Probabilistic risk assessment of AgNPs for human health through dietary consumptions of crops†
Received
16th February 2022
, Accepted 9th July 2022
First published on 20th July 2022
Abstract
Silver nanoparticles (AgNPs) are predicted to be continuously released in the agricultural sector, the subsequent potential human health risks through consumption of food crops has raised concern. This study conducted a risk assessment for the potential human health risk resulting from AgNPs released during crop production. Taking Ireland and Europe as two spatial scopes, this risk assessment linked regional agricultural strategies to health risk induced by potential AgNP emissions, and projected future risk till 2030 based on historical data. Leafy vegetables, root and tuber vegetables, and wheat based product consumption in people's dietary structure were selected. The simulated exposure was found to be less than the reference dose (RfD) with hazard quotients (HQ) less than 0.1 indicating no potential hazardous effects resulting from released AgNPs in crop production under current and future situations. Irrigation showed a dominant impact on the final risk estimate at the EU scale through the consumption of leafy vegetables (biosolid application is not allowed for food crops). Sprinkler irrigation can elevate AgNPs on foliar surfaces of leafy vegetables. The stringent biosolid application strategy helps prevent the upstream trophic transfer of AgNPs, especially in areas with sufficient rainfall, such as in Ireland. However, areas with irrigation needs, at or above the EU average level, need to consider potential surface water irrigation from watersheds with potential AgNP accumulation. This study helps build awareness of the potential contribution of agriculture-related, regional, and crop-specific variables to human health risk potential resulting from AgNP emissions. Sources of AgNPs varied according to crop categories and are highlighted by this study as critical for risk monitoring.
Environmental significance
AgNPs have been highlighted as novel pollutants in the natural environment. However, there hasn't been a complete risk assessment to capture the risk to human health posed by potential environmental release of AgNPs. Therefore, this study firstly established a complete risk assessment for humans from AgNPs entering the crop production to the oral intake. It enhanced the instructive significance of probabilistic risk assessment by direct linking regional agricultural strategies to the output risk and projecting future risk based on historical data. Most effective risk controlling approaches have been therefore clarified for AgNPs as potential sources of health hazards.
|
1. Introduction
The continuous use of engineered silver nanoparticles (AgNPs) in the consumer market leads to their inevitable release to environmental compartments. Unique physicochemical properties of AgNPs add to the uncertainties in terms of environmental persistence, exposure potential, and hazardous potential to humans. Consequently, the potential risk to human health posed by AgNPs through environmental exposure pathways has gained growing international attention.1,2 Specific hazard characterization of AgNPs illustrated that nano-specific properties leads to more persistent toxicity potential when compared to bulk form silver. Among toxicity endpoints, oral exposure to AgNPs has been highlighted as the most critical exposure route for human health.3 The trophic transfer of environmental ENPs into crops has been estimated to result in human intake through oral exposure.4,5 In Europe, sewage sludge has been applied as soil fertilizer for growing crops and is a major disposal route for this material.6 Material flow analysis has indicated that sludge treated soil is an important compartment receiving a large amount of AgNPs.7–10 Recently, a morphology study on AgNP transformation illustrated various transformation processes on particle surfaces without the intermediate dissolution process.11 This process increased the persistence of introduced silver while also retaining its nano-scale morphology. Sulfidation has been observed extensively for AgNPs with a high silver retention rate in soil conditions, with or without sewage sludge treatment12,13 and can result in about 98% retention of silver.14 This may lead to excessive plant root exposure to AgNPs, potential plant uptake, and unexpected human exposure. Surface water is one of the most important environmental endpoints for AgNPs.15,16 The presence of AgNPs in surface water has raised concern and its risk to aquatic species has been assessed.17 As surface water is the major source of irrigation water and accounts for at least 35.5% of total irrigation water usage in Europe,18 it is necessary to consider it as a potential source of AgNPs in agricultural soil.
Concerning the exposure potential through crop consumption, bioaccumulation studies have been conducted for AgNPs in recent years.4 The bioaccumulation of AgNPs in various plants through either root or foliar exposure shows specific phytoavailability compared to that of ionic silver.19,20 Furthermore, transformed and pristine AgNPs have comparable bioavailability and trophic transfer mobility.21 In soil, root exposure to AgNPs can lead to silver uptake by roots, accumulation in root tips, and translocation to edible parts.22 Likewise, the foliage exposed to AgNPs can also result in uptake and translocated in leafy vegetables.19 Therefore, as a potential novel contaminant in the agricultural field with specific bioaccumulation properties, human exposure to AgNPs is uncertain.
During the last decade, the modeling of environmental levels of ENPs has been developed by establishing even more elaborate frameworks in terms of spatial and temporal scopes.23 These studies provide exposure information and help enable downstream risk assessment. Current risk assessments of AgNPs have been conducted for natural microbial species.17,24 To date, the trophic transfer of ENPs has been highlighted as the biggest uncertainty in assessing human exposure to ENPs.4 However, there hasn't been any quantification of the potential risk posed by AgNPs to human health resulting from crop production. This maybe because of the lack of concentration data of nanoform silver in the agricultural field and the uncertain toxicity profile of AgNPs. With the recent release of country-specific material flow analysis on AgNPs in agriculture related compartments in Europe,16 exposure assessments are feasible on a national scale. In addition, the hazard characterization of AgNPs specifically based on in vivo animal studies25 could provide dose–response information. In the EU, previous perspective studies on AgNPs used 2020 as the reference time to evaluate its environmental impact.7,8,16,26,27 This sets the background to also establish a risk assessment for potential human exposure to AgNPs through the consumption of agricultural crop produce into future.
In this regard, this study aims to create a probabilistic risk assessment of the human health risk resulting from Ag exposure through dietary intake of selected crops. To the best of authors' knowledge, this is the first work quantitatively assessing and predicting human health risk resulting from potential oral exposure through the food chain, and the first risk assessment for ENPs accounting for regional variables and agricultural regimes. Also, the collective consideration of sewage sludge and irrigation as sources of the ENP contaminant is the first attempt to include these routes in a risk assessment of ENPs. This study conducted comparative risk assessments based on current and prospective AgNP levels in two regional scopes. The development of the risk assessment framework helps bridge the bioaccumulation studies of AgNPs in crop species, AgNP predictive and measured levels, regional agriculture strategies, and AgNP toxicity studies.
2. Material and method
Three crop categories, as major contributors to the human dietary structure, were selected in this study, which are wheat, leafy vegetables, and root and tuber vegetables. According to European nutrition and health report, they are among the major groups in human food commodities.28 Other than bioavailability to AgNPs, crop-specific variables were also required including irrigation and sludge applications. Ireland and Europe are the two spatial scopes selected in this study to provide comparable assessments. Irrigation and sludge applications are also region-specific and adopted as input parameters. Data on crop-specific variables were derived from scientific studies. While for the region-specific data, the official databases (e.g., FAO, EFSA, and EC)29–31 were selected to create a framework with potential to develop further as these databases are updated over time.
Fig. 1 provides the modeling process of incorporating inputs into the risk assessment. As illustrated, from two source compartments, AgNPs flow into three agricultural compartments for crop production consisting of the irrigated soil, the sludge treated and irrigated soil, and the sprinkler water. The regional and crop-specific irrigation and sludge applications, as well as soil properties were used as input parameters to calculate the AgNP concentrations in these compartments. The bioaccumulation process varies by exposure pathway and crop categories. There are three subcategories under root and tuber vegetables with varied AgNP bioavailability. The bioaccumulation of silver in edible parts of crops were then calculated. On this basis, potential human intake was estimated by considering region-specific daily crop consumption data. Noting that engineering offers nanoform silver with various functionalities for different industrial utilities, there could be AgNPs with diverse physicochemical properties released in environmental compartments. Therefore, behavioral studies on different types of AgNPs were considered contributors to probabilistic data in this study. Hazard quotients (HQ) were modeled by considering total silver intake from the ingestion of selected crops. The input parameters were treated as probabilistic distributions to cover uncertainties surrounding the source information. Table 1 summarizes the inputs and outputs of this model.
