Bioreactivity of a novel poly(epsilon-caprolactone) nanocapsule containing atrazine with human lung alveolar epithelial cells†
Received
21st November 2021
, Accepted 20th April 2022
First published on 28th April 2022
Abstract
Atrazine (ATZ), a commonly used agricultural herbicide, is potentially harmful to animals and humans. Nanoencapsulation of ATZ (NC–ATZ) within non-toxic, biodegradable poly(ε-caprolactone) (PCL) improves the herbicidal activity of ATZ 10-fold and moderates its environmental persistence, possibly reducing off-target ecological effects. These compounds may reach the pulmonary respiratory units following inhalation; thus, we investigated the effect of ATZ and NC–ATZ on an immortalised human lung alveolar type 1-like epithelial cell model (TT1 cells). The concentration-dependent effect of the compounds was analysed by assessment of viability, reactive oxygen species (ROS) production and inflammatory cytokine release. Confocal microscopy was used to visualise TT1 cell-nanoparticle interactions. NC–ATZ caused cellular effects not observed with ATZ or the PCL nanocapsule alone. NC–ATZ significantly increased lactate dehydrogenase (LDH) release at ≥1 μg ml−1 after 48 hours of exposure, peaking at 5 μg ml−1 (3-fold of the non-treated control, ***p < 0.001). Moreover, ≥1 μg ml−1 NC–ATZ was pro-inflammatory at 48 hours, peaking at 5 μg ml−1 (IL-6 release ∼125 pg ml−1; IL-8 release ∼46 pg ml−1). Confocal microscopy of fluorescently-labelled ATZ and NC–ATZ indicated high intensity fluorescence nanoparticle uptake into the cytoplasm and co-localisation in the Golgi, suggesting nanoparticle recycling within 24 hours. We provide evidence that nanoencapsulation of the pesticide ATZ alters bioreactivity, stimulating more necrosis and inflammation in human lung alveolar type 1 epithelial cells in comparison to ATZ or the PCL nanocapsule alone. However, nanoencapsulation improves the efficacy of pesticides, thus lower pesticide concentrations could be used, reducing environmental contamination. Further investigation, particularly with different exposure scenarios, is warranted in order to generate safer nanoencapsulated pesticides.
Environmental significance
The environment is being inadvertently damaged due to increasing food demands requiring pesticide use. Repeated, high quantities of pesticide use may result in pesticide resistance, reduced soil biodiversity and death of beneficial, non-target organisms. Nanoencapsulation of pesticides can reduce contamination by controlling release and improving efficacy. However, it is important to ensure that there are no unwanted effects in humans and wildlife. Although previous research suggested poly(ε-caprolactone) nanoencapsulation of atrazine was safer to human cells, we discovered adverse bioreactivity with human lung alveolar type 1 epithelial cells compared to ATZ or the nanocapsule alone. While nanoencapsulation reduces environmental exposure to ATZ, further development of safe NC–ATZ formulations is required. This study highlights the importance of determining off-target behaviours of nanomaterials.
|
1. Introduction
Estimates suggest that by 2050 the global population will be 9.7 billion; thus, with the additional threats of climate change and conflicts leading to food scarcity, the importance of maximising agricultural productivity is vital.1,2 Pests and disease are responsible for 20–40% loss of agricultural production worldwide, yet greater damage has been prevented by use of pesticides.3 However, pesticides are associated with harm to both humans and the environment. Pimentel et al. suggested the combined cost of pesticides (purchase price and harm to health/livestock) is comparable to the economic benefits of improved crop production, hence there is both a moral and economic motive for safer and more cost-effective pesticides.4
Atrazine (ATZ) is a herbicide which controls broad-leaved weeds, mainly in the production of maize, sorghum and sugar cane.5 It is among the most commonly used herbicides worldwide.6 In the USA, a country responsible for 24% of global pesticide sales, ATZ is the second most highly applied herbicide (500
000 tonnes annually).7 As well as the herbicidal benefits, the relatively cheap price of ATZ ($14 per gallon) compared to other alternatives has made it a competitive product in the agricultural sector.8 In pre-emergence treatment, ATZ is absorbed by the roots and transported to the leaves via the xylem. Direct application to the leaves inhibits photosynthesis by displacement of plastoquinone from its D1 binding-site on photosystem II in chloroplasts. This disrupts the electron transport chain and subsequent generation of reactive oxygen species causes lipid and protein damage resulting ultimately in leaf chlorosis, necrosis and plant death.6,9 Non-target plants are unaffected by rapid outward transport or degradation of ATZ.10
Evidence concerning the effects of ATZ on animals and humans is ambiguous. Several in vivo rodent studies discovered aerosolised ATZ exposure induced oxidative and nitrosative stress, leading to inflammatory cytokine release, through the nuclear factor-erythroid 2-related factor (Nrf2) pathway; alterations of the Nrf2 pathway in mouse lung and brain results in increased lipid peroxidation, autophagy and apoptosis, leading ultimately to tissue damage, neurodegeneration and pulmonary fibrosis.11–13 Moreover, multiple studies have found ATZ to cause endocrine disruption in amphibians and rodents. A study in the United States (US) of male frogs (Xenopus laevis) reared in contaminated water found low levels of ATZ (1 × 10−4 μg ml−1) triggered gonadal dysgenesis and male hermaphrodism.14 In the same amphibian species, a Canadian group reported the frogs had incomplete testicular development and decreased testicular size and germ cell numbers after 48 hours in water with ATZ (0.021 μg ml−1).15 Changes in circular RNAs have also been implicated in multiple developmental anomalies in male tadpoles exposed to ATZ, with Wnt signalling and progesterone-mediated oocyte maturation pathways identified as differentially upregulated in testis tissue.16 Additionally, in rodents, it has been demonstrated that ATZ causes delayed reproductive development in both sexes. Recent studies have shown environmentally relevant doses of ATZ (0.1–10 mg kg−1 body weight, oral exposure) had an adverse effect on sperm motility and viability of adult Calomy laucha rodents as well as pubescent C57BL/6J male mice.17,18 Higher doses of ATZ (0–300 mg kg−1 body weight, oral exposure), representative of bioaccumulation, also resulted in reduced sperm dynamics in male albino rats, as well as lowered body weight gains, raised organ weights (except in the testes), and increased T3 and T4 hormones (thyroid function disruption).19 Data from randomised control trials in rats support evidence of ATZ as a toxicant to the male reproductive system and conclude it may have similar effects on the male human reproductive system.20 In female rodents, delayed female sexual maturation (timing of uterine growth and vaginal opening) has been demonstrated. Laws et al. showed this effect with daily oral gavages of diamino-s-chlorotriazine, a by-product of ATZ, at doses of 100 mg kg−1 and 200 mg kg−1, in peripubertal female Wistar rats.21 Ashby et al. showed a similar response through post-natal oral exposure to 100 mg kg−1 ATZ in female Sprague-Dawley rats.22 A number of mechanisms have been suggested to explain these diverse disruptive effects of ATZ including stimulation of aromatase and inhibition of androgen receptors.23 Although no clear evidence of endocrine disruption has been reported in humans, in vitro studies in human cell lines, including the MCF-7 breast cancer cell line and H295R adrenocortical cell line, do support the potential of ATZ to cause adverse effects via aromatase induction.24,25 Furthermore, a US-multi centred case-control study saw poorer sperm quality (reduced concentration and motility) in exposed men; however the association was confounded, as it often is, by other pesticide exposures.26 Moreover, an association between ATZ exposure and Parkinson's disease (PD), an idiopathic disease of the central nervous system, has been identified. Multiple epidemiological studies suggest a correlation of pesticide use and PD with a recent meta-analysis finding 5 and 10 years of pesticide exposure was associated with 5 and 11% increased risk respectively of PD.27 Biochemical studies implicate pesticide exposure with the inhibition and loss of dopaminergic neurones, mitochondrial impairment, and oxidative stress in the human brain.28 Atrazine has been shown to be directly toxic for dopaminergic neurones with some studies identifying miRNA deregulation (e.g. miRNA-7, −181 and −34, often studied within the context of PD).29 In an avian study, neurodegeneration from ATZ exposure has been linked to mitochondrial damage, malformation and apoptosis of neuronal cells.30
Similarly, confounders as well as a lack of sufficient evidence led to the controversial decision by the International Agency for Research on Cancer (IARC) to choose the category of “not classifiable as to its carcinogenicity to humans” (Group 3).31In vitro, ATZ has been found to promote proliferation, migration and invasion in epithelial ovarian cancer cells (Skov3 and A2780 cell lines) by induction of reactive oxygen species.32 In the common carp, 24 h in vitro exposure caused significant alteration of multiple miRNAs in many pathways, with expression of female-biased genes resulting from decreased miRNAs in the primordial gland; evidence also indicated ATZ can upregulate aromatase through miRNAs.33 Chronic feeding regimes of 22.5 mg per kg per day of ATZ caused early onset of mammary gland tumours in female Sprague-Dawley rats compared to untreated control rats.34 These results were not replicated in the Fischer 344 rat model due to differences in hormonal environment of Sprague Dawley rats and the mechanisms that control reproductive senescence and the development of the mammary tumours during aging.31,35–37 Thus elevated endogenous oestrogen levels were only observed in Sprague-Dawley rats;34 in this case, ATZ is likely to cause dysfunction of luteinising hormone cycling and premature reproductive senescence leading to increased susceptibility to mammary tumour development.31 Fischer 334 rats have higher circulatory progesterone levels which influences the effect of oestrogen on the hypothalamic–pituitary axis in the development of mammary tumours.35,36 Consequently, these mechanisms of carcinogenesis are not relevant to humans where reproductive ageing is related to primary follicle depletion.31,34 The Agricultural Health Study (AHS), a large human prospective cohort study in the US from 1993–2001, initially observed trends with ATZ exposure relating to increased risk of lung and bladder cancer, non-Hodgkin's lymphoma (NHL) and multiple myeloma.38 However later analysis (up to 2007; from the initial recruitment timeframe) of double the number of exposed cases saw no significant excess risk with greater duration or intensity of ATZ exposure.39 Despite a larger sample size, no clear conclusions could be made due to few cancer ‘events’ (for example 152 exposed cases with NHL compared to 68 cases in the initial study) and a lack of detailed pesticide exposure measurements.39 These are common limitations for epidemiological studies investigating pesticide exposure.40 A recent finding of the AHS, in a genome-wide DNA methylation study, found methylation of PAX6 with ATZ; this implicates ATZ exposure in the development of diabetes and abnormal eye development.41
