Simultaneous removal of arsenite and cadmium by a manganese-crosslinking sodium alginate modified biochar and zerovalent iron composite from aqueous solutions†
Received
6th August 2021
, Accepted 27th October 2021
First published on 3rd December 2021
Abstract
Biochar (BC) pyrolyzed using solid waste has attracted increasing attention for wastewater treatment, while pure BC has limitations in the simultaneous removal of arsenite (As(III)) and cadmium (Cd(II)). Herein, a novel manganese-crosslinking sodium alginate modified biochar and zerovalent iron composite (Mn/SA-BC@nZVI) was prepared for the simultaneous removal of As(III) and Cd(II) from contaminated water. Mn/SA-BC@nZVI allowed for the simultaneous removal of As(III) (20 mg L−1) and Cd(II) (20 mg L−1), with efficiencies of >90% and >80% within 10 min, which were 17.18% and 28.23% higher than those of single As(III) and Cd(II) solution, respectively. Principally, this is attributed to the production of anion-bridged (MnO/FeO–As–Cd) and cation-bridged surface complexes (MnO/FeO–Cd–As) via surface multiple complexation, multiple H-bonds, electrostatic attraction, and precipitation. The Langmuir adsorption capacities of Mn/SA-BC@nZVI towards As(III) and Cd(II) were 121 and 120 mg g−1, respectively. The graphitized structure of Mn/SA-BC@nZVI promoted electron transfer and free radical production. As a result, part of As(III) was oxidized to arsenate (As(V)). The inner-sphere surface complexation of As(III) or As(V) with iron and manganese oxides was the main mechanism of As(III) removal. However, Cd–π binding with aromatic carbon components, surface complexation with oxygen-containing groups, and precipitation with CO32− were predominant in Cd(II) removal. Moreover, the current research provides an efficient adsorbent for the synchronous removal of As(III) and Cd(II) in wastewater treatment.
Environmental significance
The simultaneous removal of highly toxic anionic (e.g., As(III)) and cationic (e.g., Cd(II)) heavy metals has been a huge challenge in wastewater treatment. Here, we introduced a manganese-crosslinking sodium alginate modified biochar and zerovalent iron composite (Mn/SA-BC@nZVI). A synergistic effect of As(III) and Cd(II) removal by Mn/SA-BC@nZVI was found. The removal efficiency of As(III) and Cd(II) in a mixture solution increased by 17.18% and 28.23%, respectively, compared with that in a single As(III) or Cd(II) solution. The synergistic and single removal mechanisms of As(III) and Cd(II) were proposed. This knowledge will provide important implications for the effective solution of complex binary environmental problems.
|
1. Introduction
The presence of excessive amounts of arsenic (As) and cadmium (Cd) in the environment may pose a potential threat to human health due to their bioaccumulation and toxicity.1 Among the various As species, arsenite (As(III)) is the dominant valence state of As in the aquatic environment, deserving special attention because its toxicity is 25–60 times higher than arsenate (As(V)).2 Cd mainly exists in the form of cationic divalent Cd (Cd(II)) in the aquatic environment. As(III) and Cd(II) can coexist in mine drainage, smelting operations, and electroplating wastewater,3 resulting in the co-pollution of highly toxic anions and cations in soil and water. Therefore, the removal of As(III) and Cd(II) from contaminated water is of great importance to protect ecosystem safety and human health.4
Adsorption has been investigated extensively as an approach for the removal of As(III) or Cd(II) due to its superiority in cost, simplicity in design, lack of harmful byproducts, and ease of operation.5 Numerous adsorbents (e.g., biochar (BC),6–8 polypyrrole,9 layered double hydroxides,10 MOFs,11 and graphene oxide12) have been developed for the removal of highly toxic As(III) or Cd(II) from contaminated water. However, the synchronous removal of As(III) and Cd(II) has been an enormous challenge in water treatment processes.13 As a result, it is imperative to synthesize an efficient adsorbent that can simultaneously and rapidly remove As(III) and Cd(II) from water.
The extension of urban green areas in China has led to the increasing production of forestry waste, which has exceeded 14.4 million tons per year.14 Through reusing forestry waste, the preparation of materials using forestry waste is conducive to realizing the concept of “waste to resource”.15 The pyrolysis of forestry waste to BC materials provides a new approach for resource utilization, which has been widely investigated due to their low cost, high effectiveness, and easy application.16 BC has been extensively investigated as a potential adsorbent for removing As(III), Cd(II), and antibiotics.17–19 Nevertheless, its practical application in wastewater treatment is greatly limited by its low adsorption capacity and poor cooperativity towards highly toxic anions and cations. As a result, the modification of BC into advanced composites with novel surface properties plays a vital role in enhancing its practical application in environmental remediation.
Nanoscale zerovalent iron (nZVI) has been widely recognized as an effective adsorbent for the removal of highly toxic anions or cations.20,21 However, nZVI particles not only tend to coalesce into larger particles due to their strong van der Waals forces and magnetic attraction, but can also be easily oxidized, thus forming a passive iron oxide film on the outer surface.22,23 To overcome the above shortcomings, uniform immobilization and loading of nZVI onto the surface of carbon materials is a promising strategy, which can mitigate the oxidation of nZVI and enhance or integrate the properties of both materials for the adsorption of As(III) and Cd(II).
Additionally, the loading of manganese/iron (Mn/Fe) oxide particles on BC has gained significant attention, which can efficiently enhance the adsorption of highly toxic anions or cations by increasing the surface oxygen-containing functional groups and undergoing concurrent redox reactions.24 Unfortunately, the weak stability of BC modified by Mn salt soaking remains a problem for practical use, which is primarily due to the release of Mn. This will decrease the removal ability of the adsorbent towards the target pollutant and lead to serious Mn secondary pollution.25,26 Meanwhile, BC modified by Fe salt soaking has low adsorption of As(III) and Cd(II) because of the slow valence cycle and electron production,27 which restricts its widespread application in water treatment. In the current work, a Mn-crosslinking sodium alginate modified BC and zerovalent iron composite (Mn/SA-BC@nZVI) was prepared by an efficient and simple synthetic route. This study introduced a new crosslinking–precipitation and post-pyrolysis strategy to effectively immobilize Mn oxide on the surface of BC. In other words, Mn salt crosslinked with SA and precipitated on the surface of forestry waste. Then, the mixture was pyrolyzed to produce BC. The nZVI was subsequently loaded in situ on the surface of the modified BC, where the abundant carboxyl and hydroxyl groups dramatically reduced the release of Mn and Fe from the composite.
In the current work a new adsorbent (Mn/SA-BC@nZVI) was synthesized for the simultaneous removal of As(III) and Cd(II) from contaminated water. This study mainly aimed to (1) characterize the properties of Mn/SA-BC@nZVI; (2) determine the adsorption kinetics and capacity of Mn/SA-BC@nZVI for the removal of As(III) and Cd(II); (3) examine the synergistic removal of Mn/SA-BC@nZVI towards As(III) and Cd(II) in mixed solution; (4) explore the effects of coexisting ions, humic acid, and initial pH on the simultaneous removal of As(III) and Cd(II); and (5) discuss the single and collaborative removal mechanisms of As(III) and Cd(II) by Mn/SA-BC@nZVI.
2. Experimental section
2.1 Materials
The used materials are shown in the ESI.†
2.2 Preparation of Mn/SA-BC@nZVI
The Mn-crosslinking sodium alginate modified biochar (Mn/SA-BC) was prepared by in situ crosslinking and post-pyrolysis reactions, as presented in Scheme 1. In detail, 3 g forestry waste was added into 0.5 mol L−1 MnSO4 solution (200 mL) and mixed completely with the solution after 45 min of stirring. At the same time, 3 g SA was completely dissolved into 150 mL DI water at 60 °C. Subsequently, the SA solution was added into the forestry waste mixture solution using a peristaltic pump (Harvard Apparatus, USA) at 1 mL min−1. After stirring for 6 h at room temperature, the precipitates were filtered, washed using DI water several times, and oven-dried at 90 °C to a constant weight. Next, the precipitates were pyrolyzed at 600 °C for 2 h to give Mn/SA-BC. Mn/SA-BC was washed using DI water several times to remove impurities and oven-dried at 75 °C for at least 20 h. Finally, Mn/SA-BC was crushed, sieved, and stored for use in the subsequent experiments.