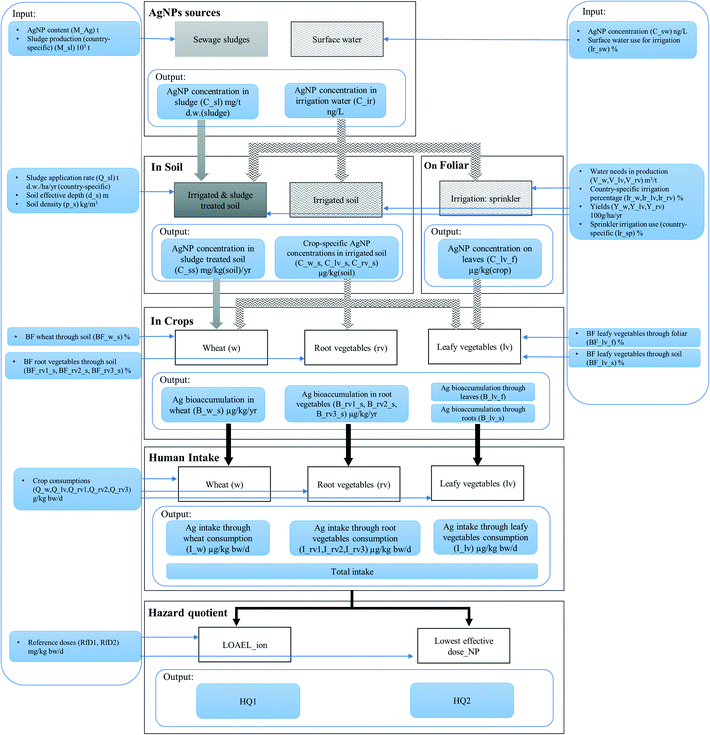 |
| Fig. 1 Framework for AgNP risk assessment model through dietary consumptions of crops. | |
Table 1 Input values and distributions for the framework model of AgNP risk assessment through dietary consumptions of crops
Symbol |
Description |
Input/calculation |
Unit |
IE |
EU |
Region-specific irrigation related parameters |
C_sw |
AgNP concentration in surface water |
Uniform (3.3, 58.9)32 |
Cumulative (0, 3200.1, 0.01–0.99, 0.2–3200) (Table S2†)33 |
ng L−1 |
Ir_sw |
Percentage of irrigation by surface water |
0.8 (Table S2†)34 |
Triangular (0, 0.90, 1) (Table S3†)34 |
% |
C_ir |
AgNP concentration in irrigation water |
Eqn (1) C_sw × Ir_sw |
ng L−1 |
Ir_sp |
Percentage of sprinkler irrigation used out of total irrigation |
0 (Table S2†)35,36 |
Triangular (0, 0, 1) (Table S3†)35,36 |
% |
Crop-specific irrigation parameters |
V_w |
Water need for wheat production |
1827 (ref. 37) |
m3 per t (crop) |
V_lv |
Water need for leafy vegetables production |
322 (ref. 37) |
m3 per t (crop) |
V_rv |
Water need for root vegetables production |
387 (ref. 37) |
m3 per t (crop) |
Ir_w |
Percentage of irrigation need out of total water needs for wheat production |
0 (Table S3†)31,38 |
0.01 (Table S4†)31,38 |
% |
Ir_lv |
Percentage of irrigation need out of total water needs for leafy vegetables production |
0.09 (Table S3†)31,38 |
0.26 (Table S4†)31,38 |
% |
Ir_rv |
Percentage of irrigation need out of total water needs of root vegetables production |
0 (Table S3†)31,38 |
0.64 (Table S4†)31,38 |
% |
Vi_w |
Irrigation need for wheat production |
Eqn (2a) V_w × Ir_w |
L per kg (crop) |
Vi_lv |
Irrigation need for leafy vegetables production |
Eqn (2b) V_lv × Ir_lv |
L per kg (crop) |
Vi_rv |
Irrigation need for root vegetables production |
Eqn (2c) V_rv × Ir_rv |
L per kg (crop) |
Y_w |
Annual yield of wheat |
101 672 (ref. 39) |
58 568 (ref. 39) |
100 g ha−1 y |
Y_lv |
Annual yield of leafy vegetable |
176 538 (ref. 39) |
198 600 (ref. 39) |
100 g ha−1 y |
Y_rv |
Annual yield of root vegetable |
0 (ref. 39) |
76 612 (ref. 39) |
100 g ha−1 y |
Vw |
Annual irrigation need per hectare for wheat production |
Eqn (3a) Vi_w × (Y_w/104) |
m3 per ha (soil) per y |
Vlv |
Annual irrigation need per hectare for leafy vegetable production |
Eqn (3b) Vi_lv × (Y_lv/104) |
m3 per ha (soil) per y |
Vrv |
Annual irrigation need per hectare for root vegetable production |
Eqn (3c) Vi_lv × (Y_lv/104) |
m3 per ha (soil) per y |
Region-specific sludge related parameters |
M_Ag |
AgNP mass content in sludge |
Table 3
|
Tonnes |
M_sl |
Total sludge production |
Table 3
|
Thousand tonnes d.w. |
C_sl |
AgNP concentration in sewage sludge |
Eqn (4) M_Ag/M_sl × 106 |
mg t−1 d.w.(biosolid) |
Q_sl |
Sludge application rate |
Uniform (0, 2) (Table S2†) |
Uniform (0, 10) (Table S3†) |
t d.w. ha−1 y |
D_sl |
AgNP annual distribution due to sewage sludge application |
Eqn (5) C_sl × Q_sl |
mg ha−1 y |
Soil properties |
d_s |
Effective depth of agricultural soil |
Triangular (0.05, 0.1, 0.3)40 |
m |
p_s |
Soil density |
Triangular (2600, 2740, 2800)41 |
kg m−3 |
Intermediate output: AgNP concentrations |
C_lv_f |
AgNP accumulation on foliar |
Eqn (6) C_ir × (Vi_lv × Ir_sp)/1000 |
μg per kg (crop) |
C_w_s |
AgNP accumulated concentration in irrigated soil for wheat production |
Eqn (7a) C_ir × (Vw/104 × (1-Ir_sp)/d_s/p_s) |
μg per kg (soil) |
C_lv_s |
AgNP accumulated concentration in irrigated soil for leafy vegetables production |
Eqn (7b) C_ir × (Vlv/104 × (1-Ir_sp)/d_s/p_s) |
μg per kg (soil) |
C_rv_s |
AgNP accumulated concentration in irrigated soil for root vegetables production |
Eqn (7c) C_ir × (Vrv/104 × (1-Ir_sp)/d_s/p_s) |
μg per kg (soil) |
C_ss |
AgNP concentration in sludge treated soil |
Eqn (8) (D_sl/d_s/104)/p_s × 1000 |
μg per kg (soil) per y |
C_w |
AgNP concentration in wheat growing soil |
Eqn (9) C_ss + C_w_s |
μg per kg (soil) |
AgNP bioaccumulation factor (BF) (crop-specific) |
BF_root |
Ag bioaccumulation factor through root exposure |
Eqn (10) Ag bioaccumulation in edible part (mg Ag per kg crop)/AgNP concentration in soil (mg Ag per kg soil) |
|
BF_foliar |
Ag bioaccumulation factor through foliar exposure |
Eqn (11) Ag bioaccumulation in edible part (mg Ag per kg crop)/AgNP accumulation on foliar (mg Ag per kg crop) |
|
BF_w_s |
Wheat through soil |
0.001 (Table S5†) |
% |
BF_lv_s |
Leafy vegetables through soil |
Triangular (0, 0.0036, 0.64) (Table S5†) |
% |
BF_lv_f |
Leafy vegetables through foliar |
0.209 (Table S5†) |
% |
BF_rv1_s |
Root and tuber vegetables through soil |
Uniform (0.05, 0.1) (Table S5†) |
% |
BF_rv2_s |
Stem/stalks vegetables through soil |
0.052 (Table S5†) |
% |
BF_rv3_s |
Sprouts, shoots and similar through soil |
Uniform (0.82, 1.80) (Table S5†) |
% |
Intermediate output: Ag bioaccumulation |
B_w_s |
Wheat through soil |
Eqn (12) C_w × BF_w_s |
μg per kg (crop) |
B_lv_s |
Leafy vegetables through soil |
Eqn (13) C_lv_s × BF_lv_s |
μg per kg (crop) |
B_lv_f |
Leafy vegetables through foliar |
Eqn (14) C_lv_f × BF_lv_f |
μg per kg (crop) |
B_rv1_s |
Root and tuber vegetables through soil |
Eqn (15a) C_rv_s × BF_rv1_s |
μg per kg (crop) |
B_rv2_s |
Stem/stalks vegetables through soil |
Eqn (15b) C_rv_s × BF_rv2_s |
μg per kg (crop) |
B_rv3_s |
Sprouts, shoots and similar through soil |
Eqn (15c) C_rv_s × BF_rv3_s |
μg per kg (crop) |
Daily crops' intake (region-specific) |
Q_w |
Daily intake of wheat and products |
Cumulative (0, 8.487, 0.05–0.975, 1.338–7.315) (Fig. S1†)29 |
Lognorm (3.08, 0.36) (Fig. S2†)29 |
g kg−1 bw d |
Q_lv |
Daily intake of leafy vegetables |
Cumulative (0, 1.234, 0.05–0.975, 0–0.96) (Fig. S1†)29 |
Loglogistic (0, 0.43, 6.64) (Fig. S2†)29 |
g kg−1 bw d |
Q_rv1 |
Daily intake of root and tuber vegetables |
Cumulative (0, 2.156, 0.05–0.975, 0–1.63) (Fig. S1†)29 |
Lognorm (0.37, 0.10) (Fig. S2†)29 |
g kg−1 bw d |
Q_rv2 |
Daily intake of stem/stalks vegetables |
Cumulative (0, 0.616, 0.05–0.975, 0–0.358) (Fig. S1†)29 |
Lognorm (0.13, 0.07) (Fig. S2†)29 |
g kg−1 bw d |
Q_rv3 |
Daily intake of sprouts, shoots and similar |
Cumulative (0, 0.206, 0.05–0.975, 0–0.124) (Fig. S1†)29 |
Expon (0.0062) (Fig. S2†)29 |
g kg−1 bw d |
Output: Ag intake through crops' consumption |
I_w |
Wheat |
Eqn (16) Q_w × B_w_s/1000 |
μg kg−1 bw d |
I_lv |
Leafy vegetables |
Eqn (17) Q_lv × (B_lv_s + B_lv_f)/1000 |
μg kg−1 bw d |
I_rv1 |
Root and tuber vegetables |
Eqn (18a) Q_rv1 × B_lv1_s/1000 |
μg kg−1 bw d |
I_rv2 |
Stem/stalks vegetables |
Eqn (18b) Q_rv1 × B_lv2_s/1000 |
μg kg−1 bw d |
I_rv3 |
Sprouts, shoots and similar |
Eqn (18c) Q_rv1 × B_lv3_s/1000 |
μg kg−1 bw d |
I_sum |
Total silver intake |
Eqn (19) I_w + I_lv + I_rv1 + I_rv2 + I_rv3 |
μg kg−1 bw d |
Output: HQ |
HQ |
Hazard quotient |
Eqn (20) I_sum/RfD |
|
2.1 AgNPs from irrigation water and irrigation parameters
Inductive coupled plasma (ICP) spectroscopy is regularly applied in quantifying AgNPs in the environment.1,30,33,42 Though the state of art SP-ICP-MS technique allows for counting nanoscale particles in complex media, it hasn't been applied widely in providing environmental concentration data.43,44 In this case, existing measurements of AgNPs in the natural environment are very diverse among regions, while the predictions cover most of the environmental compartments. In this study, given the priority on the published measurements in the natural environment, studies conducted measurements, as well as predictions of AgNP concentrations in two spatial scopes were considered. In this case, available measurements of AgNP concentrations in natural surface water within Europe were adopted (Table S2†). Detected data points at each sampling site provided by references in Table S2† were collected, and a cumulative distribution was used to capture these values (C_sw, Fig. 2a). A predicted uniform distribution of AgNP concentrations was adopted from an Irish case study32 (C_sw, Table 1).