The United States Geological Survey reported ten or more pesticide contaminants in approximately 20% of their tests and two or more in 90% of their tests in streams.42 Thus, flora and fauna are consistently exposed to multiple pesticides and, indeed, large quantities of ATZ are lost to the environment. ATZ is mobile so it can migrate into groundwater entering streams and rivers via runoff.43 In the US, ATZ is found in approximately 75% of streams and 40% of groundwater samples.42 18% of streams tested had atrazine concentrations which exceeded one or more aquatic benchmarks (including the acute and chronic ATZ concentrations above which unacceptable effects to an aquatic community occur).42 Furthermore ATZ is degraded slowly and can persist for 146 days in soil and 742 days in water.43 The most recent US Environmental Protection Agency (EPA) report, published in 2018, stated that drinking water wells in only Florida and Wisconsin exceeded the maximum contaminant levels (0.003 μg ml−1 as an annual average) for ATZ in groundwater.43 However seasonal variations in ATZ exposure, especially related to timing of precipitation and runoff events with ATZ application, can significantly increase the likelihood of periodic high contamination levels in other areas. A study of the White River in Indiana, USA in 1991–1992, demonstrated variation of 0.009 μg ml−1 (at the time of the first runoff events following high ATZ usage in May and June) to <0.001 μg ml−1 (between August to March when less pesticide was used).44
ATZ may also remain in the air following application or is volatilized from bodies of water. It can be carried long distances on particles of dust without being broken down, affecting wildlife and human populations far from the site of application.45 Certain weather conditions (including hot, windy environments) can favour entrance of ATZ into the atmosphere. For this reason, in Midwestern USA, a third of ATZ used enters the atmosphere. In Minnesota, 68.3% of ATZ found in Leech Lake originated from application sites 100–300 km away.46 Inhalation of ATZ-containing dust or spray particles by humans may cause local effects like asthma (including atopic and non-atopic wheeze)38,39,47 or they may enter the bloodstream (to cause potential systemic effects).48 The mode of application of pesticides to the crops, commonly using spray equipment, is a key determinant on the impact on health. A current gap in knowledge exists as to the extent and length of time required for ATZ to be absorbed via the lungs. Large particles can deposit in the pharynx or upper airways where they can be coughed up and swallowed via the mucociliary escalator.49 Smaller particles however can be inhaled deeper, into the alveolar region of the lung, the site of gas exchange and mediation of lung homeostasis, where they can interact with and damage the lung epithelial cells.50,51 Therefore, inhalation toxicity of ATZ is an important consideration both for the farmers during occupational use and for the general population in surrounding areas.
Due to the evidence discussed above, and elsewhere, the US EPA's decision to continue ATZ use has not been without controversy. In 2003, the EU concluded ATZ levels in groundwater would exceed 0.1 μg ml−1, despite appropriate use, and therefore banned its use in member states in 2005.52
For the US, Canada and other countries across the world with high ATZ usage, switching to alternative herbicide use could be problematic.53 The US utilises ATZ on an estimated 55% of corn crop (data from 2014) since ATZ is cheaper than its alternatives.54 This leads to an additional income of $1.45 billion annually and 6.8% greater corn yield.55–57 A ban on ATZ could have devastating impacts, reducing US gross corn sales by as much as one sixth and raising corn prices.55,56
Over the last three decades, nanotechnology – the application of materials on the nanoscale – has influenced many fields from medicine and cosmetics to food packaging. Researchers are exploring the beneficial properties of nanomaterials in farming to improve detection of agrochemicals and combat food spoilage using antimicrobial nanoparticle formulations.58,59 Nanotechnology can also aid agrochemical delivery to target plants. Nanoencapsulation – enclosing an active substance within a nano-sized shell, coating or membrane – has shown promise for this endeavour.60 Nano-sized particles are likely to be easily internalised by the plant vasculature, however little is known about how nanoencapsulation could affect the bioavailability, transport and fate of pesticides in the environment, including human and wildlife populations.
In the present study, we used poly(ε-caprolactone) (PCL) – a biodegradable, aliphatic and hydrophobic polyester, to form nanocapsules for ATZ delivery. PCL is biodegradable, harmless to the environment and degrades quickly, thus it is ideal for pesticide delivery.60 Nanoencapsulation prevents ATZ aggregation, increasing particle number per unit mass and therefore the efficacy of ATZ. The efficacy is further improved by the controlled release of ATZ from the PCL nanocapsule via relaxation of polymer chains; 45% and 65% release was observed after 24 and 48 hours respectively with a maximum release of 72% after 5.5 days.61 Furthermore, nanoencapsulated ATZ (NC–ATZ) is more evenly distributed allowing for greater bioavailability, thus fewer applications would be required to maintain efficacy, lowering cost and potential harm to the environment by reducing accumulation.62,63 Moreover, efficacy of NC–ATZ is maintained after a 10-fold dilution when applied to target mustard plants (Brassica species).9,61 Regarding the sourgrass plant (Digitaria insularis; a highly invasive weed), NC–ATZ induced greater and faster inhibition of photosystem II activity compared with conventional ATZ – even at half-dosage of NC–ATZ.64 Importantly, NC–ATZ has little genotoxicity and cytogeneticity against human leukocytes, being equivalent to deionised water, unlike toxic ATZ.65
Ongoing dermal research is further exploring the toxicity of NC–ATZ; however, inhalation exposure has previously not been investigated. On inhalation, a large percentage of nano-sized particles can reach the alveolar respiratory units, the site of gas exchange. The epithelium of the alveolar respiratory units is comprised of alveolar epithelial type 1 (AT1) and type 2 (AT2) cells. AT1 cells cover 95% of the alveolar surface thus will be a major target of inhaled nano-sized particles.66
We hypothesised on inhalation, ATZ would be toxic to human pulmonary alveolar epithelial cells (notably AT1 cells, see above). We further hypothesised that encapsulation of ATZ (NC–ATZ) would subdue the bioreactivity and toxicity of ATZ. We tested this hypothesis using a cell line derived from immortalised primary human alveolar epithelial cells, transduced type 1 (TT1) cells, to mimic the human alveolar epithelium in vitro.67 The ATZ concentrations used in this study were estimations derived from occupational exposures of those spraying the product. Normally 1 mg ml−1 ATZ is sprayed onto the crops but as a 10-fold dilution, NC–ATZ has the same efficacy. Once sprayed, ATZ becomes diluted in the air thus only a very small percentage of inhaled NCs would reach the alveoli.68 Consequently, we chose a wide range of concentrations (0.01–10 μg ml−1) to reflect the occupational and potential far-reaching general exposures. It is worth mentioning that nano-sized particles in the atmosphere take many hours or days to settle and can travel many miles, depending on weather conditions and prevailing winds, possibly impacting on the general population.
2. Materials and methods
2.1 Materials
Acetone (Sigma Aldrich), poly(ε-caprolactone) – average Mn 80
000 Da (Sigma Aldrich), Myritol® 318 – caprylic/capric triglyceride (BASF), Span® 60 (Sigma Aldrich), Tween® 80 (Sigma Aldrich), atrazine – PESTANAL® (Sigma Aldrich), 18
:
1 Liss Rhod PE [1,2-dioleoyl-sn-glycero-3-phosphoethanolamine-N-(lissamine rhodamine B sulfonyl) (ammonium salt), powder] (Avanti® Polar Lipids).
All other reagents were purchased from Sigma Aldrich unless stated otherwise.
2.2 Preparation of poly(ε-caprolactone) (PCL) nanocapsules and chemicals
PCL nanocapsules were prepared according to the interfacial deposition of pre-formed polymer described by Fessi et al.69 Firstly, the organic phase was prepared containing 30 ml of acetone, 100 mg of poly(ε-caprolactone), 200 mg of Myritol, 40 mg of Span 60 and 10 mg of atrazine (ATZ). The aqueous phase was composed of 30 ml of deionised water containing 60 mg of surfactant Tween 80. The organic phase was slowly added into the aqueous phase, with magnetic stirring. Subsequently, the resultant suspension was kept under magnetic stirring for 10 minutes, after which the organic solvent was evaporated using a rotatory evaporator. The suspension was evaporated to a final volume of 10 ml, resulting in a total atrazine concentration, in the nanocapsules, of 1 mg ml−1 with an encapsulation efficiency of 86.7 ± 0.7% (measured in an acetonitrile diluent, using high-performance liquid chromatography). Characterisation of NC–ATZ, including full methodology of encapsulation efficacy quantification, has been previously published by Grillo et al.61 For labelled nanoparticles, the fluorescent probe (18
:
1 Liss Rhod PE-Avanti® – 0.1% m of probe per m of oil – myritol) was added into the organic phase after polymer dissolution.
An ATZ solution of 1 mg ml−1 was prepared by dissolving diffuse ATZ in dimethyl sulfoxide (DMSO), ensuring that percentage weight of ATZ matched that of ATZ within the NC (NC–ATZ), so that direct comparison across both treatments could be made.
The nanocapsules in suspension (with and without ATZ) was approximately 7.5 × 1012 particles per ml. For treatment with the nanocapsule preparation, this was matched in concentration to the nanocapsule component of the NC–ATZ formula.