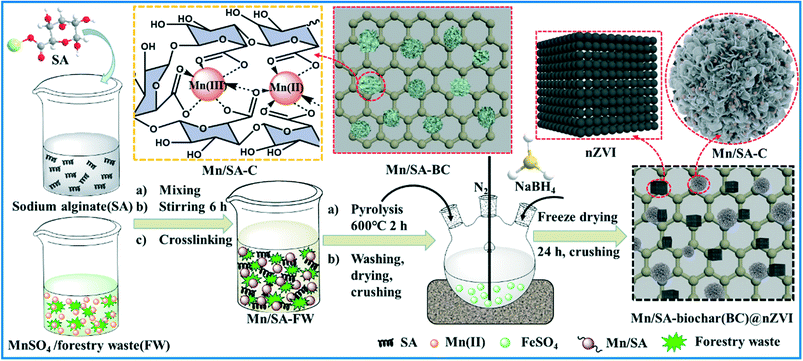 |
| Scheme 1 Schematic representation of Mn/SA-BC@nZVI synthesis. | |
Mn/SA-BC@nZVI was prepared through a liquid phase reduction–precipitation of nZVI on the surface of Mn/SA-BC (Scheme 1). Approximately 1.5 g Mn/SA-BC was added to 0.27 mol L−1 FeSO4 solution (100 mL) in a three-necked boiling flask and vigorously stirred at room temperature under a N2 atmosphere for 1 h. 0.8 mol L−1 NaBH4 solution (50 mL) was added into the flask. Subsequently, the precipitates were rapidly separated by centrifugation, washed using DI water and ethanol several times, and lyophilized at −48 °C for 24 h. Finally, the dried Mn/SA-BC@nZVI was crushed and stored at 4 °C for further use.
2.3 Characterization methods
The chemical characteristics of Mn/SA-BC@nZVI, including the specific surface area, chemical composition, crystal structure, textural properties, and morphology, were studied by various characterization techniques. The details are shown in the ESI.†
2.4 Adsorption experiments
Detailed information concerning the adsorption experiments and analytical approaches is shown in the ESI.†
3. Results and discussion
3.1 Characterization of Mn/SA-BC@nZVI
The surface elemental compositions and morphology of Mn/SA-BC, nZVI, and Mn/SA-BC@nZVI were visualized by scanning electron microscopy with energy dispersion spectrometry (SEM-EDS) (Fig. 1). The smooth surface of BC was uniformly covered with fine particles (Fig. 1a), which was attributed to the loading of Mn oxide/sulfide. The EDS analysis clearly demonstrated that the nanoparticles of Mn crosslinking SA (Mn/SA-C) were successfully loaded on the surface of BC (Fig. 1b). In comparison with the chain-like agglomerated structure of nZVI (Fig. 1c), the square granular nZVI was uniformly distributed on the surface of Mn/SA-BC (Fig. 1e), implying the important role of BC as a support for nZVI. The element compositions of Mn/SA-BC@nZVI were determined by EDS analysis, as shown in Fig. 1f, confirming that both Mn oxide/sulfide and Fe elements were possibly immobilized on the surface of BC.
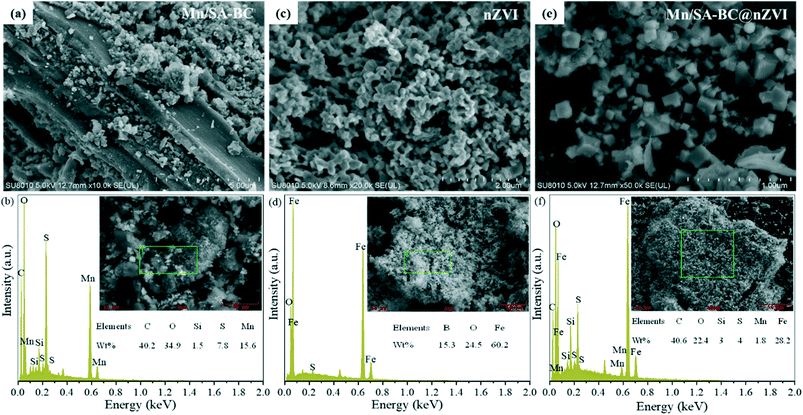 |
| Fig. 1 SEM images and SEM-EDS results of (a) and (b) Mn/SA-BC, (c) and (d) nZVI, and (e) and (f) Mn/SA-BC@nZVI. | |
The X-ray diffraction (XRD) patterns of Mn/SA-BC, nZVI, and Mn/SA-BC@nZVI are displayed in Fig. 2a. The XRD peaks of MnO (JCPDS 75-1090) at 2θ = 34.93°, 40.55°, 58.69°, 70.15°, 73.76° and 87.74° and of MnS2 (JCPDS 06-0518) at 2θ = 29.60°, 34.30°, 49.29°, 58.56°, 61.39°, 72.29°, and 82.50° were detected for Mn/SA-BC.28,29 Additionally, the values at 44.68°, 65.03°, and 82.34° corresponded to the crystal faces of (110), (200), and (211), respectively, belonging to cubic nZVI (JCPDS 87-0721).30 Regarding Mn/SA-BC@nZVI, the special diffraction peaks of MnO and MnS2 were reduced, which were possibly due to strong peak coverage with the introduction of the cubic nZVI. Concurrently, the peak width and intensity in the XRD pattern of Mn/SA-BC@nZVI are closely related to the crystallinity.6 Therefore, it was concluded that Mn/SA-BC@nZVI exhibited favorable crystallinity, primarily because of the introduction of nZVI on BC.31
 |
| Fig. 2 (a) XRD patterns of Mn/SA-BC, nZVI, and Mn/SA-BC@nZVI; (b) FTIR spectra of Mn/SA-BC and Mn/SA-BC@nZVI; (c) pore size distribution (nm) and N2 adsorption–desorption isotherm of nZVI and Mn/SA-BC@nZVI; (d) Mn 2p XPS spectra of Mn/SA-BC and Mn/SA-BC@nZVI; (e) Fe 2p XPS spectra of nZVI and Mn/SA-BC@nZVI; (f) C 1s XPS spectra of Mn/SA-BC and Mn/SA-BC@nZVI; and (g) O 1s XPS spectra of Mn/SA-BC and Mn/SA-BC@nZVI. | |
The Fourier transform infrared spectra (FTIR) of Mn/SA-BC and Mn/SA-BC@nZVI are illustrated in Fig. 2b. The bands at 1088 and 1564 cm−1 in Mn/SA-BC@nZVI were assigned to the C–OH and –COOH stretching vibration,27,32 respectively, which obviously shifted and decreased in intensity compared with that of Mn/SA-BC. According to the obtained result, hydroxyl and carboxyl groups might be involved in the complexation with metal elements. In addition, the characteristic absorption bands of Mn/SA-BC@nZVI at 793 (C–H bending vibration), 1378 (C–H3 bending vibration), and 1580 cm−1 (C
C stretching vibration)33,34 confirmed that aromatic compounds were present on the surface of Mn/SA-BC@nZVI, which was conducive to the adsorption of Cd(II). The bands at 468, 600, and 700 cm−1 were attributed to the Mn–O groups, while the bands at 614 and 880 cm−1 were ascribed to the Fe–O and Fe–OH groups,34,35 respectively, indicating that the Mn and Fe elements were immobilized on the surface of BC. In general, there were abundant functional groups (such as hydroxyl, carboxyl, and metal oxides) in Mn/SA-BC@nZVI.
The N2 adsorption–desorption isotherms of nZVI and Mn/SA-BC@nZVI were analyzed based on the IUPAC classification (Fig. 2c). The isotherm of Mn/SA-BC@nZVI was of type IV shape with a type H3 hysteresis loop, indicating the formation of wedge-shaped mesopores.10 The nZVI in the present study was a macroporous adsorbent with a type II isotherm and type H3 hysteresis loop.6 After the loading of nZVI on the surface or into the micropores of Mn/SA-BC, the specific surface area significantly increased from 4.96 m2 g−1 (nZVI) to 70.70 m2 g−1 (Mn/SA-BC@nZVI), and the average pore size decreased from 14.38 nm (nZVI) to 8.93 nm (Mn/SA-BC@nZVI). It was speculated that the porous graphitized structure of BC provided the attachment sites and enhanced the stability of Mn/SA-BC@nZVI.