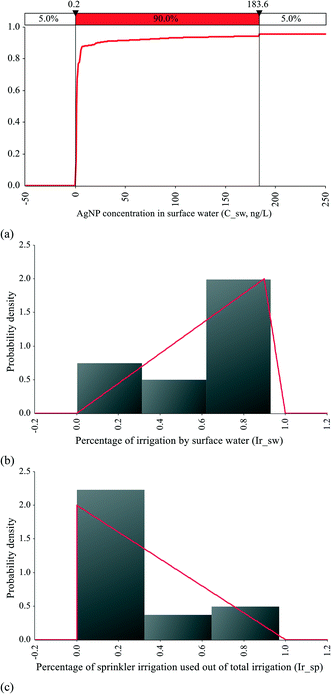 |
| Fig. 2 Best-fit distributions for irrigation related parameters: (a) measured AgNP concentrations in surface water (C_sw); (b) the percentage of surface water used for irrigation (Ir_sw); (c) the percentage of sprinkler irrigation used out of total irrigation (Ir_sp) in European scale. | |
Surface water irrigation is applied in agricultural production worldwide, and is hypothesized as a source of AgNPs in agricultural soil. The portion of surface water used for irrigation water varies according to onsite geographical and climate features. Accordingly, country-based ratios of surface water irrigation to total water used for irrigation (Ir_sw, Table 1) were adopted from the FAO's AQUASTAT database in Europe.34 This parameter was identified by the ratio of two metadata which are “area equipped for irrigation by surface water” and the “total area equipped for irrigation”. Metadata were reviewed, and abnormal values screened as described in the ESI† (Table S1). A triangular probability distribution was fitted to the data as summarized in Table S3† (Fig. 2b). This percentage was multiplied by the concentration in surface water (C_sw) resulting in the calculation of the AgNP concentrations in irrigation water (C_ir, eqn (1), Table 1).
As the amount of irrigation water used for agriculture varies according to the regional climate and soil properties, this study considered the regional irrigation water needs for different crop categories. A study of crop-specific irrigation proposed the identification of the irrigation share as the ratio of “harvested irrigated crop area” to the “total harvested crop area”.45 This approach was used in this study to determine the crop-specific percentage of irrigation needs out of total water needs for growing crops (wheat, leafy veg, and root veg, represented by Ir_w, Ir_lv, Ir_rv, respectively, as seen in Table 1). Country-based data for the harvested irrigated areas for three crop categories were available in the FAO-AQUASTAT database, from which the Irish and European levels were used (Table S4†).38 The corresponding harvested areas for three crop categories in two spatial regions were collected from the FAO-crop database (Table S4†).31 To validate whether the crop categories in databases fit the crops classification in this study, relevant categories were checked for consistency and the results were summarized in Table 2.
Table 2 Crop categories in adopted food consumption databases
Crop categories |
Labels of categories in databases |
Contained crop categories |
Edible part |
Location potentially exposed to AgNPs |
Leafy vegetable (lv) |
Vegetables |
Vegetables, fresh nes |
Leafy vegetables |
Mustard |
Leaves |
Leaves & roots |
Spinach |
Silver beet (chard) |
Lettuce |
Rocket (arugula) |
Root and tuber vegetable (rv) |
Other roots and tubers |
Roots and tubers, nes |
Root and tuber vegetables (excluding starchy- and sugar-) (rv1) |
Beetroot |
Roots and stems |
Roots |
Carrots |
Stems/stalks eaten as vegetables (rv2) |
Leek |
Sprouts, shoots and similar (rv3) |
Radish sprout |
Wheat (w) |
Wheat |
Wheat |
Grains and grain-based products |
Wheat |
Grains |
Roots |
Source database
|
FAO-AQUASTAT |
FAO-crops |
EFSA |
|
|
|
Related parameter
|
Crop-specific irrigation % calculation |
Human daily intakes |
|
|
|
By incorporating the water requirements for crop growing (Vi_w, Vi_lv, Vi_rv, m3 per t (crop), Table 1), the irrigation needs for crop production (V_w, V_lv, V_rv, L per kg (crop), Table 1) can be calculated by multiplying water requirements and irrigation fraction out of total water needs (eqn (2a–c) Table 1). A water footprint study reported water volume used for produce various kinds of crops provided the water requirements for the same crop categories as used in this study.37 The water requirement for crop production represents the biological water needs from sowing to harvesting. Maintenance of soil moisture via continuous surface irrigation is important for growing crops. The accumulation of contaminants during the irrigation period is generally considered for crops in risk assessment.46 Therefore, it is necessary to consider the total water introduction which may result in AgNP accumulation in the soil during the crops growing period. As the irrigation needs were based on the mass unit of crop production (L per kg (crop)), the calculation of total irrigation needs during crop production (Vw, Vlv, Vrv) needs to take production mass values into account. Data on yields of three crop categories (Y_w, Y_lv, Y_rv, 100 g (crop) ha−1 y) in 2017 in two spatial scopes were obtained from the FAO-crop database (Table 1).39 The calculations of Vw, Vlv, and Vrv are shown in eqn (3a–c).
2.2 AgNPs from biosolids and application parameters
To account for AgNPs contents in the biosolids, two of the most recent material flow analysis studies for the EU and respective Member States were considered as providing the most accurate evaluation of AgNP mass allocations in sludge.16,26 The mass content data (M_Ag, Table 1) were collected for the Irish and European regions. In Europe, sludge production and application data in reports and databases are represented by different Member States. Addressing this, these two material flow analysis studies16,26 considered the reliability of various data sources and assigned them scores to build uncertainty distributions. These distributions were also incorporated in this study to cover the uncertainties.
As shown by eqn (4) (Table 1), the calculation of AgNP concentrations in biosolids (dried sewage sludge) (C_sl) needs to incorporate the data of region-specific annual sludge production (M_sl). The cited studies referred to country-specific data of biosolid production to guide allocations of ENPs from WWTPs to different environmental compartments. Consistent with their data sources, annual sludge production data were retrieved from 2003 to 2020. Previous studies on AgNP mass flows extended the static model to a dynamic one by fitting historical data to regression functions which was used to project likely levels of AgNP in biosolids for future periods.9,47 By applying this method, historical data in reference years about M_Ag and M_sl were collected to examine the effect of evolving AgNP concentrations (C_sl) in biosolids on final risk potential. The regression function was used to predict the potential concentration in dried sludge (C_sl) in ten years time (assuming the current trend of increased levels of AgNP in biosolids continues). As summarized in Table 3, listed years were selected because sludge production data in these years has good coverage in Member States (EU27), and which can represent the European sludge production levels. Likewise, statistical information (e.g. mean, 25% quantile, 75% quantile values) for M_Ag in these reference years were retrieved from the reference studies.16,26 Loglogistic and lognormal distributions fit will to collected M_Ag data as detailed in Table 3.
Table 3 AgNP mass contents in sludge and sludge productions in selected years in Ireland and EU
Year |
IE |
EU |
Sludge production (thousand tonnes) |
Data source |
Fitted mass content in sludge (mg t−1) |
Ref. |
Sludge production (thousand tonnes) |
Data source |
Fitted mass content in sludge (mg t−1) |
Ref. |
2005 |
60 |
48
|
Loglogistic (0, 0.0022, 2.44) |
16
|
10 957 |
48
|
Lognorm (0.14, 0.18) |
26
|
2007 |
86.4 |
49
|
Loglogistic (0.00032, 0.0033, 2.24) |
10 136 |
50
|
Lognorm (0.23, 0.31) |
2010 |
90 |
Loglogistic (0, 0.0075, 2.5) |
9649 |
50 (prediction), 49 |
Lognorm (0.48, 0.64) |
2012 |
72.429 |
Loglogistic (0, 0.0099, 3.00) |
900 |
50 (prediction), 49 |
Lognorm (0.74, 0.78) |
2020 |
135 |
50 (prediction) |
Loglogistic (0, 0.043, 2.52) |
13 047 |
50 (prediction) |
Lognorm (2.67, 4.25) |
Though most European countries apply sewage sludge on land, as directed by Directive 86/278/EEC, more stringent restrictions for the agricultural use of sewage sludge were generally introduced on a national scale. Respective application rates of biosolids (Q_sl) on arable land in Europe were summarized in Table S3† from investigations of national legislations and application reports.6,51,52 A uniform distribution was used to cover the country-based data representing the level of application in Europe. In Ireland, the national requirement for the land use of biosolids is governed by Directive 86/278/EEC, which was also represented by a uniform distribution (Table 1). Combining the parameter C_sl, the distribution of AgNPs per hectare (D_sl) on the wheat growing land can be calculated as eqn (5) (Table 1).