2.3 Evaluation of PCL nanocapsule containing atrazine (NC-ATZ) stability
NC–ATZ was diluted in defined cell culture medium-1 (DCCM-1) (Biological Industries, Israel) and incubated at 37 °C in a growth chamber. Samples were collected at 0 hours (0 h), 24 hours (24 h) and 48 hours (48 h) to evaluate nanoparticle characteristics by dynamic light scattering (DLS), and 0 h and 48 h for atomic force microscopy (AFM).
NC–ATZ in DCCM-1 was evaluated by DLS (ZetaSizer ZS90, Malvern Instruments, UK) using a scattering detection angle of 90 °C to acquire hydrodynamic size (nm), polydispersity index (PDI) and zeta potential (mV). Samples were analysed, in triplicate, at 25 °C.
NC–ATZ in DCCM-1 was diluted in ultrapure water (1
:
1000) and left to dry for 24 h at room temperature. Images were obtained by AFM (easyScan 2 Basic, Nanosurf, Switzerland), in contact mode using TapAI-G cantilevers (BudgetSensors, Bulgaria). Images were acquired with Gwyddion 2.50 software. Results are presented in ESI† Appendix A and B.
2.4 Culture of immortalised human alveolar type-1 like epithelial (TT1) cells
TT1 cells are an immortal alveolar type 1(AT1)-like cell line derived from primary human alveolar type 2 (AT2) cells, the precursors of AT1 cells in situ, as previously described by Kemp et al.67 TT1 cells were cultured in DCCM-1 supplemented with 10% newborn calf serum (NCS) and 1% penicillin–streptomycin–glutamine (PSG). Cells were seeded in 8 or 96 well plates at 7 × 104 or 1 × 105 cells/well respectively and grown to confluence (48 h) at 37 °C, 5% CO2, prior to exposure.
2.5 Sample exposures
TT1 cells were serum starved for 24 hours prior to exposure. PCL nanocapsule alone (NC), ATZ dissolved in DMSO (ATZ) and ATZ within the PCL nanocapsule (NC–ATZ) were diluted in fresh serum-free DCCM-1 to reach desired comparable concentrations (0.01–10 μg ml−1). Therefore, the maximum final percentage of DMSO used was 1%, present in the 10 μg ml−1 sample of ATZ. Vehicle controls included DMSO and Tween 80, using concentrations equivalent to those found in ATZ and NC–ATZ respectively. Serum-free DCCM-1 was used as a negative control. Cells were exposed for 1 hour (1 h), 24 hours (24 h) and 48 hours (48 h).
2.6 MTT (3-(4,5-dimethylthiazol-2-yl)-2,5-diphenyltetrazolium bromide) assay
TT1 cells were exposed to test samples for 24 h and 48 h. Zinc oxide (ZnO) nanoparticles (NPs) are known to induce toxicity to TT1 cells (at 10 μg ml−1) thus were used as a positive control.70 Following sample exposure, cells were incubated with MTT solution (500 μg ml−1 in DCCM-1) at 37 °C in a humidified 5% CO2 atmosphere for 25 minutes. MTT is reduced by mitochondrial reductases to produce purple formazan, a marker of metabolic activity, which can thus indirectly be used as a measure of cell viability as a loss of MTT reduction.71 The cells were washed with phosphate-buffered saline (PBS) before formazan solubilisation with ≥99.9% DMSO. The optical density was measured at 570 nm in an Infinite® F50 absorbance microplate reader (Tecan, Switzerland).
2.7 LDH (lactate dehydrogenase) assay
TT1 cells were exposed to test samples for 24 h and 48 h. 45 minutes prior to exposure endpoint, 10% lysis buffer was added to duplicate, unexposed wells, to cause LDH release, for use as a positive control. LDH is released extracellularly as a result of impaired plasma membrane integrity. Following sample exposure, the conditioned media was collected and stored for use at −20 °C. The assay was then carried out according to manufacturer's instructions (Pierce™ LDH Cytotoxicity Assay Kit, Thermo Fisher Scientific).72 The optical density was measured at 450 nm in an Infinite® F50 absorbance microplate reader (Tecan, Switzerland).
2.8 Enzyme-linked immunosorbent assay (ELISA)
TT1 cells were exposed to test samples for 24 h and 48 h. Following sample exposure, the conditioned media were collected and stored for use at −20 °C. Measurement of IL-6 and IL-8 release was then determined according to the manufacturer's instructions (Human IL-6/8 Standard TMB ELISA Development Kit, Peptotech, Inc.).73 The protocol was adapted for Greiner Bio-One 96 well half area microplates. The optical density was measured at 492 nm in an Infinite® F50 absorbance microplate reader (Tecan, Switzerland).
2.9 Measurement of intracellular reactive oxygen species (ROS)
Intracellular ROS was determined via two different assays using cell-permeant reagents: dihydroethidium (DHE; qualitative) and H2DCFDA (quantitative). In both cases, TT1 cells were exposed to test samples for 1 h and 24 h in order to capture a possibly early response in ROS production.74
For the DHE assay, following test sample exposure, the intact, adherent cells were incubated with DHE (10 μM in DCCM-1) at 37 °C in a humidified 5% CO2 atmosphere for 20 minutes. Cells were subsequently washed with PBS. Oxidation of DHE produces ethidium, resulting in red fluorescence, which was detected using an Axiovert 200 inverted fluorescent microscope (Zeiss, Germany) and captured as an image.
For the H2DCFDA assay, following sample exposure, intact, adherent cells were incubated with H2DCFDA (25 μM in DCCM-1) at 37 °C in a humidified 5% CO2 atmosphere for 30 minutes. ROS oxidation of H2DCFDA results in the formation of fluorescent 2,7-dichlorofluorscein (DCF). Cells were subsequently washed with PBS and disrupted by solubilisation in DMSO. The fluorescence intensity was determined on a FLUOstar OPTIMA microplate reader (BMG Labtech, Germany), at the Ex/Em of 485 nm/530 nm, and quantified as relative fluorescence units (RFU).
Results of the ROS formation assays are presented in ESI† Appendix D and E.
2.10 Confocal laser scanning microscopy
TT1 cells were exposed for 24 h to NC and NC–ATZ, which were fluorescently labelled with 18
:
1 Liss Rhod PE [1,2-dioleoyl-sn-glycero-3-phosphoethanolamine-N-lissamine rhodamine B sulfonyl]. Following sample exposure, cell membranes were stained with wheat germ agglutinin (WGA) Alexa Fluor™ 488 conjugate (5 μg ml−1 in PBS) then fixed with 4% formaldehyde for 10 minutes at 37 °C. Cell nuclei were stained with 4,6-diamidino-2-phenylindole (DAPI; 300 nM in PBS) for 10 minutes at 37 °C. A Leica TCS SP5 confocal microscope (Leica Microsystems, UK) was used to visualise ≥15 cells/well as well as acquire Z stack images at the following wavelengths: rhodamine-derivative Ex/Em 495 nm/519 nm, WGA Ex/Em 560 nm/583 nm and DAPI Ex/Em 358 nm/461 nm.
2.11 Statistical analysis
Data are presented as the mean ± standard deviation (SD) or standard error of the mean (SEM). A one-way analysis of variance (ANOVA) followed by Dunnett's post-hoc test was used to assess significant differences between all exposure regimens to non-treated controls using GraphPad Prism® software. p values < 0.05 were considered statistically significant.
3. Results
3.1 Effect of nanoencapsulated pesticide and pesticide treatment on TT1 cell viability (MTT assay)
As evidenced in Fig. 1, NC exposure induced a significant (**p < 0.01) change in mitochondrial activity at 0.1 μg ml−1 only, increasing 28% compared to the control after 24 h. There were no significant changes following NC exposure after 48 h. There was a trend towards a concentration-dependent decrease in cell viability at 24 h and 48 h post-ATZ exposure although only 10 μg ml−1 caused a significant reduction (28%, t = 24 h, ***p < 0.001; 19%, t = 48 h, **p < 0.01). However, DMSO (1%) alone caused a similar decline (approx. 30%, ***p < 0.001) at both time points, likely a reflection of its well-documented toxicity, and most likely this was the chief determinant of the cytotoxicity of ATZ alone. Interestingly, NC–ATZ stimulated MTT/mitochondrial activity 48 h post-exposure at 0.1 μg ml−1 (*p < 0.05), 1 μg ml−1 (***p < 0.001) and 10 μg ml−1 (**p < 0.01) suggesting cell activation and/or proliferation may have occurred. There were no significant changes in viability in the cells exposed to the Tween 80 vehicle control compared to the non-treated control cells. Zinc oxide (ZnO), a cytotoxic nanoparticle, was used as a positive control and caused a significant decrease in cell viability at 10 μg ml−1 (***p < 0.001) at both time points.
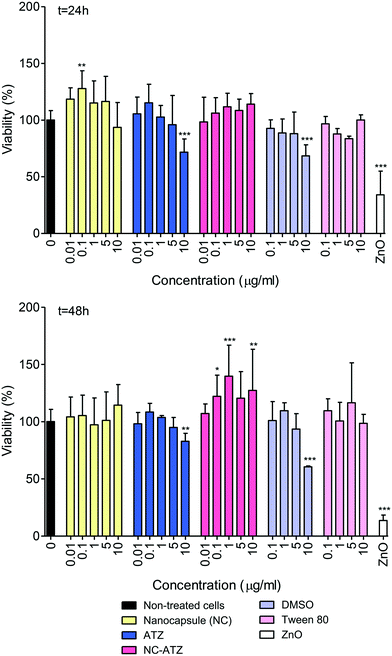 |
| Fig. 1 Cell viability of TT1 cells exposed to increasing concentrations of a PCL nanocapsule (NC), atrazine (ATZ) in DMSO, a PCL NC containing ATZ (NC–ATZ), vehicles DMSO and Tween 80 (T80), and Zinc Oxide (ZnO) nanoparticles for 24 h and 48 h. Concentrations of NC and ATZ were matched to the equivalent percentage weights in the NC–ATZ treatment. Concentrations of DMSO and T80 were matched to the equivalent percentage weights in the ATZ and NC–ATZ treatments respectively. Data are presented as a percentage of the non-treated control cells. Error bars are mean ± SD (n = 4–7). *p < 0.05, **p < 0.01, ***p < 0.001. | |
3.2 Effect of nanoencapsulated pesticide and pesticide treatment on TT1 cell viability (LDH assay)
As lactate dehydrogenase (LDH) release indicates impaired plasma membrane integrity, it indicates necrotic cell death. All data were compared to maximal potential LDH release due to 100% necrotic cell death (generated from lysed cells). As can be observed in Fig. 2, there were no significant increases in LDH release following exposure to any of the conditions at 24 h. NC–ATZ caused increased LDH release at higher concentrations (1 μg ml−1, *p < 0.05; 5 μg ml−1, ***p < 0.001 and 10 μg ml−1, **p < 0.01) peaking at 5 μg ml−1, with 3-fold greater LDH release compared to the non-treated cells, after 48 h exposure. Since NC–ATZ induced mitochondrial activity, NC–ATZ-induced necrosis is likely accompanied by activation and/or proliferation of residual cells. The positive assay control (+ve) showed a significant increase in LDH release (***p < 0.001) validating the assay reagents and methodology.