The surface chemical states of Mn/SA-BC, nZVI, and Mn/SA-BC@nZVI were characterized by X-ray photoelectron spectroscopy (XPS) (Fig. 2d–g and S2†). In addition, the binding energies at approximately 284.80, 530.70, 641.70, and 711.24 eV were ascribed to C 1s, O 1s, Mn 2p, and Fe 2p in Mn/SA-BC@nZVI, respectively (Fig. S2†). The Mn 2p spectrum of Mn/SA-BC@nZVI was deconvoluted into three peaks at 641.09, 642.25, and 643.45 eV, which denoted Mn(II), Mn(III), and Mn(IV),36 respectively, suggesting the circulation of Mn valence states (Fig. 2d). The peaks of Fe 2p at 706.78 eV were attributed to Fe0. Those located at 710.82 and 723.98 eV were assigned to Fe(II), possibly boosting the generation of hydroxyl radicals as the active sites for electron donation.37 However, the peaks at 712.72 and 725.59 eV belonging to Fe(III) demonstrated the existence of Fe(III) in Mn/SA-BC@nZVI (Fig. 2e), suggesting the oxidation of nZVI to Fe(III) during the synthesis. The Fe(III) content accounted for 39.53% of the total iron, which was conducive to the surface complexation of As(III) and Cd(II). Obviously, the formed Fe(OH)3 might occupy the adsorption site at a higher pH, which hindered the adsorption of As(III) and Cd(II). As illustrated in Fig. 2f, the C 1s peaks at 285.16, 286.20, and 288.96 eV were assigned to C–O–C, C–OH, and C
O/O–C
O, respectively, confirming the abundance of ether bonds, carboxyl groups, and hydroxyl groups in Mn/SA-BC@nZVI.6,7,38 Aromatic organics were also detected from the intensity peak at 284.60 eV, which was attributed to the C–C/C
C bonds and might be beneficial for the electron transfer and chemical stability of Mn/SA-BC@nZVI due to their hydrophobic effects.6 For the O 1s spectrum of Mn/SA-BC@nZVI (Fig. 2g), the peaks at 530.02, 530.62, 531.51, and 532.33 eV were ascribed to Mn–O, O–H, C
O, and C–O, consistent with the result of the XPS C 1s spectrum. The presence of metal oxide bonds in Mn/SA-BC@nZVI benefited the ion exchange and surface complexation of As(III) and Cd(II) in water treatment.24 In summary, abundant functional groups and metallic bonds (i.e., hydroxyl, carboxyl, aromatic organics, and Mn/Fe–O) were successfully functionalized on the surface Mn/SA-BC@nZVI, which might contribute to the complexation and simultaneous adsorption of As(III) and Cd(II).
3.2 Adsorption experiments in single systems
3.2.1 Adsorption kinetics of As(III) and Cd(II).
The adsorption kinetics of As(III) and Cd(II) onto Mn/SA-BC, nZVI, and Mn/SA-BC@nZVI were investigated (Fig. 3 and Table S1†). As shown in Fig. 3a, >90% of As(III) was rapidly adsorbed by Mn/SA-BC@nZVI within 30 min, and subsequently the adsorption equilibrium was achieved in 45 min, indicating the efficient removal of As(III) by Mn/SA-BC@nZVI. Meanwhile, As(V) was clearly observed in the As(III)-treated solution by Mn/SA-BC@nZVI (Fig. 3b) and detected on the solid Mn/SA-BC@nZVI after adsorbing As(III) (Fig. 6e). The above results strongly demonstrated that the partial oxidation of As(III) to As(V) was present in the removal process of As(III), primarily ascribed to the production of free radicals and the valence cycle of Mn/Fe elements. According to Fig. 3c, Mn/SA-BC@nZVI exhibited a highly efficient adsorption property towards Cd(II), and the maximum removal efficiency reached 90.23% within 45 min, possibly because of the abundant functional groups (e.g., hydroxyl, carboxyl, aromatic organics, and Mn/Fe–O) and large specific surface area of Mn/SA-BC@nZVI. Moreover, the pH changes in adsorption kinetics are detected in Fig. S3a.† The pH value of DI water is generally 5.8–6.3. The addition of Mn/SA-BC@nZVI (pH = 6.69) and As(III) (Fig. S3†) exerted a weak effect on the pH of the solution, while a slight drop in the pH value was present with the addition of Cd(II). These results might be interpreted by two aspects. (1) Mn/SA-BC@nZVI contained a large number of functional groups (e.g., hydroxyl, carboxyl, and metal oxides), which could adjust the solution pH with ionization and hydrolysis. (2) The hydrolysis reaction of Cd(II) and As(III) was promoted during the reaction process, and the precipitation of Cd(OH)2 occurred at pH > 8 (Fig. S4†). Both pseudo-first-order and pseudo-second-order models were fitted with the data of adsorption kinetics. However, the pseudo-second-order model had a clearly higher R2 than the pseudo-first-order model (Fig. S5 and S6 and Table S1†), indicating a more suitable fit for the adsorption of As(III) and Cd(II) by employing the pseudo-second-order kinetic model.
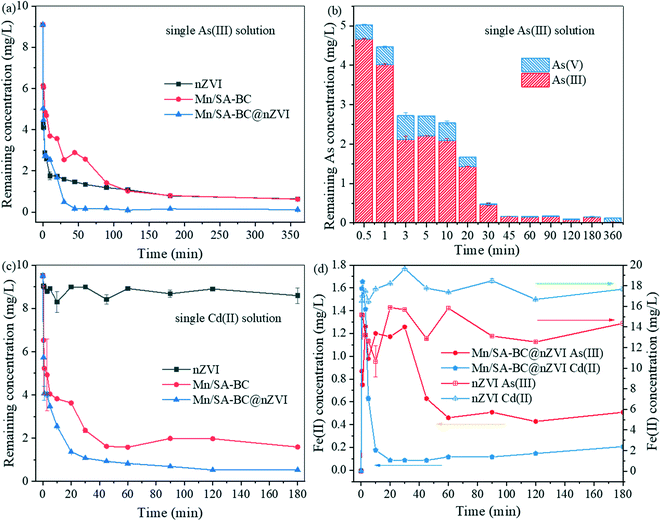 |
| Fig. 3 (a) As(III) adsorption kinetics of Mn/SA-BC, nZVI, and Mn/SA-BC@nZVI, (b) As speciation during kinetic adsorption of As(III) by Mn/SA-BC@nZVI, (c) Cd(II) adsorption kinetics of Mn/SA-BC, nZVI, and Mn/SA-BC@nZVI, and (d) the change of Fe(II) concentration during kinetic adsorption of As(III) and Cd(II) by nZVI and Mn/SA-BC@nZVI. | |
In order to explore the possible removal mechanism of As(III) and Cd(II), the changes in the Fe(II) concentration were investigated for both nZVI and Mn/SA-BC@nZVI systems with As(III) or Cd(II). Based on Fig. 3d, the Fe(II) ion concentration in the As(III) or Cd(II)-treated solution by nZVI or Mn/SA-BC@nZVI rapidly increased within 5 min because of the surface corrosion of nZVI and the production of electrons.39 Subsequently, the Fe(II) concentration of the Mn/SA-BC@nZVI system quickly decreased with the adsorption of As(III) and Cd(II), while it was basically unchanged in the nZVI system, possibly because of the oxidation and capture of Fe(II) ions by free radicals and Mn/SA-BC, respectively. This further promotes the surface complexation, precipitation, and ion exchange of As(III) and Cd(II). More attractively, the release amount of Fe(II) in the Mn/SA-BC@nZVI system was higher than that in the nZVI system without the addition of As(III) and Cd(II), which was primarily attributed to the high graphitization of BC, and this might facilitate the electron transfer of nZVI and MnO (Fig. S7†). When As(III) or Cd(II) was present, the Fe(II) concentration was found to be obviously higher than that in the absence of As(III) and Cd(II). Obviously, the obtained results demonstrated that As(III) and Fe(II) were competing with the free radicals in the adsorption system, primarily due to the low redox potentials of As(V)/As(III) (+0.56 V).40 When Fe(II) entered the solution, Cd(II) might be adsorbed by the vacant active site with electrostatic attraction, possibly because of the complexation with the produced carboxyl groups and hydroxyl groups based on the principle of hard and soft acids and bases.10
3.2.2 Adsorption isotherms of As(III) and Cd(II).
The adsorption isotherms of As(III) and Cd(II) on Mn/SA-BC@nZVI at 25 °C were modeled by the Langmuir and Freundlich models (Fig. S8 and S9, and Table S2†). Both the Langmuir and Freundlich models analyzed the experimental data; however, the Freundlich model had a higher R2, suggesting that the Freundlich model better described the adsorption process of As(III) and Cd(II). The results demonstrated the multilayer adsorption of As(III) and Cd(II) onto Mn/SA-BC@nZVI. Meanwhile, the pH value of As(III) solution increased with the added concentration, but the opposite was true in Cd(II) solution (Fig. S3b†), possibly because of the hydrolysis of As(III) and Cd(II). Considering that the data were well fitted with the Langmuir model (R2 = 0.990–0.991), the values of Langmuir maximum capacity were used to compare the adsorption capacity of Mn/SA-BC@nZVI for As(III) and Cd(II) with reported adsorbents (Table S3†). Mn/SA-BC@nZVI showed a higher adsorption potential towards As(III) and Cd(II), with maximum capacities of 121 and 120 mg g−1, respectively, which surpassed the removal capacities of most of the reported adsorbents. These results indicated that Mn/SA-BC@nZVI displayed promising potential for the removal of As(III) and Cd(II) from real wastewater.
