. Li, W. Fan, S. Song and J. Yang, Adsorption and degradation of sulfadiazine over nanoscale zero-valent iron encapsulated in three-dimensional graphene network through oxygen-driven heterogeneous Fenton-like reactions, Appl. Catal., B, 2019, 259, 118057 CrossRef CAS.
- D. Silvestri, S. Wacławek, B. Sobel, R. Torres-Mendieta, M. Pawlyta, V. V. T. Padil, J. Filip and M. Černík, Modification of nZVI with a bio-conjugate containing amine and carbonyl functional groups for catalytic activation of persulfate, Sep. Purif. Technol., 2021, 257, 117880 CrossRef CAS.
- M. Li, Y. Mu, H. Shang, C. Mao, S. Cao, Z. Ai and L. Zhang, Phosphate modification enables high efficiency and electron selectivity of nZVI toward Cr(VI) removal, Appl. Catal., B, 2020, 263, 118364 CrossRef CAS.
- Y. B. Hu and X. Y. Li, Influence of a thin aluminum hydroxide coating layer on the suspension stability and reductive reactivity of nanoscale zero-valent iron, Appl. Catal., B, 2018, 226, 554–564 CrossRef CAS.
- B. W. Zhu and T. T. Lim, Catalytic reduction of chlorobenzenes with Pd/Fe nanoparticles: Reactive sites, catalyst stability, particle aging, and regeneration, Environ. Sci. Technol., 2007, 41, 7523–7529 CrossRef CAS PubMed.
- C. B. Wang and W. X. Zhang, Synthesizing nanoscale iron particles for rapid and complete dechlorination of TCE and PCBs, Environ. Sci. Technol., 1997, 31, 2154–2156 CrossRef CAS.
- M. A. Al-Shamsi, N. R. Thomson and S. P. Forsey, Iron based bimetallic nanoparticles to activate peroxygens, Chem. Eng. J., 2013, 232, 555–563 CrossRef CAS.
- J. Feng and T. T. Lim, Iron-mediated reduction rates and pathways of halogenated methanes with nanoscale Pd/Fe: Analysis of linear free energy relationship, Chemosphere, 2007, 66, 1765–1774 CrossRef CAS PubMed.
- A. L. Roberts, L. A. Totten, W. A. Arnold, D. R. Burris and T. J. Campbell, Reductive elimination of chlorinated ethylenes by zero-valent metals, Environ. Sci. Technol., 1996, 30, 2654–2659 CrossRef CAS.
- F. Xu, S. Deng, J. Xu, W. Zhang, M. Wu, B. Wang, J. Huang and G. Yu, Highly active and stable Ni-Fe bimetal prepared by ball milling for catalytic hydrodechlorination of 4-chlorophenol, Environ. Sci. Technol., 2012, 46, 4576–4582 CrossRef CAS PubMed.
- B. Lai, Y. Zhang, Z. Chen, P. Yang, Y. Zhou and J. Wang, Removal of p-nitrophenol (PNP) in aqueous solution by the micron-scale iron-copper (Fe/Cu) bimetallic particles, Appl. Catal., B, 2014, 144, 816–830 CrossRef CAS.
- D. W. Elliott and W. X. Zhang, Field assessment of nanoscale bimetallic particles for groundwater treatment, Environ. Sci. Technol., 2001, 35, 4922–4926 CrossRef CAS PubMed.
- G. V. Lowry and M. Reinhard, Pd-catalyzed TCE dechlorination in groundwater: Solute effects, biological control, and oxidative catalyst regeneration, Environ. Sci. Technol., 2000, 34, 3217–3223 CrossRef CAS.
- B. Schrick, J. L. Blough, A. D. Jones and T. E. Mallouk, Hydrodechlorination of trichloroethylene to hydrocarbons using bimetallic nickel-iron nanoparticles, Chem. Mater., 2002, 14, 5140–5147 CrossRef CAS.