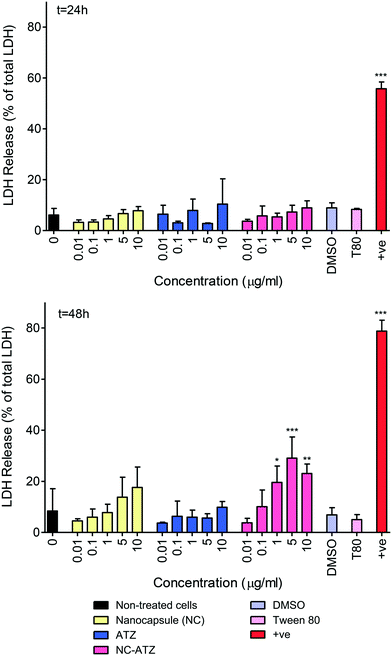 |
| Fig. 2 LDH release from TT1 cells exposed to increasing concentrations of a PCL nanocapsule (NC), atrazine (ATZ) in DMSO, a PCL NC containing ATZ (NC–ATZ), vehicles DMSO and Tween 80 (T80) and a positive control (+ve) for 24 h and 48 h. Concentrations of NC and ATZ were matched to the equivalent percentage weights in the NC–ATZ treatment. Concentrations of DMSO and T80 were matched to the equivalent percentage weights in the ATZ and NC–ATZ treatments respectively. Data are presented as a percentage of total cellular LDH (determined by LDH release from lysed control cells). Error bars are mean ± SD (n = 4–6). *p < 0.05, **p < 0.01, ***p < 0.001. | |
3.3 Effect of nanoencapsulated pesticide and pesticide treatment on inflammatory mediator release from TT1 cells
Release of inflammatory mediators, IL-6 and IL-8, by TT1 cells was quantified by ELISA (Fig. 3). NC–ATZ treatment induced a concentration-dependent increase with significant IL-6 release at 1 μg ml−1, 5 μg ml−1 and 10 μg ml−1 NC–ATZ releasing 8 pg ml−1, 9 pg ml−1 and 8 pg ml−1 of IL-6 respectively (*p < 0.05). This was amplified at 48 h (1 μg ml−1, *p < 0.05; 5 μg ml−1, ***p < 0.001; 10 μg ml−1, **p < 0.01) peaking at 5 μg ml−1 NC–ATZ exposure with 125 pg ml−1 IL-6 release. There was no significant change in IL-6 release by NC and ATZ exposed TT1 cells at either time point. IL-6 release increased significantly, compared to control, from TT1 cells exposed to DMSO (1%) at 24 h (*p < 0.05); IL-6 release was also significantly stimulated in TT1 cells exposed to DMSO (1%; *p < 0.05) at 48 h (Fig. 3 and see ESI† Appendix C, Fig. C).
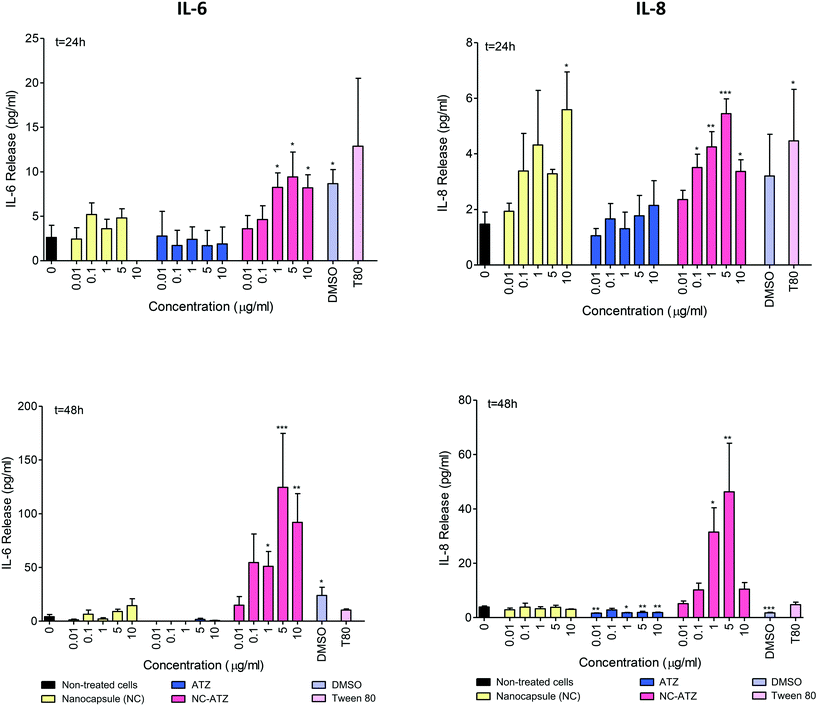 |
| Fig. 3 IL-6 and IL-8 release from TT1 cells exposed to increasing concentrations of a PCL nanocapsule (NC), atrazine (ATZ) in DMSO, a PCL NC containing ATZ (NC–ATZ), vehicles DMSO and Tween 80 (T80) for 24 h and 48 h. Concentrations of NC and ATZ were matched to the equivalent percentage weights in the NC–ATZ treatment. Concentrations of DMSO and T80 were matched to the equivalent percentage weights in the ATZ and NC–ATZ treatments respectively. Error bars are mean ± SEM (n = 3–5). *p < 0.05, **p < 0.01, ***p < 0.001. | |
Concentration-dependent trends of increasing IL-8 release by TT1 cells were observed after 24 h of exposure to NC, ATZ and NC–ATZ (Fig. 3). Only 10 μg ml−1 NC (*p < 0.05) and most concentrations of NC–ATZ (0.1 μg ml−1 and 10 μg ml−1, *p < 0.05; 1 μg ml−1, **p < 0.01; 5 μg ml−1, ***p < 0.001) were significantly increased, with NC–ATZ treatment peaking at 5 μg ml−1 with 5 pg ml−1 IL-8 release at 24 h. After 48 h of exposure, overall, NC did not stimulate significant IL-8 release (Fig. 3); however, there were significant decreases in IL-8 release caused by ATZ (0.01 μg ml−1, 5 μg ml−1 and 10 μg ml−1, **p < 0.01; 1 μg ml−1, *p < 0.05). NC–ATZ treatment (1 μg ml−1, *p < 0.05; 5 μg ml−1, **p < 0.01) significantly increased levels of IL-8 release, with levels peaking at 5 μg ml−1 with 46 pg ml−1 of NC–ATZ (**p < 0.01). Furthermore, significant IL-8 release was observed in response to 10 μg ml−1 Tween 80 exposure at 24 h (*p < 0.05) and DMSO (1%) at 48 h (***p < 0.001), perhaps as a result of cell death (Fig. 3 and see ESI† Appendix C, Fig. C).
3.4 Uptake of PCL nanoencapsulated pesticide by TT1 cells
Uptake of fluorescently-labelled NC and NC–ATZ was captured by confocal microscopy after 24 h of exposure (Fig. 4 and 5). NC (red) was readily internalised by TT1 cells, confirmed by visualising the NC inside the cells on the middle focal plane. The pan-staining indicates intracellular polymer degradation of the NC. Red fluorescence is visible in similar quantities at 0.01 μg ml−1 and 1 μg ml−1 NC, but less evenly distributed among cells at 10 μg ml−1 NC. Interestingly, when comparing the distribution of red fluorescent particles following NC and NC–ATZ exposure, NC appears to have more pan-staining at lower concentrations and overall.