3.3 Simultaneous adsorption experiments in binary systems
3.3.1 Synergistic adsorption of As(III) and Cd(II).
Considering the frequent coexistence of As(III) and Cd(II) in water and soil, the synergistic adsorption property of Mn/SA-BC@nZVI was measured using different concentration ratios of As(III) to Cd(II) (i.e., As
:
Cd = 1
:
1, 1
:
2, 1
:
3, 2
:
1, 2
:
2, 2
:
3, 3
:
1, 3
:
2, and 3
:
3). As depicted in Fig. 4a and b, Mn/SA-BC@nZVI synergistically adsorbed As(III) and Cd(II) under the above ratios. Interestingly, there was no direct correlation or reaction between As(III) and Cd(II), while the adsorption efficiency of As(III) and Cd(II) was noticeably enhanced by Mn/SA-BC@nZVI with the addition of Cd(II) or As(III), respectively (Fig. 4a and b). When the initial concentration of As(III) was 20 mg L−1, the adsorption efficiency of As(III) increased from 87.83% to 98.16% with the increase in Cd(II) concentration from 0 to 30 mg L−1 (Fig. 4a). Correspondingly, the removal efficiency of Cd(II) was strengthened from 63.90% to 95.13% by increasing the coexisting As(III) concentration from 0 to 30 mg L−1 (Fig. 4b). Meanwhile, the simultaneous adsorption kinetics of As(III) and Cd(II) on Mn/SA-BC@nZVI were investigated using the initial As(III) and Cd(II) concentration of 20 mg L−1. As shown in Fig. 4c and d, >96% of As(III) and >85% of Cd(II) were rapidly and simultaneously removed by Mn/SA-BC@nZVI within 20 min, and the removal efficiency of the mixed solution was obviously higher than the single As(III) (85.18%) or the single Cd(II) (58.35%) solution within 20 min. The results of pseudo-second-order model fitting were quite close to the experimental data (Fig. S10 and S11 and Table S1†). These results indicated that Mn/SA-BC@nZVI was an ideal adsorbent for the simultaneous adsorption of As(III) and Cd(II) due to the formation of ternary anion-bridged (MnO/FeO–As–Cd) and cation-bridged surface complexes (MnO/FeO–Cd–As).41 As accelerated the adsorption of Cd(II) by forming the Mn/Fe–O–As bridging molecule on the surface of Mn/SA-BC@nZVI, which may be related to the enhancement of surface precipitation, electrostatic attraction, multiple H-bonds, and ternary surface complexation.42,43 Moreover, the Cd(II) adsorption of Mn/SA-BC@nZVI led to the neutralization of negative charges and compression of the electrostatic layer on the surface of BC, which reduced the electronic repulsion between BC and As(III). This result provided further evidence that the ternary surface complexes were beneficial for the synergistic and efficient adsorption of anions and cations by constructing A (metal surface) and B (ligand surface) types.42,44 Interestingly, As(V) was significantly detected in both the single and mixed-treated solution by Mn/SA-BC@nZVI, and the content of As(V) was higher in the single As-treated solution than the mixed solution (Fig. 4e and f). This result suggested that surface complexation and oxidative detoxification were present simultaneously in the As(III) removal process of Mn/SA-BC@nZVI, but ternary surface complexation might facilitate the rapid adsorption of As(III) and Cd(II) by Mn/SA-BC@nZVI in the mixed solution.
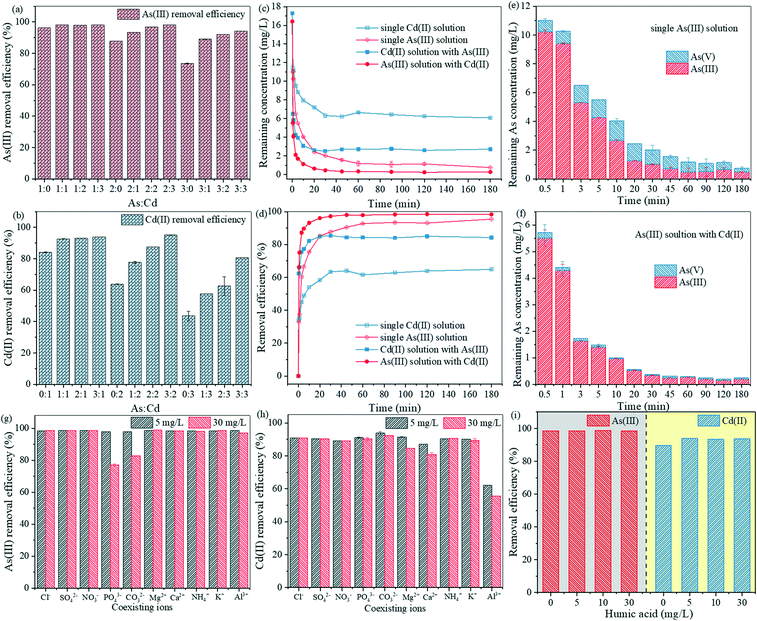 |
| Fig. 4 Removal efficiency of mixed (a) As(III) and (b) Cd(II) solutions with initial As(III) and Cd(II) concentrations of 0, 10, 20, or 30 mg L−1; (c) adsorption kinetics and (d) removal efficiency of Mn/SA-BC@nZVI in collaborative adsorption experiments with initial As(III) and Cd(II) concentrations of 20 mg L−1. As speciation during kinetic adsorption of (e) single and (f) mixed solutions; and effects of coexisting ions (Cl−, SO42−, NO3−, PO43−, CO32−, Mg2+, Ca2+, NH4+, K+, or Al3+) on the simultaneous adsorption of (g) As(III) and (h) Cd(II) by Mn/SA-BC@nZVI and (i) humic acid on the synergistic adsorption of As(III) and Cd(II) by Mn/SA-BC@nZVI. | |
3.3.2 Effects of coexisting ions and pH on the simultaneous adsorption of As(III) and Cd(II).
Generally, the simultaneous adsorption of As(III) and Cd(II) onto Mn/SA-BC@nZVI was affected by the coexisting anions (Cl−, SO42−, NO3−, PO43−, and CO32−) and cations (Mg2+, Ca2+, NH4+, K+, and Al3+) (Fig. 4g and h). PO43− exerted the most obvious distracting effect on the removal process of As(III) because of the competitive adsorption between As(III) and PO43−, which possessed similar chemical structures.45 The influence of CO32− might be primarily ascribed to its higher negative charge density (Fig. 4g). Furthermore, the coexisting cations mainly affected the Cd(II) removal of Mn/SA-BC@nZVI in the sequence of Al3+ > Ca2+ > Mg2+ > other coexisting ions (Fig. 4h). Al3+, Ca2+, and Mg2+ reduced the negative charge density of Mn/SA-BC@nZVI or competed for the active sites with Cd(II) due to the high positive charge density. According to the result, the electrostatic attraction played an important role in the Cd(II) adsorption process of Mn/SA-BC@nZVI. As shown in Fig. 4i, humic acid exerted no significant effect on the simultaneous adsorption of As(III) and Cd(II), possibly because of mutual repulsion between the electron-rich humic acid and Mn/SA-BC@nZVI.46 Fig. S12† exhibits the effect of pH on the synergistic adsorption of As(III) and Cd(II) by Mn/SA-BC@nZVI. The As(III) adsorption efficiency of Mn/SA-BC@nZVI in the mixed solution was maintained at above 98% at pH below 9. When the pH ranged from 2 to 9, the As(III) species in the mixed solution was primarily H3AsO3 due to its pKa = 9.2 (eqn (1)), which was conducive to the occurrence of surface complexation with an As–O–Mn/Fe bond. The association between As(III) and FeOOH/MnOOH increased with increasing pH (eqn (2)). Obviously, the As(III) removal efficiency was reduced to 84.56% with increasing pH from 9.0 to 10.0, primarily attributed to the formation of Fe(OH)3, H2AsO3−, and HAsO32− (eqn (3) and (4)), which may override the adsorption site and reinforce the electrostatic repulsion of As(III) and Mn/SA-BC@nZVI.46,47 Similarly, the amounts of removed Cd(II) in the mixed solution increased with increasing pH from 2.0 to 6.0 (Fig. S12†), while a slight decrease was observed at pH = 10. The results were ascribed to the positively charged surfaces and protonation of Mn/SA-BC@nZVI at a lower pH, hindering the adsorption of Cd(II) on the surface of Mn/SA-BC@nZVI because of electrostatic repulsion. With the continuous increase in pH to 7, deprotonation occurred on the adsorption sites of Mn/SA-BC@nZVI, which was beneficial for the complexation between adsorbents and Cd(II). When the pH was greater than 8, large amounts of Cd(OH)2 precipitates were produced (Fig. S4†), suggesting that the precipitation was conducive to the removal of Cd(II). | [H3AsO3]0 ↔ [H2AsO3]−![[thin space (1/6-em)]](https://www.rsc.org/images/entities/char_2009.gif) pKa = 9.2 | (1) |
| FeOOH/MnOOH + [H3AsO3]0 → Fe/Mn–O–[H2AsO3] + H2O | (2) |
| [H3AsO3]0 + OH− → [H2AsO3]− + H2O | (3) |
| [H2AsO3]− + OH− → [HAsO3]2− + H2O | (4) |
3.4 Reusability of Mn/SA-BC@nZVI
As shown in Fig. S13,† the reusability of Mn/SA-BC@nZVI for 5 cycles of As(III) adsorption was investigated. After adsorbing As(III), Mn/SA-BC@nZVI was regenerated by the NaOH extraction method. That is, the adsorbent was immersed with 0.5 mol L−1 NaOH for 12 h to desorb As(III). Then, the recovered adsorbent was lyophilized at −48 °C for 5 h for the next cycle of adsorption–regeneration. The removal efficiency of As(III) showed a large decrease from the first to the second cycle, possibly because of the undesorbed As(III) on Mn/SA-BC@nZVI. However, the removal efficiency of As(III) still exceeded 65% of the initial maximum in subsequent cycles because of the strong elution of NaOH. Mn/SA-BC@nZVI showed high renewability and reusability when adsorbing As(III), which is a promising adsorbent for wastewater treatment.