2.3 AgNP concentrations
2.3.1 In sprinkler water.
As an important irrigation system for leafy vegetables, previous risk assessments have recognized sprinkler irrigation as a potential route for waterborne contaminants entering the food chain.46,53,54 Assuming when the sprinkler irrigation is necessary, the local weather is warm enough so that the loss of sprinkler water from the foliage will be due to evaporation. In other words, there is no consideration of the AgNPs' translocation from the surface of foliage to the soil in this study. Following the concept of calculating the percentage of irrigation needs (Ir_w, Ir_lv, and Ir_rv) and the percentage of surface water used in irrigation (Ir_sw), the percentage of sprinkler water usage out of total irrigation water (Ir_sp, Table 1) was identified as the ratio of two metadata: “total agricultural water managed area” and “area equipped for sprinkler irrigation”. Such country-specific data are well-documented in the FAO-AQUASTAT database and used here,35,36 and are summarized in Table S3.† A triangular distribution was used to fit the country-based results of Ir_sp to represent the European sprinkler irrigation percentage (Fig. 2c). As listed, there is no sprinkler irrigation documented for Ireland due to sufficient rainfed intensity serving as overhead water source for crops. The accumulation of contaminants on foliage of leafy vegetables is important to their final human exposure.46 Therefore, the accumulation of AgNPs due to sprinkler irrigation applied on leafy vegetables during the growing season should be considered in the exposure assessment. In this perspective, the total water used for sprinkler irrigation during crop growth was calculated (Vi_lv × Ir_sp). As no degradation was considered for AgNPs on crop foliage, the total accumulation of AgNPs on foliage (C_lv_f) was calculated as the multiplication of total water used for sprinkler irrigation and AgNP concentration in irrigation water (C_ir) (eqn (6), Table 1).
2.3.2 In irrigated soil.
Following identifications of related parameters, the calculation of AgNP concentrations in crop-specific agricultural soil after irrigation is captured in eqn (7a–c) (Table 1). The fraction of water not serving as sprinkler irrigation (1-Ir_sp) was seen as adding to the soil moisture. The crop-specific irrigation needs multiplied by this percentage resulted in surface irrigation volumes. Other than the AgNP concentration in irrigation water and the irrigation volume, the AgNP concentration in irrigated soil is also influenced by soil properties. The effective soil depth represents the vertical mobile depth after the dispersion of AgNPs on the surface layer of soil. In behavioral studies of NPs in soil, the top layer of soil (0–30 cm) was generally used for observation.12,55,56 A long-term behavioral study found that the majority of silver was retained in the top 10 cm of the biosolids treated soil.57 In a previous risk assessment, a triangular distribution considering 10 cm depth in top soil has the highest accumulation potential for heavy metals and this was also used in this study (d_s, Table 1).40 Also, a triangular distribution of soil density (ρ_s) was used41 (Table 1), covering the soil density values used in previous studies of ENPs' transport in soil.58 This range is also coherent to the topsoil density identified as good for field-crop productivity.59 This framework considers crops produced in ideal conditions of the soil. Therefore, soil density and depth (d_s, p_s) are not regional parameters. Their two triangular distributions are considered to cover the deviation derived by different crop categories. Given the d_s and p_s, the crop-specific irrigation needs per kilogram soil were calculated, which was used to convert the AgNP concentration in irrigation water (C_ir, μg m−3) to the concentration in irrigated soil (C_w_s, C_lv_s, C_rv_s, μg per kg(soil)).
2.3.3 In sludge treated soil.
According to Article 7 of Directive 86/278/EEC,30 the application of sewage sludge has been prohibited on soil growing vegetables and fruit crops among European Member States. Therefore, biosolid application on agricultural land was only considered for wheat production. The algorithm converting the AgNP distribution (D_sl, mg ha−1) to the AgNP concentration in sludge treated soil (C_ss, μg per kg(soil)) considered the soil properties (d_s, p_s) as shown as eqn (8) (Table 1).
AgNP behavioral studies have confirmed high retention rates of silver under various conditions of agricultural soil and biosolids applications.12–14,60 AgNPs in these compartments can be transformed into more stable particulates, hindering dissolution in soil pore water.11,14 Therefore, the total inflow of AgNPs in agricultural soil was regarded as being available for crop uptake. It is important to note that in this case, the identification of AgNP concentration in agricultural soil represents the worst case scenario in terms of potential human exposure.
2.4 Bioaccumulation
Currently, the uptake and retention ability of AgNPs in edible parts of plants has been studied among leafy vegetables, root and tuber vegetables, and wheat, and is generally characterized by the bioaccumulation factor (BF). The bioaccumulation of AgNPs through root exposure has been reported among selected crops.19,61–64 The crop species used in selected bioaccumulation studies were regarded as representatives of related crop categories. The algorithms used for the BF through root and foliar exposure are shown as eqn (10) and (11) (Table 1). The data on AgNP concentrations and the determinations of BFs for different types of crops through different exposure routes were summarized in Table S5.† Studies have been conducted on the bioaccumulation of AgNPs through foliar exposure of leafy vegetables.19,20 Among them, the data in one bioaccumulation study20 were used (BF_lv_f) because it provided the net silver uptake through foliar exposure and the accumulated dose of AgNPs on foliage and was used to calculate bioaccumulation through foliage (eqn (11)). A triangular distribution was used to model the BF of AgNPs from soil to leafy vegetables (BF_lv_s, Fig. 3) and uniform distributions were used to characterize the BFs of AgNPs through tubers and sprout vegetables (BF_rv1_s, BF_rv3_s). BFs for wheat from AgNPs in soil (BF_w_s) and stem/stalks vegetables through soil (BF_rv2_s) have been adopted from relevant studies, which followed the calculation algorithms as captured in eqn (10) and (11).62,64 The bioaccumulated silver in crop edible parts was calculated by multiplying respective BFs by AgNP concentrations in corresponding food product compartments (eqn (12)–(15c), Table 1).
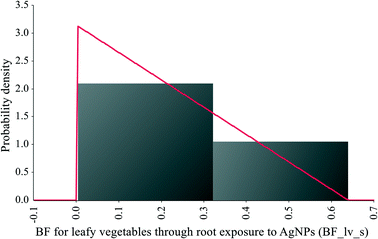 |
| Fig. 3 Best fit distribution of BF for leafy vegetables through root exposure to AgNPs (BF_lv_s). | |
2.5 Hazard characterization
2.5.1 Human intake.
Several databases by Food and Agriculture Organization (FAO), Environmental Protection Agency (EPA), and European Food Safety Authority (EFSA) document human food consumption data for European countries.29,31,65 For adult daily consumption of selected crops, the EFSA European food consumption database29 was chosen as its well-organized food categories using a hierarchical classification system. According to the guidance of EFSA, data were relatively abundant at 1st and 2nd hierarchies of food categories.66 In this study, one 1st level category and four 2nd level categories correlated to crop categories identified in this study as summarized in Table 2. The systematic classification of food categories in EFSA67 has been reviewed to ensure crop species used in this study were incorporated in adopted categories of the database. For each crop category, a cumulative distribution was used to fit the statistical data in each European country. The average of country-based distributions was used to represent the European level. The cumulative distributions of Irish data and simulated distributions of EU average data are illustrated in Fig. S1 and S2.† As shown, the lognormal, logistic, and exponential distributions fitted well to European average data and are detailed in Table 1. Eqn (16)–(18c) (Table 1) calculate silver intake through dietary consumption of different crop categories.
2.5.2 Dose–response.
The hazard quotient (HQ) is the ratio of the total intake value to the reference dose (RfD), which is adopted in this study as a risk endpoint (eqn (20), Table 1). In bioaccumulation studies, acid digestion is generally applied before analysis detecting the silver concentration in biomass, which does not allow for identification of silver forms (i.e., ionic vs. particles). However, direct uptake of the particulate form of silver has been observed in plants from a number of studies.19,68,69 As most of the selected bioaccumulation studies didn't differentiate ionic or particulate silver in crops' edible parts, the oral RfD of silver ions and AgNPs were both considered in this risk assessment. The RfD of pristine AgNPs was adopted due to the lack of the dose response relationship of transformed AgNPs such as sulfurized AgNPs. As observed by recent morphology studies, the Ag2S formation is not uniform on the surface of AgNPs in natural soil and partially retains pristine surface properties.11,70 Therefore, it is assumed that transformed and pristine AgNPs have comparable toxicity levels.
The lowest observed adverse effect level (LOAEL) of 14 μg kg−1 d reported by the report from the integrated risk information system of US environmental protection agency (USEPA IRIS) (Table 4)71 was chosen as the RfD of bulk silver. For the RfD of AgNPs, there is no official limit value and in vivo toxicity studies were considered. The hazard characterization study for AgNPs listed candidate RfDs from effective doses reported for toxic effects and converted them to human equivalent doses (HED).3 Accordingly, this study incorporated the lowest dose of 15 μg kg−1 (HED: 2 μg kg−1)72 as the other RfD of AgNPs. This subchronic oral exposure to this dose of AgNPs was observed with the reprotoxicity response.
Table 4 Reference doses for oral exposed bulk silver and AgNPs
Toxicity endpoint |
Ag form |
RfD (μg kg−1 d) |
Ref. |
LOAEL |
Bulk form |
14 |
65
|
Lowest effective dose of reprotoxicity |
NP |
2 |
72
|
2.6 Data analysis and software
As shown in Table 1, various probability distributions were used to account for variability and uncertainty surrounding different input parameters, which were assembled in Microsoft Excel (version 2107) with the @Risk 7.5.1 add-on package. Monte Carlo simulation with 10
000 iterations was used to generate output distributions. Sensitivity analysis was carried out on the total silver intake through crop consumption using the Spearman's rank order correlation to assess how the input parameters contribute to the final exposure.