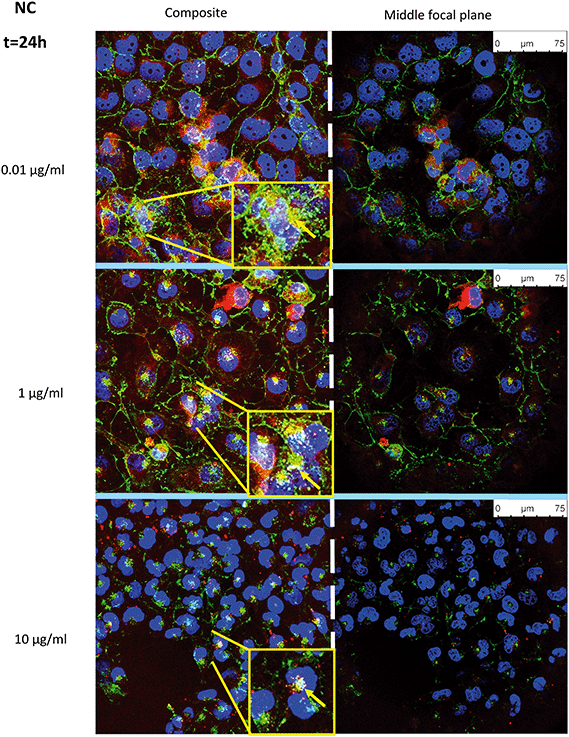 |
| Fig. 4 Visualisation of nanoparticle uptake by TT1 cells after 24 h of exposure to increasing concentrations of a fluorescently-labelled PCL nanocapsule (NC) (red). Concentrations of NC were matched to the equivalent percentage weights in the NC–ATZ treatment. Nuclei are stained with DAPI (blue) and membranes with WGA (green). Images on the left are a composite of all sections at any given concentration (demonstrating uptake) whilst images on the right depict the corresponding middle focal plane through the cells (confirming internalisation) (n = 1). | |
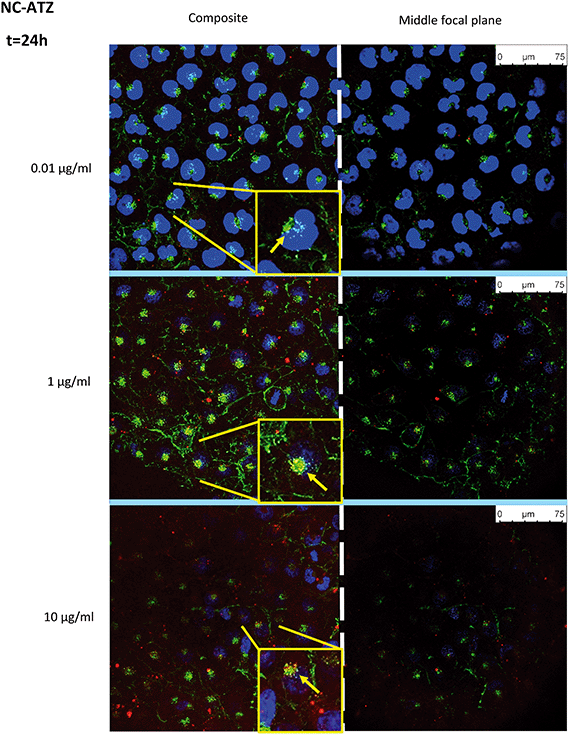 |
| Fig. 5 Visualisation of nanoparticle uptake by TT1 cells after 24 h of exposure to increasing concentrations of a fluorescently-labelled PCL nanocapsule (NC) (red) containing ATZ. Nuclei are stained with DAPI (blue) and membranes with WGA (green). Images on the left are a composite of all sections at any given concentration (demonstrating uptake) whilst images on the right depict the corresponding middle focal plane through the cells (confirming internalisation) (n = 1). | |
Co-localisation of the NP and membranes (yellow) suggests NC uptake into the membrane-rich Golgi-apparatus, in close apposition to the nucleus. This is shown more explicitly within magnified inserts (Fig. 4 and 5, yellow arrows).
4. Discussion
This study revealed some very striking differences between the bioreactivity of ATZ and nanoencapsulated ATZ (NC–ATZ) with human lung alveolar epithelial type 1-like cells. Over a period of 48 h, NC–ATZ caused increased TT1 cell mitochondrial activity, indicative of either cell activation and/or cell proliferation, and yet induced LDH release – a marker of necrotic cell death – whilst stimulating release of proinflammatory mediators. Different response patterns were observed with the NC and ATZ alone. TT1 cells readily internalised the NC as well as NC–ATZ, which produced a staining pattern indicative of cytoplasmic dispersal and recycling of the capsules, presumably releasing the drug intracellularly. Interestingly, there were no changes in ROS during the first 24 h despite significantly elevated levels of IL-6 and IL-8 in response to NC–ATZ exposure.
The bioreactivity of nanoencapsulated ATZ is likely to be dependent on size, shape, composition, charge and surface chemistry of the NC, as has been demonstrated by studies of nanoparticle toxicity.75–77 Grillo et al. demonstrated NC–ATZ size and shape remains similar to NC, however, other properties may be different due to the process of combining the NC and ATZ.61 Characteristics of NC–ATZ may also differ in the cell culture media used in this study. Previous research on human leukocytes, algae and plants cells showed NC–ATZ to be safer than ATZ alone, although microcrustaceans were more sensitive to NC–ATZ toxicity.61,62,65 Thus, it is likely that NC–ATZ has differential effects depending on the organism and cell targets. It is important to appreciate that TT1 cells have been shown to internalise nanoparticles via both passive (passing through cell membranes without using specific processes or cell energy) and active (largely endocytic) processes.74,78,79In situ, such processes enhance transportation of small molecules across the alveolar barrier. Thus, the observation of NC uptake aligns with our previous findings.74,80,81 Our other studies of TT1 cells have shown that surface chemistry and charge of nanoparticles impacts on cell death.80,81 Amine-modified polymer NPs permeabilise TT1 cell plasma membranes, inducing ROS and apoptotic cell death, whereas the same concentrations of neutral and negatively-charged NPs have little effect on TT1 cells. On the other hand, negatively charged NPs induce apoptotic cell death in cardiomyocytes by inducing ROS and mitochondrial damage.80,81 Thus, altered NC surface chemistry during processing could account for the heightened bioreactivity of NC–ATZ seen here. Alternatively, since polymer NPs degrade within 24 h and significant effects on cell viability and LDH release are only seen after 48 h (Fig. 1 and 2), perhaps a degradation product is causing this increased harmful effect compared to ATZ alone.82–84
IL-6 is released acutely in response to pathogen-associated molecular patterns through signalling pathways and activation of transcription factors e.g. NF-κB. IL-8 is an important neutrophil chemoattractant, inducing neutrophil migration and activation, e.g. degranulation and ROS production. These cytokines were investigated due to their role in many inflammatory lung diseases. NC–ATZ was proinflammatory at 24 h and 48 h (Fig. 3), reflecting activation of pattern recognition receptors, e.g. toll-like receptors.85 Acute increases in IL-6 and IL-8 have been associated with lung injury and, in severe cases, acute respiratory distress syndrome.86,87 However the relatively low concentrations released following NC–ATZ exposure may not be physiologically relevant. Conversely, AT1 cells cover 95% of the alveolar surface area and such a marked increase in release of IL-6 and IL-8 at the gas liquid interface of the lung as observed here, may be problematic. Moreover, the increase in mitochondrial activity seen following exposure to NC–ATZ for 48 h supports the suggestion that the cells have been activated. Chronic levels of IL-6 and IL-8 are associated with inflammatory lung diseases, therefore the effect of chronic exposure to NC–ATZ on cytokine release from TT1 cells is an important consideration.88,89
Inflammatory signalling often involves the generation of ROS; interestingly, ROS was unchanged compared to non-treated controls (see ESI† Appendix E, Fig. E). Visual assessment indicated that the DHE assay succeeded, showing deep red cells at baseline levels (see Appendix D, Fig. D1 and D2).
Holes in the monolayer appeared following exposure to NC–ATZ for 24 h, which subsequently were observed at higher concentrations of NC and NC–ATZ treatment after 48 h (see Appendix D, Fig. D1 and D2). Necrotic cell death may have contributed to the impaired monolayer observed, particularly for NC–ATZ 48 h exposure (Fig. 2). Cell death, as indicated by LDH release, and loss of adherent cells may account for the low detection of cellular ROS. In addition, these NCs may interfere with TT1 tight junctions, as observed with 300 nm carbon nanotubes.78 Decrease of alveolar barrier integrity would be problematic in vivo, possibly leading to increased risk of respiratory infections as well as unwanted access to the bloodstream and other organs.75
Confocal microscopy showed pan staining of fluorescently-labelled NC uptake by TT1 cells indicating intracellular polymer degradation and cytosolic dispersion of the NC constituents (Fig. 4 and 5), which previous studies suggest occurs within 24 h.80,81 These NCs are 240–260 nm thus are likely to be endocytosed via clathrin-coated pits or micropinocytosis.61,75,79 Co-localisation of the NC and membrane (yellow) suggests uptake of NC into the Golgi apparatus and subsequent recycling (Fig. 4 and 5). The contrasting confocal images for NC and NC–ATZ are evidence of differential TT1 cell interactions. Lower fluorescence following NC–ATZ uptake compared to NC uptake suggests differing uptake mechanisms and cellular dynamics, where the proinflammatory processes might have (as yet unknown) knock-on effects.80,81
Overall, these experiments suggest NC–ATZ is more harmful compared to NC or ATZ alone. This highlights the importance of in vitro research prior to experimentation ex vivo or in animal models. Further work could investigate other factors influencing the effect of NC–ATZ in vivo using different exposure scenarios. Within the alveoli, macrophages are likely to take up a large proportion of NC–ATZ and AT2 cells produce surfactant, which will further interact with the NCs. Surfactant may alter alveolar macrophage uptake and toxicity of NC–ATZ, whilst the nanoparticle may alter the function of surfactant (including its ability to provide a biological barrier) and precipitate disease.90 To investigate the impact of surfactant, air–liquid interface conditions would be required (compared to the submerged culture conditions in this study) and would be provide a greater understanding of the physiological interactions of NC–ATZ within the alveoli.91 Additionally, other body systems will be exposed to NC–ATZ. Modification of the NC properties and construction of the NC–ATZ particles could also be investigated to provide a safer product. Establishing the effects of novel nanoencapsulated pesticides on alveolar epithelial co-cultures and other body systems (primarily dermal or gastrointestinal) would further our understanding of the possible effects in vivo. Further characterisation of NC–ATZ within these models is likely to provide a better understanding of the mechanisms underpinning its bioreactivity. Finally, comparing the results obtained with NC–ATZ with commercial formulations would be useful to better understand the effects of surfactants and additives used in typical atrazine herbicide formulations in comparison to those used in NC–ATZ preparations.