3.5 Characterization of Mn/SA-BC@nZVI after adsorbing As(III) and Cd(II)
Fig. 5 and S14† show the SEM images and SEM-EDS results of Mn/SA-BC@nZVI after adsorbing As(III) and Cd(II). The surface square granular nZVI completely disappeared after adsorbing As(III) and Cd(II), indicating that complexation and oxidation reaction participated in the removal of As(III) and Cd(II). It was noteworthy that the As and Cd peaks were clearly detected in the EDS spectra, and there were relevant solid particulates on the surface of Mn/SA-BC@nZVI, providing further evidence that the surface complexation of Fe/Mn elements was an important adsorption mechanism of Mn/SA-BC@nZVI for As(III) and Cd(II).
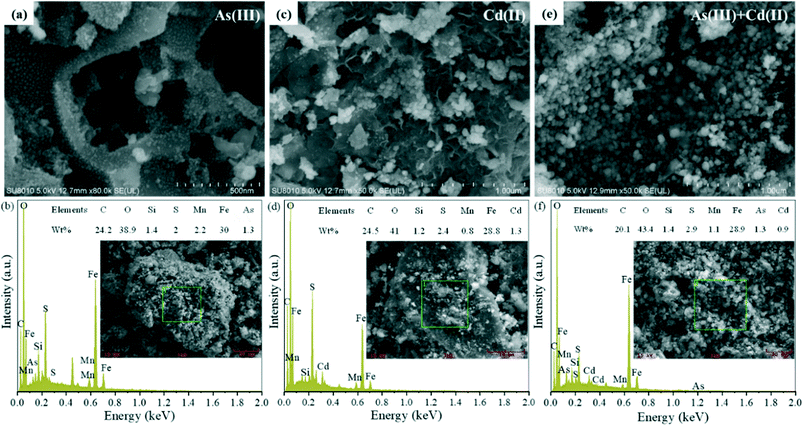 |
| Fig. 5 SEM images and SEM-EDS results of the samples after Mn/SA-BC@nZVI adsorption of (a) and (b) As(III), (c) and (d) Cd(II), and (e) and (f) As(III) and Cd(II), respectively. | |
The XRD patterns of nZVI and Mn/SA-BC@nZVI before and after adsorbing As(III) and Cd(II) are shown in Fig. S15† and 6a, respectively. Compared with Mn/SA-BC@nZVI, several new reflections for MnAsO4 and FeAsO4 were detected on Mn/SA-BC@nZVI after adsorbing As(III), suggesting that surface complexation and oxidation reaction occurred during the As(III) adsorption process. Similarly, CdFe2O4 and CdMn2O4 were formed on the surface of Mn/SA-BC@nZVI after adsorbing Cd(II), which was also attributed to the surface complexation with manganese and iron oxyhydroxide minerals. Significantly, the sharp peaks at 2θ = 34.30°, 44.68°, 49.29°, 65.03°, and 82.50° disappeared after adsorbing As(III) and Cd(II),30 probably ascribed to the formation of As2S3 and CdS by surface precipitation, as well as FeAsO4 and CdFe2O4 by surface complexation with metal oxides. However, the crystals of As2S3 and CdS were undetected in Mn/SA-BC@nZVI after the adsorption of As(III) and Cd(II), which was due to its low content and the production of encapsulated As2S3 and CdS by the manganese and iron complex.48,49 In conclusion, the results obviously illustrated that the major adsorption mechanism of As(III) and Cd(II) by Mn/SA-BC@nZVI occurred via surface complexation with manganese and iron oxyhydroxides.
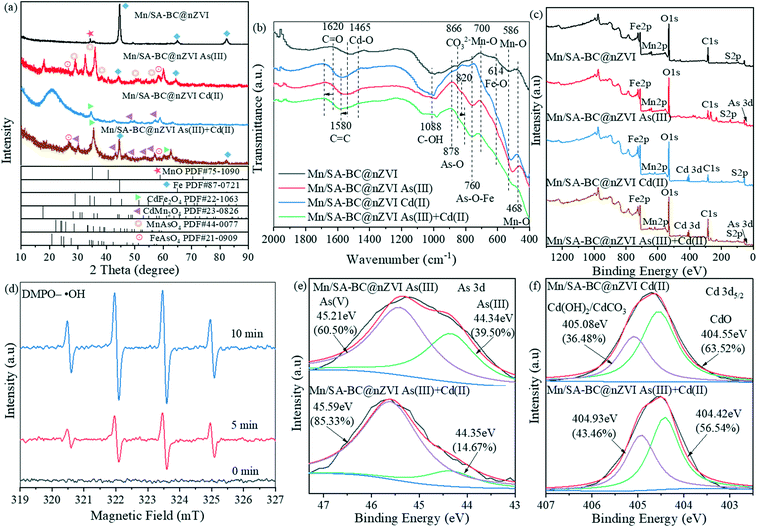 |
| Fig. 6 (a) XRD patterns, (b) FTIR spectra, and (c) wide-scan survey XPS spectra of Mn/SA-BC@nZVI before and after the adsorption of As(III) and Cd(II); (d) ESR spectra of DMPO–˙OH in the Mn/SA-BC@nZVI reaction system without the addition of As(III) and Cd(II); XPS spectra of (e) As 3d and (f) Cd 3d5/2 of Mn/SA-BC@nZVI after the adsorption of As(III) and Cd(II). | |
The FTIR spectra of Mn/SA-BC@nZVI before and after adsorbing As(III) and Cd(II) are shown in Fig. 6b. The bands at 1580 and 1620 cm−1 corresponding to the C
C and C
O stretching vibrations exhibited a remarkable shift to higher wavelengths after adsorbing As(III) and Cd(II), respectively, implying that As(III) and Cd(II) were bound to Mn/SA-BC@nZVI through coordination of those surface functional groups such as Cd–π with a C
C bond and mono- or multi-dentate inner-sphere complexes with the carboxyl.24,50 The strong electron-rich domains of C
C can enhance the Cd–π interaction, which will promote the adsorption of Cd(II). When compared with Mn/SA-BC@nZVI, the band at 866 cm−1 (CO32−) disappeared after adsorbing Cd(II), possibly due to precipitation with CO32−.24 The peaks of Mn–O and Fe–O bands of Mn/SA-BC@nZVI were weakened after the adsorption of As(III) and Cd(II), further confirming the significant contribution of metal oxides to the complexation of As(III) and Cd(II). The newly generated bands at 760 and 878 cm−1 were attributed to As–O–Fe and As–O stretching vibrations after adsorbing As(III), which was ascribed to the occurrence of bidentate binuclear complexation with Mn/SA-BC@nZVI.51 Apart from that, a remarkable bathochromic shift of the As–O vibration was observed from 820 to 808 cm−1 after the synergistic adsorption of As(III) and Cd(II), indicating the formation of ternary surface complexes (MnO/FeO–Cd–As and MnO/FeO–As–Cd).52
To further investigate the simultaneous removal mechanisms of As(III) and Cd(II) using Mn/SA-BC@nZVI, the XPS spectra of Mn/SA-BC@nZVI before and after adsorbing As(III) and Cd(II) were analyzed (Fig. 6c, e and f, and S16†). Noteworthily, novel adsorption peaks for As 3d (52.28 eV) and Cd 3d (422.48 eV) appeared in the XPS spectra of Mn/SA-BC@nZVI after the adsorption of As(III) and Cd(II), respectively (Fig. 6c).24,53 The high-resolution As 3d spectra of Mn/SA-BC@nZVI were deconvoluted into two peaks at binding energies of 44.34 and 45.21 eV after adsorbing As(III), which could be assigned to As(III) and As(V), respectively (Fig. 6e).5 Interestingly, the peak area ratio of As(V) after simultaneous adsorption of As(III) and Cd(II) (85.33%) was higher than that of the single adsorption of As(III) (60.50%), indicating that the oxidation of As(III) could be promoted by the formation of ternary surface complexes in a synergetic adsorption system. Nevertheless, the remaining As species were primarily As(III) in the single and mixed solutions (Fig. 4e and f), and the residual concentration of As in As(V)-contaminated solutions was significantly lower than that in As(III)-polluted solutions within 0–30 min (Fig. S17†). The results indicated that As(V) might be adsorbed more efficiently and quickly than As(III) due to the formation of more H-bonds with the oxygen atoms in As(V) oxoanions and stronger electrostatic attraction between As(V) and Mn/Fe clusters of Mn/SA-BC@nZVI. Meanwhile, it was noteworthy that nZVI and MnO of Mn/SA-BC@nZVI might react with H2O and dissolved oxygen to produce free radicals. The ESR results displayed the typical signals of DMPO–˙OH without the addition of As(III) and Cd(II) (Fig. 6d and Table S4†). This result reveals the production of hydroxyl radicals, which might promote the oxidation of As(III) and valence cycle of Mn/Fe. The Cd 3d5/2 peaks at 404.55 and 405.08 eV represented the CdO and Cd(OH)2/CdCO3 of Mn/SA-BC@nZVI after adsorbing Cd(II) (Fig. 6f),54 respectively, possibly attributed to the chelation and precipitation, which was in accordance with the observation of microspherical particles in the SEM image (Fig. 5).