- H. L. Lien and W. X. Zhang, Nanoscale Pd/Fe bimetallic particles: Catalytic effects of palladium on hydrodechlorination, Appl. Catal., B, 2007, 77, 110–116 CrossRef CAS.
- Y. H. Tee, E. Grulke and D. Bhattacharyya, Role of Ni/Fe nanoparticle composition on the degradation of trichloroethylene from water, Ind. Eng. Chem. Res., 2005, 44, 7062–7070 CrossRef CAS.
- Y. H. Kim, E. R. Carraway and Y. H. Kim, Reductive dechlorination of TCE by zero valent bimetals, Environ. Technol., 2003, 24, 69–75 CrossRef CAS PubMed.
- C. J. Lin, S. L. Lo and Y. H. Liou, Dechlorination of trichloroethylene in aqueous solution by noble metal-modified iron, J. Hazard. Mater., 2004, 116, 219–228 CrossRef CAS PubMed.
- F. He, Z. Li, S. Shi, W. Xu, H. Sheng, Y. Gu, Y. Jiang and B. Xi, Dechlorination of Excess Trichloroethene by Bimetallic and Sulfidated Nanoscale Zero-Valent Iron, Environ. Sci. Technol., 2018, 52, 8627–8637 CrossRef CAS PubMed.
- W. X. Zhang, C. B. Wang and H. L. Lien, Treatment of chlorinated organic contaminants with nanoscale bimetallic particles, Catal. Today, 1998, 40, 387–395 CrossRef CAS.
- B.-W. Zhu and T.-T. Lim, Catalytic Reduction of Chlorobenzenes with Pd/Fe Nanoparticles: Reactive Sites, Catalyst Stability, Particle Aging, and Regeneration, Environ. Sci. Technol., 2007, 41, 7523–7529 CrossRef CAS PubMed.
- X. Chen, X. Yao, C. Yu, X. Su, C. Shen, C. Chen, R. Huang and X. Xu, Hydrodechlorination of polychlorinated biphenyls in contaminated soil from an e-waste recycling area, using nanoscale zerovalent iron and Pd/Fe bimetallic nanoparticles, Environ. Sci. Pollut. Res., 2014, 21, 5201–5210 CrossRef CAS PubMed.
-
W. Stumm and J. J. Morgan, Aquatic Chemistry : Chemical Equilibria and Rates in Natural Waters, Wiley, 2012 Search PubMed.
- A. Liu, J. Liu, J. Han and W. X. Zhang, Evolution of nanoscale zero-valent iron (nZVI) in water: Microscopic and spectroscopic evidence on the formation of nano- and micro-structured iron oxides, J. Hazard. Mater., 2017, 322, 129–135 CrossRef CAS PubMed.
- H. Yang, F. Ito, D. Hasegawa, T. Ogawa and M. Takahashi, Appl. Phys. A: Mater. Sci. Process., 2007, 101, 09J112 Search PubMed.
- Y. Su, A. S. Adeleye, Y. Huang, X. Zhou, A. A. Keller and Y. Zhang, Direct Synthesis of Novel and Reactive Sulfide-modified Nano Iron through Nanoparticle Seeding for Improved Cadmium-Contaminated Water Treatment, Sci. Rep., 2016, 6, 1–14 CrossRef PubMed.
- A. Sharma, S. Uppal, A. Arora, S. Gautam, S. Singh, R. J. Choudhary and S. K. Mehta, Magnetically retrievable Ce-doped Fe3O4 nanoparticles as scaffolds for the removal of azo dyes, RSC Adv., 2019, 9, 23129–23141 RSC.
- Z. Qi, H. Lan, T. P. Joshi, R. Liu, H. Liu and J. Qu, Enhanced oxidative and adsorptive capability towards antimony by copper-doping into magnetite magnetic particles, RSC Adv., 2016, 6, 66990–67001 RSC.