3. Results and discussion
3.1 AgNP concentrations in agricultural soil
The simulated distributions for C_sl typically follow the loglogistic and lognormal shapes (Fig. S3†), which is consistent with the probability distributions of M_Ag (Table 3). Fig. 4 shows the quadratic regression curves fitted by the mean, 15th percentile (Q15), and 85th percentile (Q85) values and the prediction of the potential AgNP concentration in biosolids in 2030. The projected increase in AgNP concentrations in biosolids over time was evident both in Ireland and Europe. The calculated output AgNP concentrations in agricultural soil for respective crop production typically followed a loglogistic type distribution. Their statistic information including the mean, 5th, and 95th percentile values were shown in Table 5. In both spatial scopes, the AgNPs concentrations in sludge treated soil for wheat production are much greater than in other crop growing soil that have AgNPs sourced from irrigation. There is no manual irrigation used for wheat production in Ireland (Table 1), as a result the silver concentration in wheat growing soil (C_w) in Ireland comes totally from potential biosolid application. While in the EU, the surface water irrigation has a minor contribution to the C_w values in 2020 and 2030 (7.0 × 10−4 μg per kg(soil)) compared to the contribution of biosolid application (0.26 μg per kg(soil) in 2020, 0.57 μg per kg(soil) in 2030). These results are in line with previous studies which show that biosolid application is a major pathway for AgNPs build up in agricultural soil.12,73 The output AgNP concentration in sludge treated soil in Europe predicted for 2030 is in the range of the PEC of AgNPs in sludge treated soil in 2030 (0.027–2.123 μg per kg(soil)) reported by a previous estimation.74
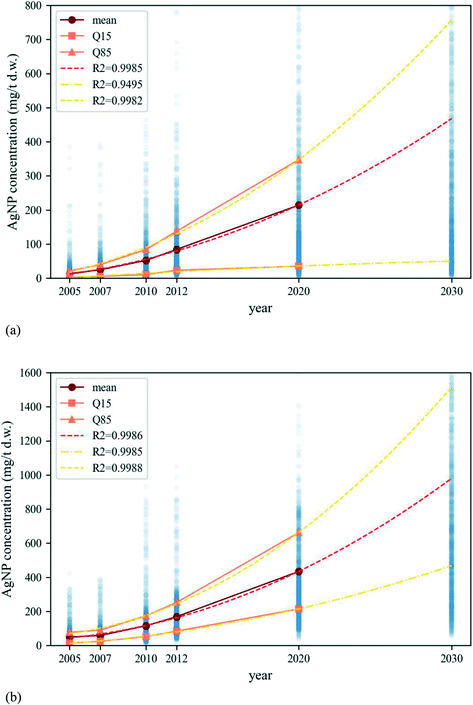 |
| Fig. 4 Quadratic curves of mean, Q15, and Q85 levels of AgNP concentrations in (a) European and (b) Irish biosolids, fitted by annual AgNP concentrations in reference years and predicted for annual level in 2030 (dots represent simulated results). | |
Table 5 AgNP concentrations in soil of leafy vegetables production (C_lv_s), root vegetables production (C_rv_s), and wheat production in 2020 and 2030 (C_w) in IE and EU scopes shown as mode (most frequent), 5th (5% percentile), and 5th (95% percentile) values (unit: μg per kg(soil))
Spatial scope |
Symbol |
5th |
Mean |
95th |
IE |
C_lv_s |
0.0006 |
0.0035 |
0.008 |
C_rv_s |
0 |
0 |
0 |
C_w (2020) |
0.006 |
0.12 |
0.38 |
C_w (2030) |
0.010 |
0.28 |
0.97 |
EU |
C_lv_s |
2.1 × 10−5 |
0.02 |
0.04 |
C_rv_s |
2.3 × 10−5 |
0.02 |
0.04 |
C_w (2020) |
0.008 |
0.3 |
1.13 |
C_w (2030) |
0.014 |
0.67 |
2.63 |
3.2 Crop-specific silver intakes
The mean levels of total silver intake (I_sum, eqn (19), Table 1) through daily crop ingestion are summarized in Table 6. It shows that the potential final silver intake by adults in the two spatial scopes and two reference years did not exceed the RfDs. The current and perspective intake levels in the Irish scope are predicted to be below those in the European scope, which is due to the fact that irrigation is rare in Ireland and has a very limited contribution on the AgNP buildup in agricultural soil compared to the European average. This regional difference implies the regional irrigation regimes under different climate conditions can result in variations of AgNP distributions in agricultural fields. Within the European scope, the predicted future increase of AgNP concentrations in sewage sludge will have a negligible influence on total silver intake. This is mainly because biosolids have been forbidden for use on growing vegetables in the EU,30 the only potential receiver among the selected crops is wheat, which has an extremely low BF of AgNPs from roots to grains (Table 1).64 The stringent biosolid application directed by the Directive 86/278/EEC effectively limits potential AgNPs uptake contributed by biosolids in European agricultural soil, as illustrated in Table 5, and hence limits potential dietary exposure. On the basis of the principle of sewage sludge application in the EU, Ireland reduced the maximum allowable application of sewage sludge on arable land6,48 which limits the impact of higher AgNP concentrations in biosolids compared to the European average (Table 1), and therefore resulted in the very low total silver intake.
Table 6 Total silver intake levels (mean, 5th, 95th percentile) through total daily crop consumption (unit: μg kg−1 bw d)
Year |
IE (5th, 95th) |
EU (5th, 95th) |
2020 |
6.48 × 10−07 (4.60 × 10−08, 2.04 × 10−06) |
1.43 × 10−04 (3.21 × 10−07, 2.73 × 10−04) |
2030 |
1.19 × 10−06 (6.66 × 10−08, 4.06 × 10−06) |
1.44 × 10−04 (3.93 × 10−07, 2.76 × 10−04) |
Fig. 5 demonstrates the contribution of crop-specific consumption to the current and perspective total silver intake resulting from AgNP emissions in Ireland and Europe. With the projected increasing AgNP concentration in biosolids over time, the contribution of daily wheat product ingestion to total silver intake will be even more significant compared to the ingestion of leafy vegetables. However, when extending to the European scope, leafy vegetable consumption potentially represents the highest contribution to silver intake resulting from AgNP release. There were no observable contributions from the three root vegetable categories. In Ireland, this is consistent with negligible AgNP concentrations in their growing soil (Table 5). While in Europe, though the irrigated soil for root and tuber vegetables receives a proportion of AgNPs (Table 5), the relative low BF for the majority of root and tuber vegetables (BF_rv1_s, BF_rv2_s, Table 1) and small daily intakes (Q_rv1, Q_rv2. Q_rv3, Table 1) result in limited silver exposure. Moreover, the dominating contribution of leafy vegetable consumption is also due to the additional route for leafy vegetables to be exposed to AgNPs through the use of sprinkler irrigation. In previous studies, sprinkler irrigation was a cause for concern when growing leafy vegetables due to its potential to contribute waterborne contaminations.46,53 Silver bioaccumulation has been observed following exposure of vegetable foliage to AgNP, with comparable AgNP bioavailability relative to root exposure.19,20,75 The importance of sprinkler irrigation highlights the need for investigation into AgNP bioaccumulation through foliage on other crop categories.
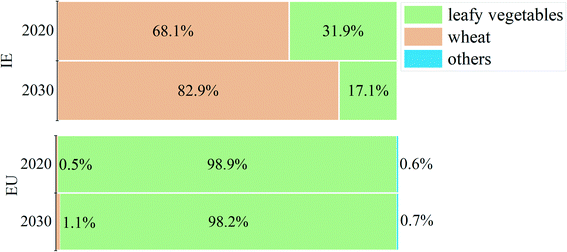 |
| Fig. 5 Contributions of crop intake to the total silver intake in the Irish and European scope in year 2020 and 2030. | |
3.3 Hazard quotients
The mean simulated probabilistic distributions for HQs are given in Table 7. The highest HQ occurred in the European scope (4.47 × 10−04) while there is no observable difference of HQ results between two reference years. The HQs followed a lognormal type distribution for all eight simulations. The probabilistic distribution shows the 99th percentile estimates of the highest HQ is far below the lowest threshold (HQ = 0.1) (HQ ≤ 0.1, no hazard exists; HQ = 0.1–1.0 low hazard; HQ = 1.1–10, moderate hazard; HQ ≥ 10, high hazard),76,77 which indicates no potential health hazard posed by AgNPs through crop consumption under the scenarios considered. Taking Irish cases as examples, Fig. 6 illustrates the four probability density distributions for HQs by using data in two reference years and two RfDs. The move of two distributions of HQs in 2020 to the right-hand side indicates the increase of hazard potential over time. However, compared to the effect of time, the application of different RfDs has a much greater influence on the HQ probability density distributions. This comparison suggests that under the present hazard potential level and in the next decade, the knowledge gap on the in vivo toxicity mechanism of silver introduced by AgNPs following dietary exposure represents the greatest uncertainty. Currently, the characterization studies on sulfurized AgNPs are still at the stage of morphology identification.11 The future hazard characterization on transformed AgNPs observed in natural environmental compartments would help improve the accuracy of risk assessment estimates. In addition, the morphology and composition of bioaccumulated silver in crops need further investigation.