The abundant oxygen-containing groups (e.g., Mn–O, Fe–O, C–O–C, C–OH, and C
O/O–C
O) were uniformly distributed on the surface of Mn/SA-BC@nZVI, which efficiently removed As(III) and Cd(II) from the aquatic environment (Fig. S16†). After adsorbing As(III) and Cd(II), the XPS peaks of Mn 2p (Fig. S16a†) and Fe 2p (Fig. S16b†) on Mn/SA-BC@nZVI displayed significant changes, proving that both the Mn–O and Fe–O groups were involved in the adsorption of As(III) and Cd(II) from the aqueous solution.27 Attractively, the peak area ratio of Mn(II) reduced from 39.60% to 26.09% after the adsorption of Cd(II) by Mn/SA-BC@nZVI, indicating the occurrence of surface complexation and ion exchange between Mn oxide and Cd(II). The binding energy of C–OH groups significantly increased from 286.20 to 286.50, 286.52, and 286.55 eV after the adsorption of As(III) and Cd(II) in the single and mixed solutions, respectively (Fig. S16c†). This is possibly due to the formation of the Cd–π bond with C–OH groups that lowered the electron cloud density of the carbon atoms in C–O. Moreover, the peak area ratio of C–O–C groups decreased from 35.55% to 28.00%, 30.71%, and 31.16% after adsorption of As(III) and Cd(II) in the single and mixed solutions. Similarly, the peak area ratio of Fe/Mn–O groups reduced from 22.31% to 21.63%, 19.70%, and 18.99% after adsorption of As(III) and Cd(II) in the single and mixed solutions (Fig. S16d†). These results strongly demonstrated that the highly toxic anions and cations were anchored onto the surface of Mn/SA-BC@nZVI by numerous oxygen-containing groups.
3.6 Adsorption mechanisms of As(III) or Cd(II) by Mn/SA-BC@nZVI
The adsorption mechanisms of As(III) and Cd(II) by Mn/SA-BC@nZVI are illustrated in Scheme 2a. During the adsorption, numerous electrons were released by the rapid oxidation of nZVI (eqn (5)). Then they were rapidly transferred to the dissolved oxygen and H2O by highly graphitized BC. Large amounts of H2O2 would be produced during this process (eqn (6)).55 Next, H2O2 was catalyzed by Fe(II)/Mn(II) ions to generate ˙OH on the surface of Mn/SA-BC@nZVI (eqn (7) and (8)).56,57 Consequently, the valence cycle of Fe/Mn was accelerated with the generation of electrons and ˙OH (eqn (9) and (10)), facilitating the oxidation and detoxification of As(III) (eqn 11).39 The removal of As(III) was mainly attributed to the formation of the inner-sphere bidentate-mononuclear complexes with Mn–O and Fe–O groups (eqn (12)).58 The fitted XPS peaks of Mn/SA-BC@nZVI after adsorbing As(III) displayed that the area ratios of As(III) and As(V) were approximately 39.50 and 60.50% (Fig. 6e), respectively, suggesting that the oxidation and inner-sphere surface complexation mechanisms simultaneously existed in As(III) removal by Mn/SA-BC@nZVI (eqn 11–12). Nevertheless, the residual concentration of As(V) in As(III)-contaminated solutions (Fig. 4e and f) was obviously lower than that of As(III), possibly attributed to the competitive advantages of As(V) in the adsorption process via more H-bonds and stronger electrostatic attraction.59,60 Similarly, the predominant removal mechanism of Cd(II) by Mn/SA-BC@nZVI referred to surface complexation with oxygen-containing groups, such as metal oxides (Mn–O and Fe–O) and soft oxygenated functional groups (e.g., C–O–C, C–OH, and C
O/O–C
O) (eqn (13)).61 In addition, ion exchanges easily occurred between Cd(II) and Fe(II) from nZVI oxidation, as supported by the results of Fe(II) concentration in solution (eqn (14)) (Fig. 3d and S7†). Moreover, part of Cd(II) was also adsorbed through the Cd–π bond with aromatic carbon components and precipitation with CO32− (eqn (15)).62 | O2 + 2H2O + 2e− → 2H2O2 | (6) |
| H2O2 + Fe(II)/Mn(II) → OH− + ˙OH + Fe(III)/Mn(III) | (8) |
| Mn(II)/Fe(II) + ˙OH → Mn(III)/Mn(IV)/Fe(III) | (9) |
| Mn(III)/Mn(IV)/Fe(III) + e− → Mn(II)/Fe(II) | (10) |
| As(III) + ˙OH/Mn(IV)/Mn(III)/Fe(III) → As(V) | (11) |
| As(III)/As(V) + Mn/SA-BC@nZVI → As–O–Mn/Fe | (12) |
| Cd(II) + RCOO− → RCOO–Cd | (13) |
| Mn/SA-BC@nZVI + Cd(II) → Mn/SA-BC–O–Cd + Fe(II) | (14) |
| Cd(II) + CO32− → CdCO32− | (15) |
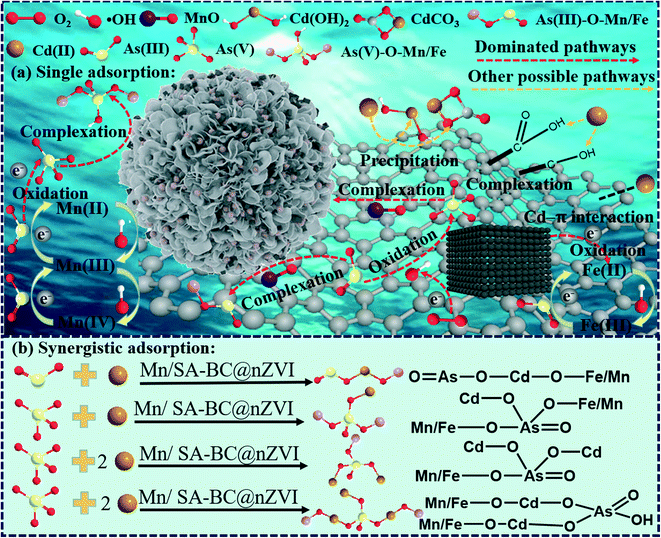 |
| Scheme 2 Schematic diagram of the removal mechanisms in (a) single and (b) synergistic adsorption of As(III) and Cd(II) by Mn/SA-BC@nZVI. | |
3.7 Simultaneous adsorption mechanisms of As(III) and Cd(II) by Mn/SA-BC@nZVI
The synergistic adsorption mechanisms in the mixed solution of As(III) and Cd(II) by Mn/SA-BC@nZVI are illustrated in Scheme 2b. The simultaneous removal of As(III) and Cd(II) by Mn/SA-BC@nZVI was mainly ascribed to the formation of cation-bridged surface complexes (MnO/FeO–Cd–As) and anion-bridged surface complexes (MnO/FeO–As–Cd) by the bidentate edge-sharing and bidentate corner-sharing complexes.42,63 A point worth emphasizing is that the ligand exchange reactions and precipitation of ternary surface complexes (MnO/FeO–Cd–As and MnO/FeO–As–Cd) might partially contribute to the binding of As(III) and Cd(II) on the surface of Mn/SA-BC@nZVI.64 In addition, the oxidation–adsorption ratio of As(III) on Mn/SA-BC@nZVI obviously increased after the synergistic adsorption of As(III) and Cd(II) compared with single adsorption of As(III) (Fig. 6e), suggesting that the oxidation–complexation of As(III) was facilitated by Mn/SA-BC@nZVI with the presence of Cd(II), possibly because of the rapid complexation between binary complexes (MnO/FeO–Cd) and As(V) via electrostatic attraction and hydrogen bonding. The As(V) concentration of single As(III)-treated solution was significantly higher than that of the mixed solution of As(III) and Cd(II) (Fig. 4e and f), providing further evidence that binary complexes (MnO/FeO–Cd) might stimulate the capture of As(III) through oxidation–complexation. More attractively, the anion-bridged surface complexes (e.g., MnO/FeO–As, MnO/FeO–As–Cd, and MnO/FeO–As–Cd–As) and cation-bridged surface complexes (e.g., MnO/FeO–Cd, MnO/FeO–Cd–As, and MnO/FeO–Cd–As–Cd) served as the persistent adsorption sites to immobilize the highly toxic anions and cations by surface multiple complexation, electrostatic attraction, multiple H-bonds, and precipitation.