5. Conclusion
Although previous research suggested nanoencapsulation of ATZ was safer (less cytotoxic and genotoxic) to human cells, this study revealed that it may be more bioreactive compared to ATZ alone if inhaled. The differences observed between NC and NC–ATZ cannot be attributed to ATZ alone, suggesting altered properties of the nanoencapsulated pesticide. A change in the physicochemical properties of the NC may be responsible. To better understand/modify mechanisms of NC–ATZ toxicity, different nanomaterial properties of NC and NC–ATZ must be determined/modified. Nanomaterials have brought many advantages to science, but we are yet to be fully cognisant of their behaviours.
Although this study suggests nanoencapsulation is more harmful compared to ATZ alone, the improved herbicidal efficacy with nanoencapsulation could significantly reduce environmental contamination and respiratory exposure. This may be of vital importance in countries where pesticide regulation and respiratory protection is less stringent. Therefore, research into PCL nanoencapsulation of ATZ should be continued and further developed, to improve its biocompatibility in humans and other organisms.
Conflicts of interest
The authors declare no conflicts of interest.
Acknowledgements
Dr. Renata de Lima and Dr. Leonardo F. Fraceto would like to thank São Paulo Research Foundation (FAPESP, #16/50003-4 and #17/21004-5) and CNPq, and also Dr. Estefânia V. Ramos Campos for the nanocapsule preparation and Dr. Anderson do Espirito Santo Pereira for nanoparticle characterization. Professor Teresa Tetley and Dr Lareb Dean recieved funding from the National Institute for Health Research Health Protection Research Unit (NIHR HPRU) in Health Impact of Environmental Hazards at King's College London in partnership with Public Health England (PHE) in collaboration with Imperial College London, London, UK.
References
- United Nations, Department of Economic and Social Affairs, Population Division, World Population Prospects: The 2015 Revision, Key Findings and Advance Tables. United Nations, New York, Working paper number: ESA/P/WP.241. 2015, https://esa.un.org/unpd/wpp/publications/files/key_findings_wpp_2015.pdf, (accessed November 2021).
- F. P. Carvalho, Pesticides, environment, and food safety, Food Energy Secur., 2017, 6, 48–60 CrossRef.
- S. Savary, A. Ficke, J. N. Aubertot and C. Hollier, Crop losses due to diseases and their implications for global food production losses and food security, Food Secur., 2012, 4, 519–537 CrossRef.
-
D. Pimentel and M. Burgess, Environmental and economic costs of the application of pesticides primarily in the United States, Integrated Pest Management, ed. D Pimentel and R Peshin, Springer, Dordrecht, 2014, vol. 3, pp. 47–71 Search PubMed.
- M. Salazar-Ledesma, B. Prado, O. Zamora and C. Siebe, Mobility of atrazine in soils of a wastewater irrigated maize field, Agric., Ecosyst. Environ., 2018, 255, 73–83 CrossRef CAS.
- J. Liu, J. H. Zhou, Q. N. Guo, L. Y. Ma and H. H. Yang, Physiochemical assessment of environmental behaviours of herbicide atrazine in soils associated with its degradation and bioavailability to weeds, Chemosphere, 2021, 262, 127830 CrossRef CAS PubMed.
-
D. Atwood and C. Paisley Jones, Pesticides Industry Sales and Usage, 2008–2012 Market Estimates, U.S. Environmental Protection Agency, https://www.epa.gov/sites/production/files/2017-01/documents/pesticides-industry-sales-usage-<?pdb_no 2016?>2016<?pdb END?>_0.pdf, (accessed November 2021).
-
J. A. Ferrell and B. A. Sellers, Approximate Herbicide Pricing, The Institute of Food and Agricultural Sciences, University of Florida, Publication #SS-AGR-16, https://edis.ifas.ufl.edu/wg056, (accessed November 2021).
- H. C. Oliveira, R. Stolf-Moreira, C. B. R. Martinez, R. Grillo, M. B. de Jesus and L. F. Fraceto, Nanoencapsulation enhances the post-emergence herbicidal activity of atrazine against mustard plants, PLoS One, 2015, 10, e0132971 CrossRef PubMed.
- W. C. Koskinen and S. A. Clay, Factors affecting atrazine fate in north central US soils, Rev. Environ. Contam. Toxicol., 1997, 151, 117–165 CAS.
- R. D'Amico, F. Monaco, R. Fusco, R. Siracusa, D. Impellizzeri, A. F. Peritore, R. Crupi, E. Gugliandolo, S. Cuzzocrea, R. Di Paola, T. Genovese and M. Cordaro, Atrazine inhalation worsens pulmonary fibrosis regulating the Nuclear Factor-Erythroid 2-Related Factor (Nrf2) pathways inducing brain comorbidities, Cell. Physiol. Biochem., 2021, 55, 704–725 CrossRef PubMed.
- R. D'Amico, F. Monaco, R. Fusco, A. F. Peritore, T. Genovese, D. Impellizzeri, R. Crupi, L. Interdonato, A. M. Sforza, E. Gugliandolo, R. Siracusa, S. Cuzzocrea, M. Cordaro and R. Di Paola, Exposure to atrazine induces lung inflammation through Nrf2-HO1 and Beclin 1/LC3 Pathways, Cell. Physiol. Biochem., 2021, 55, 413–427 CrossRef PubMed.
- T. Genovese, R. Siracusa, R. Fusco, R. D'Amico, D. Impellizzeri, A. F. Peritore, R. Crupi, E. Gugliandolo, R. Morabito, S. Cuzzocrea, A. T. Salinaro, M. Cordaro and R. Di Paola, Atrazine inhalation causes neuroinflammation, apoptosis and accelerating brain aging, Int. J. Mol. Sci., 2021, 22, 7938 CrossRef CAS PubMed.
- T. Hayes, K. Haston, M. Tsui, A. Hoang, C. Haeffele and A. Vonk, Atrazine-induced hermaphroditism at 0.1 ppb in American leopard frogs (Rana pipiens): laboratory and field evidence, Environ. Health Perspect., 2003, 111, 568 CrossRef CAS PubMed.
- L. Tavera-Mendoza, S. Ruby, P. Brousseau, M. Fournier, D. Cyr and D. Marcogliese, Response of the amphibian tadpole (Xenopus laevis) to atrazine during sexual differentiation of the testis, Environ. Toxicol. Chem., 2002, 21, 527–531 CrossRef CAS.
- L. Sai, L. Li, C. Hu, B. Qu, Q. Guo, Q. Jia, Y. Zhang, C. Bo, X. Li, H. Shao, J. C. Ng and C. Peng, Idenitification of circular RNAs and their alterations involved in developing male Xenopus laevis chronically exposed to atrazine, Chemosphere, 2018, 200, 295–301 CrossRef CAS PubMed.
- G. Q. Saalfeld, A. S. V. Junior, T. Castro, F. A. Pereira, S. M. M. Gheller, A. C. da Silva, C. D. Corcini, C. E. da Rosa and E. P. Colares, Low atrazine dosages reduce sperm quality of Calomys laucha mice, Environ. Sci. Pollut. Res., 2018, 25, 2924–2931 CrossRef CAS PubMed.
- L. E. Cook, B. J. Finger, M. P. Green and A. J. Pask, Exposure to atrazine during puberty reduces sperm viability, increases weight gain and alters the expression of key metabolic genes in the liver of male mice, Reprod., Fertil. Dev., 2019, 31, 920–931 CrossRef CAS PubMed.
- A. M. Khozimy, H. El-Danasoury and M. Abuzeid, Biochemical effects of treatments with herbicide atrazine in male albino rats, Journal of the Advances in Agricultural Researches, 2022, 4, 43–57 CrossRef.
- S. Zhu, T. Zhang, Y. Wang, X. Zhou, S. Wang and Z. Wang, Meta-analysis and experimental validation identified atrazine as a toxicant in the male reproductive system, Environ. Sci. Pollut. Res., 2021, 28, 37482–37497 CrossRef CAS PubMed.
- S. C. Laws, J. M. Ferrell, T. E. Stoker and R. L. Cooper, Pubertal development in female Wistar rats following exposure to propazine and atrazine biotransformation by-products, diamino-S-chlorotriazine and hydroxyatrazine, Toxicol. Sci., 2003, 76, 190–200 CrossRef CAS PubMed.
- J. Ashby, H. Tinwell, J. Stevens, T. Pastoor and C. B. Breckenridge, The effects of atrazine on the sexual maturation of female rats, Regul. Toxicol. Pharmacol., 2002, 35, 468–473 CrossRef CAS PubMed.
- J. B. Sass and A. Colangelo, European Union bans atrazine while the United States negotiates continued use, Int. J. Occup. Environ. Health, 2006, 12, 260–267 CrossRef CAS PubMed.
- J. T. Sanderson, R. J. Letcher, M. Heneweer, J. P. Giesy and M. van den Berg, Effects of chloro-s-triazine herbicides and metabolites on aromatase activity in various human cell lines and on vitelllogenin production in male carp hepatocytes, Environ. Health Perspect., 2001, 109, 1027–1031 CrossRef CAS PubMed.
- W. Q. Fan, T. Yanase, H. Morinaga, S. Gondo, T. Okabe, M. Nomura, T. Komatsu, K. I. Morohashi, T. B. Hayes, R. Takayanagi and H. Nawata, Atrazine-induced aromatase expression is SF-1 dependent: Implications for endocrine disruption in wildlife and reproductive cancers in humans, Environ. Health Perspect., 2007, 115, 720–727 CrossRef PubMed.
- S. H. Swan, R. L. Kruse, F. Liu, D. B. Barr, E. Z. Drobnis, J. B. Redmon, C. Wang, C. Brazil and J. W. Overstreet, Study for Future Families Research Group, Semen quality in relation to biomarkers of pesticide exposure, Environ. Health Perspect., 2003, 111, 1478–1484 CrossRef CAS PubMed.
- D. Yan, Y. Zhang, L. Liu, N. Shi and H. Yan, Pesticide exposure and risk of Parkinson's disease: Dose-response meta-analysis of observational studies, Regul. Toxicol. Pharmacol., 2018, 96, 57–63 CrossRef CAS PubMed.