Table 7 Mean hazard quotients including uncertainty analysis (5th, 95th percentile) based on two RfDs
Scenario |
HQ RfD1 (5th, 95th) |
HQ RfD2 (5th, 95th) |
IE 2020 |
4.63 × 10−08 (3.29 × 10−09, 1.46 × 10−07) |
3.24 × 10−07 (2.30 × 10−08, 1.02 × 10−06) |
IE 2030 |
8.50 × 10−08 (4.76 × 10−09, 2.90 × 10−07) |
5.95 × 10−07 (3.33 × 10−08, 2.03 × 10−06) |
EU 2020 |
1.23 × 10−05 (2.30 × 10−08, 1.95 × 10−05) |
7.16 × 10−05 (1.61 × 10−07, 1.36 × 10−04) |
EU 2030 |
1.03 × 10−05 (2.80 × 10−08, 1.97 × 10−05) |
7.22 × 10−05 (1.96 × 10−07, 1.38 × 10−04) |
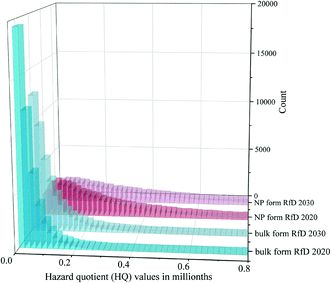 |
| Fig. 6 Probability distributions of HQs based on Irish AgNP releases and agricultural conditions in 2020 and 2030 using two RfDs. | |
3.4 Sensitivity analysis
Results of the sensitivity analysis are shown in Fig. 7, the influential variables on simulated HQs vary in the two spatial scopes. In the European scope (Fig. 7a), AgNP concentration in surface water (C_sw) is the most significant factor influencing the final health risk with a positive correlation coefficient of 0.68 (2020) and 0.63 (2030). In addition, the irrigation management parameters including sprinkler percentage (Ir_sp) and surface water irrigation percentage (Ir_sw) are also important factors. Comparatively, sludge related parameters, including AgNP content in sludge (M_sl) and the annual sludge application rate (Q_sl), show less importance to the final risk in 2020. This is due to the fact that sludge is not applied to certain food crops, hence the only source of AgNPs would be from irrigation water. With the AgNP concentration in sludge increases with time, the annual sludge application rate (Q_sl) becames more important as the correlation coefficient increased from 0.16 to 0.2. Consistent with the crop-specific silver intake illustration (Fig. 5), leafy vegetables are the only crop category whose daily intake (Q_lv) shows a positive correlation to the hazard quotient. Its coefficient (0.15) showed less of an impact than parameters in relation to irrigation management. These observations indicate the importance of irrigation management during crop production on AgNP risk control, which is more significant than the management of sludge treatment. In Europe, the upgrading of irrigation equipment by introducing more sprinkler and drip irrigation systems in order to increase irrigation efficiency will be an important element of the future crop production78 which may lead to higher potential AgNP contamination in the future. This is consistent with previous risk assessments for other waterborne contaminants indicating potential concentrations of contaminants in surface water and sprinkler irrigation usage are the most important parameters and positively correlated with potential human exposure.46
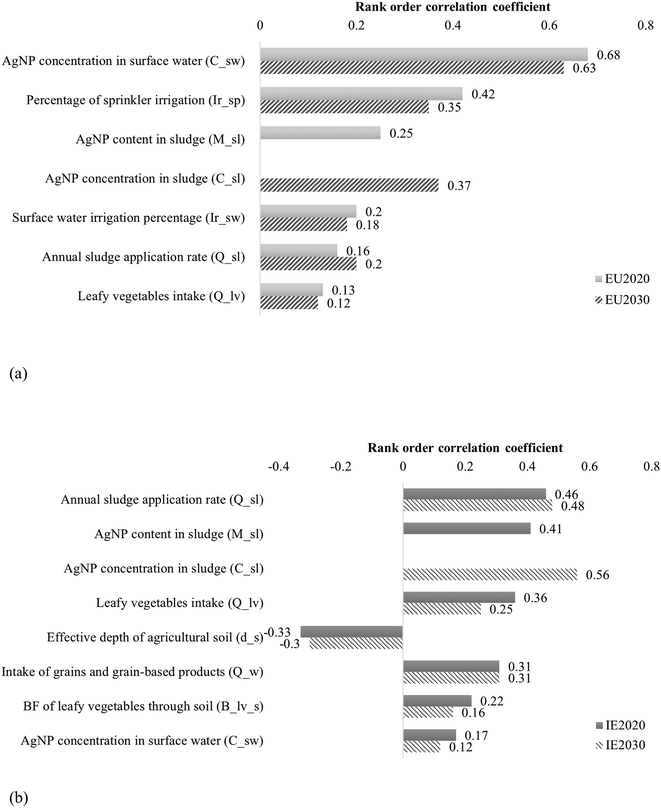 |
| Fig. 7 Sensitivity analysis of HQs in (a) in European scope and (b) Irish scope comparing 2020 and 2030 levels. | |
The sensitivity analysis results for HQs in the Irish scope indicate that in regions with sufficient rainfall, the AgNP content in sludge and the sludge application rate (M_sl, Q_sl, respectively) have significant effects on the final risk potential (correlation coefficients: 0.41, 0.46, respectively). Therefore, the increase of AgNP concentration in sewage sludge in 2030 (C_sl, 0.56) has shown a significant impact on the final risk. As the AgNP concentration in sludge increases, the sludge treatment parameter namely sludge application rate (Q_sl) has a greater effect on the final HQ. Correspondingly, the AgNPs from surface water (C_sw) used for irrigating leafy vegetables has less of an effect. The intake of grain based products (Q_w) became more influential than intake of leafy vegetables (Q_lv) and relative bioaccumulation factor (B_lv_s). The positive correlation for leafy vegetable bioaccumulation through root exposure (B_lv_s) is due to the extremely low HQs in Irish scenarios, when the HQ increased to values such as those seem in EU situation, the contribution of the bioaccumulation factors would not be as important. The only negative correlation value was for the effective depth of soil (d_s) highlighting the impact of soil properties on the contamination level of AgNPs in crops. The deeper transport into the soil can reduce the concentration in soil and the potential uptake by the plant. Vertical mobility studies on AgNPs show rather high transport potential compared to other ENPs but this varied in different soil columns.79 This highlights the importance of behavioral studies of AgNPs, especially in agricultural soil, in influencing final risk levels. Overall, a reduction in both sludge application and the AgNP content in sewage sludge would provide the largest decrease of potential health risk resulting from AgNPs emissions in areas with sufficient rainfall for crop production.
4. Conclusion
This study is among the first to establish a probabilistic risk assessment framework for assessing the human health risk posed by the potential release of AgNPs in agricultural crop production, which considered both the surface water irrigation and biosolid application as sources of AgNPs. According to simulated results of HQs under the scenarios considered, daily consumption of leafy vegetables, root and tuber vegetables, and wheat products poses very little threat to human health resulting from the environmental release of AgNPs. Regional parameters in Ireland and Europe and crop-specific variables among three crop categories were analyzed for their influence on the final risk. For the scenarios considered, the prediction of AgNP concentrations in biosolids indicates the increase of AgNP concentrations in biosolids in the next decade has very limited impact on the final risk potential.
At the EU average, AgNPs from irrigation is the dominant contribution to human total silver intake. Adjusting from sprinkler irrigation to drip or surface irrigation is identified as effective approach to reduce further the potential risk. In regions with sufficient rainfall, such as in Ireland, the silver intake by consumers through crop ingestion is most sensitive to the AgNP contents in biosolids and the application rate of biosolids. Currently, the control of biosolid application on growing vegetables and the limited maximum application rate can help to effectively prevent potential AgNP contamination. This study highlights the potential contribution of agriculture-related AgNP emissions to human health risk potential. Findings provide an in depth understanding of the influences of agricultural schemes, regional variables, and AgNP behavior in the crop production process on the final human health risk.
To reduce the uncertainty around the present risk quantification, more research is needed in terms of the bioaccumulation processes of AgNPs with diverse physicochemical properties. It is important to carry out the hazard characterization based on the morphology identification of bioaccumulated AgNPs. Moreover, the onsite detection and characterization of AgNPs, or regional and temporal predictions of various AgNP releases entering the crop production process could also help contribute to a more precise assessment.
Conflicts of interest
There are no conflicts to declare.
Acknowledgements
The authors would like to acknowledge the China Scholarship Council (CSC) and University College Dublin (UCD) for supporting this study under CSC-UCD Scheme.
Notes and references
- A. P. K. Jantunen, S. Gottardo, K. Rasmussen and H. P. Crutzen, An inventory of ready-to-use and publicly available tools for the safety assessment of nanomaterials, NanoImpact, 2018, 12, 18–28 CrossRef PubMed.
- J. B. Guinée, R. Heijungs, M. G. Vijver and W. J. G. M. Peijnenburg, Setting the stage for debating the roles of risk assessment and life-cycle assessment of engineered nanomaterials, Nat. Nanotechnol., 2017, 12, 727–733 CrossRef PubMed.
- Y. Li and E. Cummins, Hazard characterization of silver nanoparticles for human exposure routes, J. Environ. Sci. Health A Tox. Hazard. Subst. Environ. Eng., 2020, 55, 704–725 CrossRef CAS PubMed.
- A. Zeb, W. Liu, J. Wu, J. Lian and Y. Lian, Knowledge domain and emerging trends in nanoparticles and plants interaction research: A scientometric analysis, NanoImpact, 2021, 21, 100278 CrossRef CAS PubMed.
- J. L. Gardea-Torresdey, C. M. Rico and J. C. White, Trophic Transfer, Transformation, and Impact of Engineered Nanomaterials in Terrestrial Environments, Environ. Sci. Technol., 2014, 48, 2526–2540 CrossRef CAS PubMed.