4. Conclusion
To conclude, a new Mn/SA-BC@nZVI material was fabricated for the simultaneous removal of As(III) and Cd(II) from contaminated water. Mn/SA-BC@nZVI had a high graphitization degree, favorable specific surface area, and abundant oxygen-containing groups. The adsorption of As(III) and Cd(II) was well fitted with the Freundlich and Langmuir models. The maximum adsorption capacities of As(III) and Cd(II) were 121 mg g−1 and 120 mg g−1, respectively. A strong synergistic effect between nZVI and Mn/SA-BC was demonstrated to enhance the removal of As(III) and Cd(II). The carbon-rich graphitized structure of Mn/SA-BC promoted electron transfer from nZVI to H2O and dissolved oxygen, which was beneficial for the production of hydroxyl radicals. The dominant removal mechanisms of As(III) were oxidation and inner-sphere surface complexation, while those of Cd(II) were Cd–π binding with aromatic carbon components, surface complexation with Mn–O, Fe–O, C–O–C, C–OH, and C
O/O–C
O groups, and precipitation with CO32−. More importantly, in the mixed solution of As(III) and Cd(II), the removal efficiencies of both As(III) and Cd(II) by Mn/SA-BC@nZVI were significantly strengthened, which was primarily ascribed to the generation of anion-bridged and cation-bridged surface complexes via surface multiple complexation, electrostatic attraction, multiple H-bonds, and precipitation.
Author contributions
Wei Mao: writing – original draft. Lixun Zhang: writing – review & editing. Ying Zhang: investigation. Yuntao Guan: funding acquisition, writing – review & editing.
Conflicts of interest
There are no conflicts to declare.
Acknowledgements
The current work was supported by the National Natural Science Foundation of China [No. 51979141]; Key Research and Development program of Guangdong Province [No. 2019B110205003]; Shenzhen Science and Technology Research Project [No. JSGG20191129094410446]; and Key Project of institutions of higher learning in Shenzhen [No. WDZC20200821090937001].
Notes and references
- M. Luo, H. Lin, Y. He, B. Li, Y. Dong and L. Wang, Efficient simultaneous removal of cadmium and arsenic in aqueous solution by titanium-modified ultrasonic biochar, Bioresour. Technol., 2019, 284, 333–339 CrossRef PubMed.
- C. Shan, Y. Liu, Y. Huang and B. Pan, Non-radical pathway dominated catalytic oxidation of As(III) with stoichiometric H2O2 over nanoceria, Environ. Int., 2019, 124, 393–399 CrossRef PubMed.
- J. Wu, Z. Li, D. Huang, X. Liu, C. Tang, S. J. Parikh and J. Xu, A novel calcium-based magnetic biochar is effective in stabilization of arsenic and cadmium co-contamination in aerobic soils, J. Hazard. Mater., 2020, 387, 122010 CrossRef PubMed.
- J. Wu, D. Huang, X. Liu, J. Meng, C. Tang and J. Xu, Remediation of As(III) and Cd(II) co-contamination and its mechanism in aqueous systems by a novel calcium-based magnetic biochar, J. Hazard. Mater., 2018, 348, 10–19 CrossRef PubMed.
- L. Ma, D. Cai and S. Tu, Arsenite simultaneous sorption and oxidation by natural ferruginous manganese ores with various ratios of Mn/Fe, Chem. Eng. J., 2020, 382, 123040 CrossRef.
- L. Zhang, S. Tang, F. He, Y. Liu, W. Mao and Y. Guan, Highly efficient and selective capture of heavy metals by poly(acrylic acid) grafted chitosan and biochar composite for wastewater treatment, Chem. Eng. J., 2019, 378, 122215 CrossRef.
- T. Wang, S. Liu, W. Mao, Y. Bai, K. Chiang, K. Shah and J. Paz-Ferreiro, Novel Bi2WO6 loaded N-biochar composites with enhanced photocatalytic degradation of rhodamine B and Cr(VI), J. Hazard. Mater., 2020, 389, 121827 CrossRef PubMed.
- Y.-D. Chen, X. Duan, C. Zhang, S. Wang, N.-Q. Ren and S.-H. Ho, Graphitic biochar catalysts from anaerobic digestion sludge for nonradical degradation of micropollutants and disinfection, Chem. Eng. J., 2020, 384, 123244 CrossRef.
- L. Xiang, C.-G. Niu, N. Tang, X.-X. Lv, H. Guo, Z.-W. Li, H.-Y. Liu, L.-S. Lin, Y.-Y. Yang and C. Liang, Polypyrrole coated molybdenum disulfide composites as adsorbent for enhanced removal of Cr(VI) in aqueous solutions by adsorption combined with reduction, Chem. Eng. J., 2021, 408, 127281 CrossRef.
- L. Zhang, F. He, W. Mao and Y. Guan, Fast and efficient removal of Cr(VI) to ppb level together with Cr(III) sequestration in water using layered double hydroxide interclated with diethyldithiocarbamate, Sci. Total Environ., 2020, 727, 138701 CrossRef PubMed.
- H. Niu, Y. Zheng, S. Wang, S. He and Y. Cai, Stable hierarchical microspheres of 1D Fe–gallic acid MOFs for fast and efficient Cr(vi) elimination by a combination of reduction, metal substitution and coprecipitation, J. Mater. Chem. A, 2017, 5, 16600–16604 RSC.
- T. K. Das, T. S. Sakthivel, A. Jeyaranjan, S. Seal and A. N. Bezbaruah, Ultra-high arsenic adsorption by graphene oxide iron nanohybrid: Removal mechanisms and potential applications, Chemosphere, 2020, 253, 126702 CrossRef PubMed.
- L. Wang, D. W. Cho, D. C. W. Tsang, X. Cao, D. Hou, Z. Shen, D. S. Alessi, Y. S. Ok and C. S. Poon, Green remediation of As and Pb contaminated soil using cement-free clay-based stabilization/solidification, Environ. Int., 2019, 126, 336–345 CrossRef PubMed.
- Q. Yu, L. Chen, W. Wang, Q. Wang, R. Bai, X. Zhuang, Y. Guo, W. Qi and Z. Yuan, Impact of blending on hydrolysis and ethanol fermentation of garden wastes, J. Cleaner Prod., 2018, 190, 36–43 CrossRef.
- W. Mao, L. Zhang, Y. Liu, T. Wang, Y. Bai and Y. Guan, Facile assembled N, S-codoped corn straw biochar loaded Bi2WO6 with the enhanced electron-rich feature for the efficient photocatalytic removal of ciprofloxacin and Cr(VI), Chemosphere, 2021, 263, 127988 CrossRef PubMed.
- Z. Ahmad, B. Gao, A. Mosa, H. Yu, X. Yin, A. Bashir, H. Ghoveisi and S. Wang, Removal of Cu(II), Cd(II) and Pb(II) ions from aqueous solutions by biochars derived from potassium-rich biomass, J. Cleaner Prod., 2018, 180, 437–449 CrossRef.
- X. Dong, L. Q. Ma, Y. Zhu, Y. Li and B. Gu, Mechanistic investigation of mercury sorption by Brazilian pepper biochars of different pyrolytic temperatures based on X-ray photoelectron spectroscopy and flow calorimetry, Environ. Sci. Technol., 2013, 47, 12156–12164 CrossRef PubMed.
- Y. Zhou, B. Gao, A. R. Zimmerman, H. Chen, M. Zhang and X. Cao, Biochar-supported zerovalent iron for removal of various contaminants from aqueous solutions, Bioresour. Technol., 2014, 152, 538–542 CrossRef PubMed.
- J. Chen, X. Yu, C. Li, X. Tang and Y. Sun, Removal of tetracycline via the synergistic effect of biochar adsorption and enhanced activation of persulfate, Chem. Eng. J., 2020, 382, 122916 CrossRef.