- M. S. Islam, F. Azim, H. Saju, A. Zargaran, M. Shirzad, M. Kamal, K. Fatema, S. Rehman, M. A. M. Azad and S. E. Barough, Pesticides and Parkinson's disease: Current and future perspective, J. Chem. Neuroanat., 2021, 115, 101966 CrossRef CAS PubMed.
- A.-M. Aloizou, V. Siokas, E.-M. Sapouni, N. Sita, I. Liampa, A. G. Brotis, V. N. Rakitskii, T. I. Burykina, M. Aschner, D. P. Bogdanos, A. Tsatsakis, G. M. Hadjigeorgiou and E. Dardiotis, Parkinson's disease and pesticides: Are microRNAs the missing link?, Sci. Total Environ., 2020, 744, 140591 CrossRef CAS PubMed.
- J. Lin, H.-S. Zhao, L. Qin, X.-N. Li, C. Zhang, J. Xia and J.-L. Li, Atrazine triggers mitochondrial dysfunction and oxidative stress in quail (Coturnix C. coturnix) cerebrum via activating xenobiotic-sensing nuclear receptors and modulating cytochrome P450 systems, J. Agric. Food Chem., 2018, 66, 6402–6413 CrossRef CAS PubMed.
-
E. D. Kuempel and T. Sorahan, Identification of Research Needs to Resolve the Carcinogenicity of High-priority IARC Carcinogens, Views and Expert Opinions of an IARC/NORA Expert Group Meeting, Lyon, France, IARC Technical Publication No. 42, 2010 Search PubMed.
- J. Chen, J. Liu, S. Wu, W. Lu, Y. Xia, J. Zhao, Y. Yang, Y. Wang, Y. Peng and S. Zhao, Atrazine promoted epithelial ovarian cancer cells proliferation and metastasis by inducing low dose Reactive Oxygen Species (ROS), Iran. J. Biotechnol., 2021, 19, e2623 Search PubMed.
- F. Wang, Q.-W. Yang, W.-J. Zhao, Q.-Y. Du and Z.-J. Chang, Effects of short-time exposure to atrazine on miRNA expression profiles in the gonad of common carp (Cyprinus carpio), BMC Genomics, 2019, 20, 587 CrossRef PubMed.
- L. T. Wetzel, L. G. Luempert III, C. B. Breckenridge, M. O. Tisdel, J. T. Stevens, A. K. Thakur, P. J. Extrom and J. C. Eldridge, Chronic effects of atrazine on estrus and mammary tumor formation in female Sprague-Dawley and Fischer 344 rats, J. Toxicol. Environ. Health, Part A, 1994, 43, 169–182 CAS.
- R. L. Cooper, S. C. Laws, P. C. Das, M. G. Narotsky, J. M. Goldman, E. L. Tyrey and T. E. Stoker, Atrazine and reproductive function: Mode and mechanism of action studies, Birth Defects Res., Part B, 2007, 80, 98–112 CrossRef CAS PubMed.
- K. S. Estes, J. W. Simpkins and S. P. Kalra, Normal LHRH neuronal function and hyperprolactinemia in old pseudopregnant Fischer 344 rats, Neurobiol. Aging, 1982, 3, 247–252 CrossRef CAS PubMed.
- J. C. Eldridge, M. K. Tennant, L. T. Wetzel, C. B. Breckenridge and J. T. Stevens, Factors affecting mammary tumor incidence in chlorotriazine-treated female rats: Hormonal properties, dosage, and animal strain, Environ. Health Perspect., 1994, 102, 29–36 CrossRef CAS PubMed.
- J. A. Rusiecki, A. De Roos, W. J. Lee, M. Dosemeci, J. H. Lubin, J. A. Hoppin, A. Blair and M. C. Alavanja, Cancer incidence among pesticide applicators exposed to atrazine in the Agricultural Health Study, J. Natl. Cancer Inst., 2004, 96, 1375–1382 CrossRef CAS PubMed.
- L. E. B. Freeman, J. A. Rusiecki, J. A. Hoppin, J. H. Lubin, S. Koutros, G. Andreotti, S. H. Zahm, C. J. Hines, J. B. Coble, F. Barone-Adesi, J. Sloan, D. P. Sandler, A. Blair and M. C. R. Alavanja, Atrazine and cancer incidence among pesticide applicators in the Agricultural Health Study (1994-2007), Environ. Health Perspect., 2011, 119, 1253–1259 CrossRef CAS PubMed.
- A. J. De Roos, S. Zahm, K. Cantor, D. Weisenburger, F. Holmes, L. Burmeister and A. Blair, Integrative assessment of multiple pesticides as risk factors for non-Hodgkin's lymphoma among men, Occup. Environ. Med., 2003, 60, e11 CrossRef CAS PubMed.
- T. T. Hoang, C. Qi, K. C. Paul, M. Lee, J. D. White, M. Richards, S. S. Auerbach, S. Long, S. Shrestha, T. Wang, L. E. B. Freeman, J. N. Hoffman, C. Parks, BIOS Consortium, C.-J. Xu, B. Ritz, G. H. Koppelman and S. J. London, Epigenome-wide DNA methylation and pesticide use in the Agricultural Lung Health study, Environ. Health Perspect., 2021, 129, 97008 CrossRef CAS PubMed.
-
R. J. Gilliom, J. E. Barbash, C. G. Crawford, P. A. Hamilton, J. D. Martin, N. Nakagaki, L. H. Nowell, J. C. Scott, P. E. Stackelberg, G. P. Thelin and D. M. Wolock, Pesticides in the nation's streams and ground water 1992–2001, National Water-Quality Assessment Program, U.S. Geological Survey Circular 1291, 2006, https://pubs.usgs.gov/circ/2005/1291/pdf/circ1291.pdf, (accessed November 2021).
- United States Environmental Protection Agency, Washington D.C 20460, Atrazine, Draft Human Health Risk Assessment, 2018, https://www.regulations.gov/document?D=EPA-HQ-OPP-2013-0266-1159, (accessed November 2021).
-
D. S. Carter, M. J. Lydy and C. G. Crawford, Water-quality assessment of the White River Basin, Indiana: Analysis of available information on pesticides, US Geological Survey 1972–92, 1995, https://www.in.gov/dnr/water/files/WFWR_web50-119.pdf, (accessed November 2021).
- U.S. Department of Health and Human Services, Public Health Service, Agency for Toxic Substances and Disease Registry (ATSDR), Toxicological profile for atrazine, 2003, https://www.atsdr.cdc.gov/ToxProfiles/tp153.pdf, (accessed November 2021).
-
M. Cohen, B. Commoner, P. Bartlett, P. Cooney and H. Eisl, Exposure to endocrine disruptors from long-range air transport of pesticides. Center for the Biology of Natural Systems, Queens College, 1997, https://www.academia.edu/5712627/Exposure_of_endocrine_disrupters_from_long-range_air_transport_of_, (accessed November 2021).
- J. A. Hoppin, D. M. Umbach, S. Long, S. J. London, P. K. Henneberger, A. Blair and D. P. Sandler, Pesticides are associated with allergic and non-allergic wheeze among male farmers, Environ. Health Perspect., 2017, 125, 535–543 CrossRef CAS PubMed.
- R. Ikonen, J. Kangas and H. Savolainen, Urinary atrazine metabolites as indicators for rat and human exposure to atrazine, Toxicol. Lett., 1988, 44, 109–112 CrossRef CAS PubMed.
- S. Ganesan, A. T. Comstock and U. S. Sajjan, Barrier function of airway tract epithelium, Tissue Barriers, 2013, 1, e24997 CrossRef PubMed.
- K. Donaldson, L. Tran, L. A. Jimenez, R. Duffin, D. E. Newby, N. Mills, W. MacNee and V. Stone, Combustion-derived nanoparticles: A review od their toxicology following inhalation exposure, Part. Fibre Toxicol., 2005, 2, 10 CrossRef PubMed.
- H. Schulz, Mechanisms and factors affecting intrapulmonary particle deposition: Implications for efficient inhalation and therapies, Pharm. Sci. Technol. Today, 1998, 1, 326–344 CrossRef.
- Health and Consumer Protection Directorate-General, Scientific Committee on Plants, Opinion of the Scientific Committee on Plants on specific questions from the Commission concerning the evaluation of atrazine in the context of council directive 91/414/EEC, Brussels, Belgium: European Commission, 2003, https://ec.europa.eu/transparency/regdoc/rep/10102/2016/EN/SWD-2016-211-F1-EN-MAIN-PART-10.PDF, (accessed November 2021).
- N. D. Jablonowski, A. Schaffer and P. Burauel, Still present after all these years: Persistence plus potential toxicity raise questions about the use of atrazine, Environ. Sci. Pollut. Res., 2011, 18, 328–331 CrossRef CAS PubMed.
- C. M. Benbrook, Trends in glyphosate herbicide use in the United States and globally, Environ. Sci. Eur., 2016, 28, 3 CrossRef PubMed.
- F. Ackerman, The economics of atrazine, Int. J. Occup. Environ. Health, 2007, 13, 437–445 CrossRef PubMed.
- United States Environmental Protection Agency, Biological and Economic Analysis Division, Assessment of Potential Mitigation Measures for Atrazine, 2002, https://ppp.purdue.edu/wp-content/uploads/2016/08/ABA-02-03.pdf, (accessed November 2021).
-
R. S. Fawcett and I. A. Huxley, Two Decades of Atrazine Yield Benefits Research, Triazine Network, 2006.
- S. M. Rodrigues, P. Demokritou, N. Dokoozlian, C. O. Hendren, B. Karn, M. S. Mauter, O. A. Sadik, M. Safarpour, J. M. Unrine, J. Viers, P. Welle, J. C. White, M. R. Wiesner and G. V. Lowry, Nanotechnology for sustainable food production: Promising opportunities and scientific challenges, Environ. Sci.: Nano, 2017, 4, 767–781 RSC.