- T. Phenrat, T. Thongboot and G. V. Lowry, Electromagnetic Induction of Zerovalent Iron (ZVI) Powder and Nanoscale Zerovalent Iron (NZVI) Particles Enhances Dechlorination of Trichloroethylene in Contaminated Groundwater and Soil: Proof of Concept, Environ. Sci. Technol., 2015, 50, 872–880 CrossRef PubMed.
- L. Yang, J. Gao, Y. Liu, Z. Zhang, M. Zou, Q. Liao and J. Shang, Removal of Methyl Orange from Water Using Sulfur-Modified nZVI Supported on Biochar Composite, Water, Air, Soil Pollut., 2018, 229, 1–10 CrossRef CAS.
- F. Alonso, I. P. Beletskaya and M. Yus, Metal-mediated reductive hydrodehalogenation of organic halides, Chem. Rev., 2002, 102, 4009–4091 CrossRef CAS PubMed.
- M. Stefaniuk, P. Oleszczuk and Y. S. Ok, Review on nano zerovalent iron (nZVI): From synthesis to environmental applications, Chem. Eng. J., 2016, 287, 618–632 CrossRef CAS.
- C. Lee and D. L. Sedlak, Enhanced Formation of Oxidants from Bimetallic Nickel-Iron Nanoparticles in the Presence of Oxygen, Environ. Sci. Technol., 2008, 42, 8528 CrossRef CAS PubMed.
- D. Comandella, M. H. Ahn, H. Kim and K. Mackenzie, Enhanced protection of PDMS-embedded palladium catalysts by co-embedding of sulphide-scavengers, Sci. Total Environ., 2017, 601–602, 658–668 CrossRef CAS PubMed.
-
W. Stumm and J. Morgan, Aquatic Chemistry: Chemical Equilibria and Rates in Natural Waters, Wiley, Wiley Interscience, 3rd edn, 1995 Search PubMed.
- E. J. Kim, T. Le Thanh and Y. S. Chang, Comparative toxicity of bimetallic Fe nanoparticles toward Escherichia coli: Mechanism and environmental implications, Environ. Sci.: Nano, 2014, 1, 233–237 RSC.
- W. J. Liu, T. T. Qian and H. Jiang, Bimetallic Fe nanoparticles: Recent advances in synthesis and application in catalytic elimination of environmental pollutants, Chem. Eng. J., 2014, 236, 448–463 CrossRef CAS.
- M. Bayat, B. Nasernejad and C. Falamaki, Preparation and characterization of nano-galvanic bimetallic Fe/Sn nanoparticles deposited on talc and its enhanced performance in Cr(VI) removal, Sci. Rep., 2021, 11, 1–17 CrossRef PubMed.
- D. M. Cwiertny, S. J. Bransfield and A. L. Roberts, Influence of the Oxidizing Species on the Reactivity of Iron-Based Bimetallic Reductants, Environ. Sci. Technol., 2007, 41, 3734–3740 CrossRef CAS PubMed.
- C. Y. Hu, S. L. Lo, Y. H. Liou, Y. W. Hsu, K. Shih and C. J. Lin, Hexavalent chromium removal from near natural water by copper–iron bimetallic particles, Water Res., 2010, 44, 3101–3108 CrossRef CAS PubMed.
-
K. Grieger, R. Hjorth, A. W. Carpenter, F. Klaessig, E. Lefevre, C. Gunsch, K. Soratana, A. E. Landis, F. Wickson, D. Hristozov and I. Linkov, in Sustainable Environmental Remediation Using NZVI by Managing Benefit-Risk Trade-Offs, Springer, 2019, pp. 511–562 Search PubMed.
Footnotes |
† Electronic supplementary information (ESI) available. See DOI: 10.1039/d1ew00791b |
‡ These authors contributed equally to this work. |
|
This journal is © The Royal Society of Chemistry 2022 |