- M. C. Collivignarelli, A. Abbà, A. Frattarola, M. C. Miino, S. Padovani, I. Katsoyiannis and V. Torretta, Legislation for the Reuse of Biosolids on Agricultural Land in Europe: Overview, Sustainability, 2019, 11, 1–22 CrossRef.
- F. Gottschalk, R. W. Scholz and B. Nowack, Probabilistic material flow modeling for assessing the environmental exposure to compounds: Methodology and an application to engineered nano-TiO2 particles, Environ. Model. Softw., 2010, 25, 320–332 CrossRef.
- T. Y. Sun, N. A. Bornhöft, K. Hungerbühler and B. Nowack, Dynamic Probabilistic Modeling of Environmental Emissions of Engineered Nanomaterials, Environ. Sci. Technol., 2016, 50, 4701–4711 CrossRef CAS PubMed.
- N. Stoudmann, B. Nowack and C. Som, Prospective environmental risk assessment of nanocellulose for Europe, Environ. Sci.: Nano, 2019, 6, 2520–2531 RSC.
- J. Kuenen, V. Pomar-Portillo, A. Vilchez, A. Visschedijk, H. Denier Van Der Gon, S. Vázquez-Campos, B. Nowack and V. Adam, Inventory of country-specific emissions of engineered nanomaterials throughout the life cycle, Environ. Sci.: Nano, 2020, 7, 3824–3839 RSC.
- G. Metreveli, J. David, R. Schneider, S. Kurtz and G. E. Schaumann, Morphology, structure, and composition of sulfidized silver nanoparticles and their aggregation dynamics in river water, Sci. Total Environ., 2020, 739, 139989 CrossRef CAS PubMed.
- A. E. Pradas del Real, H. Castillo-Michel, R. Kaegi, B. Sinnet, V. Magnin, N. Findling, J. Villanova, M. Carrière, C. Santaella, A. Fernández-Martínez, C. Levard and G. Sarret, Fate of Ag-NPs in Sewage Sludge after Application on Agricultural Soils, Environ. Sci. Technol., 2016, 50, 1759–1768 CrossRef CAS PubMed.
- M. Li, B. K. Greenfield, L. M. Nunes, F. Dang, H. L. Liu, D. M. Zhou and B. Yin, High retention of silver sulfide nanoparticles in natural soils, J. Hazard. Mater., 2019, 378, 120735 CrossRef CAS PubMed.
- V. Bolaños-Benítez, F. McDermott, L. Gill and J. Knappe, Engineered silver nanoparticle (Ag-NP) behaviour in domestic on-site wastewater treatment plants and in sewage sludge amended-soils, Sci. Total Environ., 2020, 722, 137794 CrossRef PubMed.
- F. Gottschalk, T. Sonderer, R. W. Scholz and B. Nowack, Modeled environmental concentrations of engineered nanomaterials (TiO2, ZnO, Ag, CNT, fullerenes) for different regions, Environ. Sci. Technol., 2009, 43, 9216–9222 CrossRef CAS PubMed.
- V. Adam, Q. Wu and B. Nowack, Integrated dynamic probabilistic material flow analysis of engineered materials in all European countries, NanoImpact, 2021, 22, 100312 CrossRef CAS PubMed.
- J. Il Kwak, R. Cui, S. H. Nam, S. W. Kim, Y. Chae and Y. J. An, Multispecies toxicity test for silver nanoparticles to derive hazardous concentration based on species sensitivity distribution for the protection of aquatic ecosystems, Nanotoxicology, 2016, 10, 521–530 CrossRef PubMed.
-
FAO, Irrigation in Eastern Europe in figures – AQUASTAT Survey 2016, Irrigation in Eastern Europe in figures – AQUASTAT Survey 2016, https://www.fao.org/3/CA3380EN/ca3380en.pdf, (accessed 20 January 2021).
- J. Wu, G. Wang, M. G. Vijver, T. Bosker and W. J. G. M. Peijnenburg, Foliar versus root exposure of AgNPs to lettuce: Phytotoxicity, antioxidant responses and internal translocation, Environ. Pollut., 2020, 261, 114117 CrossRef CAS PubMed.
- M. Li, H. L. Liu, F. Dang, H. Hintelmann, B. Yin and D. Zhou, Alteration of Crop Yield and Quality of Three Vegetables upon Exposure to Silver Nanoparticles in Sludge-Amended Soil, ACS Sustainable Chem. Eng., 2020, 8, 2472–2480 CrossRef CAS.
- F. Dang, Y. Z. Chen, Y. N. Huang, H. Hintelmann, Y. Bin Si and D. M. Zhou, Discerning the Sources of Silver Nanoparticle in a Terrestrial Food Chain by Stable Isotope Tracer Technique, Environ. Sci. Technol., 2019, 53, 3802–3810 CrossRef CAS PubMed.
- A. Yan and Z. Chen, Impacts of silver nanoparticles on plants: A focus on the phytotoxicity and underlying mechanism, Int. J. Mol. Sci., 2019, 20 Search PubMed.
- V. Adam and B. Nowack, European country-specific probabilistic assessment of nanomaterial flows towards landfilling, incineration and recycling, Environ. Sci.: Nano, 2017, 4, 1961–1973 RSC.
- G. Montes de Oca-Vásquez, F. Solano-Campos, J. R. Vega-Baudrit, R. López-Mondéjar, I. Odriozola, A. Vera, J. L. Moreno and F. Bastida, Environmentally relevant concentrations of silver nanoparticles diminish soil microbial biomass but do not alter enzyme activities or microbial diversity, J. Hazard. Mater., 2020, 391, 122224 CrossRef PubMed.
- Y. Li and E. Cummins, Hazard characterization of silver nanoparticles for human exposure routes, J. Environ. Sci. Health A Tox. Hazard. Subst. Environ. Eng., 2020, 55, 704–725 CrossRef CAS PubMed.
- S. Rajkovic, N. A. Bornhöft, R. van der Weijden, B. Nowack and V. Adam, Dynamic probabilistic material flow analysis of engineered nanomaterials in European waste treatment systems, Waste Manage., 2020, 113, 118–131 CrossRef CAS PubMed.
- Y. Wang and B. Nowack, Dynamic probabilistic material flow analysis of nano-SiO2, nano iron oxides, nano-CeO2, nano-Al2O3, and quantum dots in seven European regions, Environ. Pollut., 2018, 235, 589–601 CrossRef CAS PubMed.
-
FAO, Food Balance Sheets - A Handbook, Rome, 2001 Search PubMed.
-
EFSA, Food composition data|European Food Safety Authority, Food composition data|European Food Safety Authority, https://www.efsa.europa.eu/en/microstrategy/food-composition-data, (accessed 4 August 2021).
-
EC, EUR-Lex Council Directive 86/278/EEC of 12 June 1986 on the Protection of the Environment, and in Particular of the Soil, when Sewage Sludge is Used in Agriculture, 1986.
-
FAO, Area harvested (1000 ha)- FAOSTAT, Area harvested (1000 ha)- FAOSTAT, https://www.fao.org/faostat/en/#data/QC, (accessed 16 March 2021).
- N. O'Brien and E. Cummins, Nano-Scale Pollutants: Fate in Irish Surface and Drinking Water Regulatory Systems, Hum. Ecol. Risk Assess., 2010, 16, 847–872 CrossRef.
- J. Zhao, M. Lin, Z. Wang, X. Cao and B. Xing, Engineered nanomaterials in the environment: Are they safe?, Crit. Rev. Environ. Sci. Technol., 2020, 1–36 Search PubMed.
-
FAO, % of area equipped for irrigation by surface water- AQUASTAT database, % of area equipped for irrigation by surface water- AQUASTAT database, https://www.fao.org/aquastat/statistics/query/index.html?lang=en, (accessed 12 March 2021).
-
FAO, total agricultural water managed area (1000ha)- AQUASTAT database, total agricultural water managed area (1000ha)- AQUASTAT database, https://www.fao.org/aquastat/statistics/query/index.html?lang=en, (accessed 12 March 2021).
-
FAO, Area equipped for full control irrigation: sprinkler irrigation (1000 ha)- AQUASTAT database, Area equipped for full control irrigation: sprinkler irrigation (1000 ha)- AQUASTAT database, https://www.fao.org/aquastat/statistics/query/index.html?lang=en, (accessed 1 March 2021).
-
M. M. Mekonnen and A. Y. Hoekstra, The Green, Blue and Grey Water Footprint of Crops and Derived Crop Products, 2010 Search PubMed.
-
FAO, Harvested irrigated
area (1000 ha)- AQUASTAT database, Harvested irrigated area (1000 ha)- AQUASTAT database, https://www.fao.org/aquastat/statistics/query/index.html?lang=en, (accessed 16 March 2021).
-
FAO, FAOSTAT_Crop statistics, FAOSTAT_Crop statistics, https://www.fao.org/faostat/en/#data/QC, (accessed 8 June 2021).
- E. Hosseini Koupaie and C. Eskicioglu, Health risk assessment of heavy metals through the consumption of food crops fertilized by biosolids: A probabilistic-based analysis, J. Hazard. Mater., 2015, 300, 855–865 CrossRef CAS PubMed.
-
G. D. Betrie, R. Sadiq, K. A. Morin and S. Tesfamariam, Uncertainty Analysis of an Aquivalence-based Fate and Transport Model using a Hybrid Fuzzy-Probabilistic Approach, 12th Int. Environ. Spec. Conf., 2012, ENV1013, ENV10131–ENV10139 Search PubMed.