- J. Machell, K. Prior, R. Allan and J. M. Andresen, The water energy food nexus–challenges and emerging solutions, Environ. Sci.: Water Res. Technol., 2015, 1, 15–16 RSC.
- M. Nuruzzaman, M. M. Rahman, Y. Liu and R. Naidu, Nanoencapsulation, nano-guard for pesticides: a new window for safe application, J. Agric. Food Chem., 2016, 64, 1447–1483 CrossRef CAS PubMed.
- R. Grillo, N. Z. dos Santos, C. R. Maruyama, A. H. Rosa, R. de Lima and L. F. Fraceto, Poly (ε-caprolactone) nanocapsules as carrier systems for herbicides: Physico-chemical characterization and genotoxicity evaluation, J. Hazard. Mater., 2012, 231–232, 1–9 CrossRef CAS PubMed.
- A. E. Pereira, R. Grillo, N. F. Mello, A. H. Rosa and L. F. Fraceto, Application of poly (epsilon-caprolactone) nanoparticles containing atrazine herbicide as an alternative technique to control weeds and reduce damage to the environment, J. Hazard. Mater., 2014, 268, 207–215 CrossRef CAS PubMed.
- S. N. M. Yusoff, A. Kamari and N. F. A. Aljafree, A review of materials used as carrier agents in pesticide formulations, Int. J. Environ. Sci. Technol., 2016, 13, 2977–2994 CrossRef.
- B. T. Sousa, A. E. S. Pererira, L. F. Fraceto, H. C. de Oliveira and G. Dalazen, Effectiveness of nanoatrazine in post-emergent control of the tolerant weed Digitaria insularis, J. Plant Prot. Res., 2020, 60, 185–192 CAS.
- Z. Clemente, R. Grillo, M. Jonsson, N. Santos, L. O. Feitosa, R. Lima and L. F. Fraceto, Ecotoxicological evaluation of poly (ε-caprolactone) nanocapsules containing triazine herbicides, J. Nanosci. Nanotechnol., 2014, 14, 4911–4917 CrossRef CAS PubMed.
- J. D. Crapo, B. E. Barry, P. Gehr, M. Bachofen and E. R. Weibel, Cell number and cell characteristics of the normal human lung, Am. Rev. Respir. Dis., 1982, 126, 332–337 CAS.
- S. J. Kemp, A. J. Thorley, J. Gorelik, M. J. Seckl, M. J. O'Hare, A. Arcaro, Y. Korchev, P. Goldstraw and T. D. Tetley, Immortalization of human alveolar epithelial cells to investigate nanoparticle uptake, Am. J. Respir. Cell Mol. Biol., 2008, 39, 591–597 CrossRef CAS PubMed.
- W. Yang, J. I. Peters and R. O. Williams III, Inhaled nanoparticles - a current review, Int. J. Pharm., 2008, 356, 239–247 CrossRef CAS PubMed.
-
H. Fessi, F. Puisieux and J. P. Devissaguet, Procédé de préparation de systèmes colloïdaux dispersibles d'une substance sous forme de nanocapsules, European Pat., 274961A1, 1988 Search PubMed.
- J. Zhang, X. Qin, B. Wang, G. Xu, Z. Qin, J. Wang, L. Wu, X. Ju, D. D. Bose, F. Qiu, H. Zhou and Z. Zou, Zinc oxide nanoparticles harness autophagy to induce cell death in lung epithelial cells, Cell Death Dis., 2017, 8, e2954 CrossRef CAS PubMed.
-
T. L. Riss, R. A. Moravec, A. L. Niles, S. Duellman, H. A. Benink, T. J. Worzella and L. Minor, Cell viability assays, Assay Guidance Manual, ed. G. S. Sittampalam, K. Brimacombe, A. Grossman, M. Arkin, D. D. Auld, C. Austin, J. Baell, B. Bejcek, J. M. M. Caaveiro, T. D. Y. Chung, N. P. Cousens, J. L. Dahlin, V. Devanaryan, T. L. Foley, M. Glicksman, M. D. Hall, J. V. Haas, S. R. J. Hoare, J. Inglese, P. W. Iversen, S. D. Kahl, S. C. Kales, S. Kirshner, M. Lal-Nag, Z. Li, J. McGee, O. McManus, T. Riss, O. J. Trask, J. R. Weidner, M. J. Wildey, M. Xia and X. Xu, 2013, https://www.ncbi.nlm.nih.gov/books/NBK144065/, (accessed November 2021) Search PubMed.
- Thermo Fisher Scientific, Pierce LDH Cytotoxicity Assay Kit, https://www.thermofisher.com/order/catalog/product/88953, (accessed May 2018).
- Peprotech, ELISA: Sandwich TMB, https://www.peprotech.com/ELISA-Sandwich-TMB, (accessed May 2018).
- A. Katsumiti, A. J. Thorley, I. Arostegui, P. Reip, E. Valsami-Jones, T. D. Tetley and M. P. Cajaraville, Cytotoxicity and cellular mechanisms of toxicity of CuO NPs in mussel cells in vitro and comparative sensitivity with human cells, Toxicol. In Vitro, 2018, 48, 146–158 CrossRef CAS PubMed.
- K. L. Aillon, Y. Xie, N. El-Gendy, C. J. Berkland and M. L. Forrest, Effects of nanomaterial physicochemical properties on in vivo toxicity, Adv. Drug Delivery Rev., 2009, 61, 457–466 CrossRef CAS PubMed.
- H. C. Fischer and W. C. Chan, Nanotoxicity: the growing need for in vivo study, Curr. Opin. Biotechnol., 2007, 18, 565–571 CrossRef CAS PubMed.
- H. Sun, J. Cuijuan, L. Wu, X. Bai and S. Zhai, Cytotoxicity-related bioeffects induced by nanoparticles: the role of surface chemistry, Front. Bioeng. Biotechnol., 2019, 7, 414 CrossRef PubMed.
- P. Ruenraroengsak, S. Chen, S. Hu, J. Melbourne, S. Sweeney, A. J. Thorley, J. N. Skepper, M. S. Shaffer, T. D. Tetley and A. E. Porter, Translocation of functionalized multi-walled carbon nanotubes across human pulmonary alveolar epithelium: dominant role of epithelial type 1 cells, ACS Nano, 2016, 10, 5070–5085 CrossRef CAS PubMed.
- A. J. Thorley, P. Ruenraroengsak, T. E. Potter and T. D. Tetley, Critical determinants of uptake and translocation of nanoparticles by the human pulmonary alveolar epithelium, ACS Nano, 2014, 8, 11778–11789 CrossRef CAS PubMed.
- P. Ruenraroengsak, P. Novak, D. Berhanu, A. J. Thorley, E. Valsami-Jones, J. Gorelik, Y. E. Korchev and T. D. Tetley, Respiratory epithelial cytotoxicity and membrane damage (holes) caused by amine-modified nanoparticles, Nanotoxicology, 2012, 6, 94–108 CrossRef CAS PubMed.
- P. Ruenraroengsak and T. D. Tetley, Differential bioreactivity of neutral, cationic and anionic polystyrene nanoparticles with cells from the human alveolar compartment: robust response of alveolar type 1 epithelial cells, Part. Fibre Toxicol., 2015, 12, 19 CrossRef PubMed.
- T. Akagi, F. Shima and M. Akashi, Intracellular degradation and distribution of protein-encapsulated amphiphilic poly (amino acid) nanoparticles, Biomaterials, 2011, 32, 4959–4967 CrossRef CAS PubMed.
- A. Barthel, M. Dass, M. Droge, J. M. Cramer, D. Baumann, M. Urban, K. Landfester, V. Mailander and I. Lieberwirth, Imaging the intracellular degradation of biodegradable polymer nanoparticles, Beilstein J. Nanotechnol., 2014, 5, 1905–1917 CrossRef PubMed.
- A. K. Mohammad and J. J. Reineke, Quantitative detection of PLGA nanoparticle degradation in tissues following intravenous administration, Mol. Pharmaceutics, 2013, 10, 2183–2189 CrossRef CAS PubMed.
- C. Buzea, I. I. Pacheco and K. Robbie, Nanomaterials and nanoparticles: Sources and toxicity, Biointerphases, 2007, 2, MR17-71 CrossRef PubMed.
- B. T. Thompson, R. C. Chambers and K. D. Liu, Acute respiratory distress syndrome, N. Engl. J. Med., 2017, 377, 562–572 CrossRef CAS PubMed.
- G. U. Meduri, G. Kohler, S. Headley, E. Tolley, F. Stentz and A. Postlethwaite, Inflammatory cytokines in the BAL of patients with ARDS: Persistent elevation over time predicts poor outcome, Chest, 1995, 108, 1303–1314 CrossRef CAS PubMed.
- N. Mukaida, Pathophysiological roles of interleukin-8/CXCL8 in pulmonary diseases, Am. J. Physiol. Lung Cell Mol. Physiol., 2003, 284, L566–L577 CrossRef CAS PubMed.
- W. Stankiewicz, M. P. Dabrowski, A. Chcialowski and T. Plusa, Cellular and cytokine immunoregulation in patients with chronic obstructive pulmonary disease and bronchial asthma, Mediators Inflamm., 2002, 11, 307–312 CrossRef PubMed.
- C. A. Ruge, U. F. Schaefer, J. Herrmann, J. Kirch, O. Canadas, M. Echaide, J. Perez-Gil, C. Casals, R. Müller and C. M. Lehr, The interplay of lung surfactant proteins and lipids assimilates the macrophage clearance of nanoparticles, PLoS One, 2012, 7, e40775 CrossRef CAS PubMed.
- G. Wang, X. Zhang, X. Liu and J. Zheng, Co-culture of human alveolar epithelial (A549) and macrophage (THP-1) cells to study the potential toxicity of ambient PM2.5: a comparison of growth under ALI and submerged conditions, Toxicol. Res., 2020, 9, 636–651 CrossRef PubMed.
|
This journal is © The Royal Society of Chemistry 2022 |