- T. Y. Sun, F. Gottschalk, K. Hungerbühler and B. Nowack, Comprehensive probabilistic modelling of environmental emissions of engineered nanomaterials, Environ. Pollut., 2014, 185, 69–76 CrossRef CAS PubMed.
- E. Bolea, M. S. Jimenez, J. Perez-Arantegui, J. C. Vidal, M. Bakir, K. Ben-Jeddou, A. C. Gimenez-Ingalaturre, D. Ojeda, C. Trujillo and F. Laborda, Analytical applications of single particle inductively coupled plasma mass spectrometry: A comprehensive and critical review, Anal. Methods, 2021, 13, 2742–2795 RSC.
- D. Mozhayeva and C. Engelhard, A critical review of single particle inductively coupled plasma mass spectrometry-A step towards an ideal method for nanomaterial characterization, J. Anal. At. Spectrom., 2020, 35, 1740–1783 RSC.
- G. Wriedt, M. van der Velde, A. Aloe and F. Bouraoui, A European irrigation map for spatially distributed agricultural modelling, Agric. Water Manag., 2009, 96, 771–789 CrossRef.
- E. O'Flaherty, A. G. Solimini, F. Pantanella, M. De Giusti and E. Cummins, Human exposure to antibiotic resistant-Escherichia coli through irrigated lettuce, Environ. Int., 2019, 122, 270–280 CrossRef PubMed.
- N. A. Bornhöft, T. Y. Sun, L. M. Hilty and B. Nowack, A dynamic probabilistic material flow modeling method, Environ. Model. Softw., 2016, 76, 69–80 CrossRef.
- A. Kelessidis and A. S. Stasinakis, Comparative study of the methods used for treatment and final disposal of sewage sludge in European countries, Waste Manage., 2012, 32, 1186–1195 CrossRef CAS PubMed.
-
Eurostat, Sewage sludge production and disposal, Sewage sludge production and disposal, https://ec.europa.eu/eurostat/databrowser/view/env_ww_spd/default/map?lang=en, (accessed 1 March 2021).
-
R. Milieu Ltd and WRc, Environmental, economic and social impacts of the use of sewage sludge on land Final Report Part III: Project Interim Reports RPA, 2010 Search PubMed.
-
European Commission, Disposal and Recycling Routes for Sewage Sludge Part 2—Regulatory Report, 2001 Search PubMed.
-
European Commission, Disposal and Recycling Routes for Sewage Sludge Part 1—Sludge Use Acceptance Report, 2001 Search PubMed.
- H.-F. Mok and A. J. Hamilton, Exposure Factors for Wastewater-Irrigated Asian Vegetables and a Probabilistic Rotavirus Disease Burden Model for Their Consumption, Risk Anal., 2014, 34, 602–613 CrossRef PubMed.
- M. Gallardo, L. E. Jackson, K. Schulbach, R. L. Snyder, R. B. Thompson and L. J. Wyland, Production and water use in lettuces under variable water supply, Irrig. Sci., 1996, 16, 125–137 CrossRef.
- W. Du, Y. Sun, R. Ji, J. Zhu, J. Wu and H. Guo, TiO2 and ZnO nanoparticles negatively affect wheat growth and soil enzyme activities in agricultural soil, J. Environ. Monit., 2011, 13, 822 RSC.
- F. Liu, B. Xu, Y. He, P. C. Brookes, C. Tang and J. Xu, Differences in transport behavior of natural soil colloids of contrasting sizes from nanometer to micron and the environmental implications, Sci. Total Environ., 2018, 634, 802–810 CrossRef CAS PubMed.
- S. E. Taylor, C. I. Pearce, I. Chowdhury, L. Kovarik, I. Leavy, S. Baum, A. I. Bary and M. Flury, Long-term accumulation, depth distribution, and speciation of silver nanoparticles in biosolids-amended soils, J. Environ. Qual., 2020, 49, 1679–1689 CrossRef CAS PubMed.
- A. Taghavy and L. M. Abriola, Modeling reactive transport of polydisperse nanoparticles: assessment of the representative particle approach, Environ. Sci.: Nano, 2018, 5, 2293–2303 RSC.
- W. D. Reynolds, B. T. Bowman, C. F. Drury, C. S. Tan and X. Lu, Indicators of good soil physical quality: density and storage parameters, Geoderma, 2002, 110, 131–146 CrossRef CAS.
- P. Wang, N. W. Menzies, P. G. Dennis, J. Guo, C. Forstner, R. Sekine, E. Lombi, P. Kappen, P. M. Bertsch and P. M. Kopittke, Silver Nanoparticles Entering Soils via the Wastewater-Sludge-Soil Pathway Pose Low Risk to Plants but Elevated Cl Concentrations Increase Ag Bioavailability, Environ. Sci. Technol., 2016, 50, 8274–8281 CrossRef CAS PubMed.
- K. Vishwakarma, Shweta, N. Upadhyay, J. Singh, S. Liu, V. P. Singh, S. M. Prasad, D. K. Chauhan, D. K. Tripathi and S. Sharma, Differential phytotoxic impact of plant mediated silver nanoparticles (AgNPs) and silver nitrate (AgNO3) on Brassica sp., Front. Plant Sci., 2017, 8, 1501 CrossRef PubMed.
- N. Saleeb, R. Gooneratne, J. Cavanagh, C. Bunt, A. K. M. M. Hossain, S. Gaw and B. Robinson, The Mobility of Silver Nanoparticles and Silver Ions in the Soil-Plant System, J. Environ. Qual., 2019, 48, 1835–1841 CrossRef CAS.
- N. Zuverza-Mena, R. Armendariz, J. R. Peralta-Videa and J. L. Gardea-Torresdey, Effects of Silver Nanoparticles on Radish Sprouts: Root Growth Reduction and Modifications in the Nutritional Value, Front. Plant Sci., 2016, 7, 90 Search PubMed.
- J. Yang, F. Jiang, C. Ma, Y. Rui, M. Rui, M. Adeel, W. Cao and B. Xing, Alteration of Crop Yield and Quality of Wheat upon Exposure to Silver Nanoparticles in a Life Cycle Study, J. Agric. Food Chem., 2018, 66, 2589–2597 CrossRef CAS PubMed.
-
USEPA, Exposure factors handbook, 2018 Search PubMed.
- EFSA, Use of the EFSA Comprehensive European Food Consumption Database in Exposure Assessment, EFSA J., 2011, 9(3), 2097 Search PubMed.
- EFSA, The food classification and description system FoodEx 2 (revision 2), EFSA Support. Publ., 2015, EN-804, 90 Search PubMed.
- F. Dang, Q. Wang, W. Cai, D. Zhou and B. Xing, Uptake kinetics of silver nanoparticles by plant: relative importance of particles and dissolved ions, Nanotoxicology, 2020, 14, 654–666 CrossRef CAS PubMed.
- J. He, Y. Li, H. Qi, H. Li and W. Zhang, Biochar amendment changed soil-bound fractions of silver nanoparticles and ions but not their uptake by radish at an environmentally-relevant concentration, BioChar, 2020, 2, 307–317 CrossRef.
- R. D. Kent, J. G. Oser and P. J. Vikesland, Controlled evaluation of silver nanoparticle sulfidation in a full-scale wastewater treatment plant, Environ. Sci. Technol., 2014, 48, 8564–8572 CrossRef CAS PubMed.
-
U.S. EPA, Silver (CASRN 7440-22-4)|IRIS|US EPA, 1991 Search PubMed.
- F. T. Mathias, R. M. Romano, M. M. L. Kizys, T. Kasamatsu, G. Giannocco, M. I. Chiamolera, M. R. Dias-da-Silva and M. A. Romano, Daily exposure to silver nanoparticles during prepubertal development decreases adult sperm and reproductive parameters, Nanotoxicology, 2015, 9, 64–70 CrossRef CAS PubMed.
- K. Schlich, T. Klawonn, K. Terytze and K. Hund-Rinke, Hazard assessment of a silver nanoparticle in soil applied via sewage sludge, Environ. Sci. Eur., 2013, 25, 17 CrossRef.
- B. Giese, F. Klaessig, B. Park, R. Kaegi, M. Steinfeldt, H. Wigger, A. von Gleich and F. Gottschalk, Risks, Release and Concentrations of Engineered Nanomaterial in the Environment, Sci. Rep., 2018, 8, 1565 CrossRef PubMed.
- C. C. Li, F. Dang, M. Li, M. Zhu, H. Zhong, H. Hintelmann and D. M. Zhou, Effects of exposure pathways on the accumulation and phytotoxicity of silver nanoparticles in soybean and rice, Nanotoxicology, 2017, 11, 699–709 CrossRef CAS PubMed.
-
A. D. Lemly, Evaluation of the Hazard Quotient Method for Risk Assessment of Selenium, 1996 Search PubMed.
- R. Clarke, D. Peyton, M. G. Healy, O. Fenton and E. Cummins, A quantitative risk assessment for metals in surface water following the application of biosolids to grassland, Sci. Total Environ., 2016, 566–567, 102–112 CrossRef CAS PubMed.
-
EEA, Water intensity of crop production in Europe — European Environment Agency, Water intensity of crop production in Europe — European Environment Agency, https://www.eea.europa.eu/data-and-maps/indicators/economic-water-productivity-of-irrigated-2/assessment, (accessed 31 May 2021).
- Y. Li and E. Cummins, A semi-quantitative risk ranking of potential human exposure to engineered nanoparticles (ENPs) in Europe, Sci. Total Environ., 2021, 778, 146232 CrossRef CAS PubMed.
|
This journal is © The Royal Society of Chemistry 2022 |