- J. D. Vernon and J. C. Bonzongo, Volatilization and sorption of dissolved mercury by metallic iron of different particle sizes: implications for treatment of mercury contaminated water effluents, J. Hazard. Mater., 2014, 276, 408–414 CrossRef PubMed.
- G. H. Qasim, S. Lee, G. Lee, W. Lee, Y. Hong and S. Han, Dissolved oxygen and nitrate effects on the reduction and removal of divalent mercury by pumice supported nanoscale zero-valent iron, Environ. Sci.: Water Res. Technol., 2018, 4, 1651–1661 RSC.
- J. Sun, J. Yang, Y. Liu, M. Guo, Q. Wen, W. Sun, J. Yao, Y. Li and F. Jiang, Magnetically-mediated regeneration and reuse of core-shell Fe(0)@Fe(III) granules for in-situ hydrogen sulfide control in the river sediments, Water Res., 2019, 157, 621–629 CrossRef PubMed.
- M. J. Asay, S. P. Fisher, S. E. Lee, F. S. Tham, D. Borchardt and V. Lavallo, Synthesis of unsymmetrical N-carboranyl NHCs: directing effect of the carborane anion, Chem. Commun., 2015, 51, 5359–5362 RSC.
- J. Xiao, R. Hu, G. Chen and B. Xing, Facile synthesis of multifunctional bone biochar composites decorated with Fe/Mn oxide micro-nanoparticles: Physicochemical properties, heavy metals sorption behavior and mechanism, J. Hazard. Mater., 2020, 399, 123067 CrossRef CAS PubMed.
- E. Leiva, C. Tapia and C. Rodriguez, Removal of Mn(II) from Acidic Wastewaters Using Graphene Oxide-ZnO Nanocomposites, Molecules, 2021, 26, 2713 CrossRef CAS PubMed.
- E. Grygo-Szymanko, A. Tobiasz and S. Walas, Speciation analysis and fractionation of manganese: A review, TrAC, Trends Anal. Chem., 2016, 80, 112–124 CrossRef CAS.
- G. Yin, X. Song, L. Tao, B. Sarkar, A. K. Sarmah, W. Zhang, Q. Lin, R. Xiao, Q. Liu and H. Wang, Novel Fe-Mn binary oxide-biochar as an adsorbent for removing Cd(II) from aqueous solutions, Chem. Eng. J., 2020, 389, 124465 CrossRef CAS.
- W. Zhang, J. Sheng, J. Zhang, T. He, L. Hu, R. Wang, L. Mai and S. Mu, Hierarchical three-dimensional MnO nanorods/carbon anodes for ultralong-life lithium-ion batteries, J. Mater. Chem. A, 2016, 4, 16936–16945 RSC.
- R. B. Pujari, A. C. Lokhande, A. R. Shelke, S. B. Kale, D.-W. Lee and C. D. Lokhande, MnS2/carbon nanotube electrode for improved supercapacitor performance, Solid State Sci., 2021, 111, 106449 CrossRef CAS.
- Y. Ma, X. Lv, D. Xiong, X. Zhao and Z. Zhang, Catalytic degradation of ranitidine using novel magnetic Ti3C2-based MXene nanosheets modified with nanoscale zero-valent iron particles, Appl. Catal., B, 2021, 284, 119720 CrossRef CAS.
- C. Liu, L. Chen, D. Ding and T. Cai, From rice straw to magnetically recoverable nitrogen doped biochar: Efficient activation of peroxymonosulfate for the degradation of metolachlor, Appl. Catal., B, 2019, 254, 312–320 CrossRef CAS.
- J. Zhang, Q. Zhu and Z. Xing, Preparation of new materials by ethylene glycol modification and Al(OH)3 coating NZVI to remove sulfides in water, J. Hazard. Mater., 2020, 390, 122049 CrossRef CAS PubMed.
- J. Lu, Y. Yang, P. Liu, Y. Li, F. Huang, L. Zeng, Y. Liang, S. Li and B. Hou, Iron-montmorillonite treated corn straw biochar: Interfacial chemical behavior and stability, Sci. Total Environ., 2020, 708, 134773 CrossRef CAS PubMed.
- L. Wu, S. Zhang, J. Wang and X. Ding, Phosphorus retention using iron (II/III) modified biochar in saline-alkaline soils: Adsorption, column and field tests, Environ. Pollut., 2020, 261, 114223 CrossRef CAS PubMed.
- J. Liu, A. Liu and W.-x. Zhang, The influence of polyelectrolyte modification on nanoscale zero-valent iron (nZVI): Aggregation, sedimentation, and reactivity with Ni(II) in water, Chem. Eng. J., 2016, 303, 268–274 CrossRef CAS.
- T. Xiong, X. Yuan, H. Wang, L. Jiang, Z. Wu, H. Wang and X. Cao, Integrating the (311) facet of MnO2 and the fuctional groups of poly(m-phenylenediamine) in core-shell MnO2@poly(m-phenylenediamine) adsorbent to remove Pb ions from water, J. Hazard. Mater., 2020, 389, 122154 CrossRef CAS PubMed.
- J. Wang, Z. Deng, T. Feng, J. Fan and W.-X. Zhang, Nanoscale zero-valent iron (nZVI) encapsulated within tubular nitride carbon for highly selective and stable electrocatalytic denitrification, Chem. Eng. J., 2021, 417, 129160 CrossRef CAS.
- W. Mao, T. Wang, H. Wang, S. Zou and S. Liu, Novel Bi2WO6 loaded g-C3N4 composites with enhanced photocatalytic degradation of dye and pharmaceutical wastewater under visible light irradiation, J. Mater. Sci.: Mater. Electron., 2018, 29, 15174–15182 CrossRef CAS.
- K. Liu, F. Li, J. Cui, S. Yang and L. Fang, Simultaneous removal of Cd(II) and As(III) by graphene-like biochar-supported zero-valent iron from irrigation waters under aerobic conditions: Synergistic effects and mechanisms, J. Hazard. Mater., 2020, 395, 122623 CrossRef CAS PubMed.
- B. Leclercq, H. Kabbour, A. M. Arevalo-Lopez, S. Daviero-Minaud, C. Minaud, R. David and O. Mentré, Synthesis, structure and magnetic behavior of iron arsenites with hierarchical magnetic units, Inorg. Chem. Front., 2020, 7, 3987–3999 RSC.
- W. Jiang, J. Lv, L. Luo, K. Yang, Y. Lin, F. Hu, J. Zhang and S. Zhang, Arsenate and cadmium co-adsorption and co-precipitation on goethite, J. Hazard. Mater., 2013, 262, 55–63 CrossRef CAS PubMed.
- D. Huang, J. Wu, L. Wang, X. Liu, J. Meng, X. Tang, C. Tang and J. Xu, Novel insight into adsorption and co-adsorption of heavy metal ions and an organic pollutant by magnetic graphene nanomaterials in water, Chem. Eng. J., 2019, 358, 1399–1409 CrossRef CAS.
- X. Ren, S. Yang, X. Tan, C. Chen, G. Sheng and X. Wang, Mutual effects of copper and phosphate on their interaction with gamma-Al2O3: combined batch macroscopic experiments with DFT calculations, J. Hazard. Mater., 2012, 237–238, 199–208 CrossRef CAS PubMed.
- X. Ren, Q. Wu, H. Xu, D. Shao, X. Tan, W. Shi, C. Chen, J. Li, Z. Chai, T. Hayat and X. Wang, New Insight into GO, Cadmium(II), Phosphate Interaction and Its Role in GO Colloidal Behavior, Environ. Sci. Technol., 2016, 50, 9361–9369 CrossRef CAS PubMed.
- Y.-S. Han, J.-H. Park, Y. Min and D.-H. Lim, Competitive adsorption between phosphate and arsenic in soil containing iron sulfide: XAS experiment and DFT calculation approaches, Chem. Eng. J., 2020, 397, 125426 CrossRef CAS.
- Y. Wei, S. Wei, C. Liu, T. Chen, Y. Tang, J. Ma, K. Yin and S. Luo, Efficient removal of arsenic from groundwater using iron oxide nanoneedle array-decorated biochar fibers with high Fe utilization and fast adsorption kinetics, Water Res., 2019, 167, 115107 CrossRef CAS PubMed.
- Y. Georgiou, I. T. Papadas, E. Mouzourakis, E. Skliri, G. S. Armatas and Y. Deligiannakis, Mesoporous spinel CoFe2O4 as an efficient adsorbent for arsenite removal from water: high efficiency via control of the particle assemblage configuration, Environ. Sci.: Nano, 2019, 6, 1156–1167 RSC.
- Y. Li, X. Qi, G. Li and H. Wang, Double-pathway arsenic removal and immobilization from high arsenic-bearing wastewater by using nature pyrite as in situ Fe and S donator, Chem. Eng. J., 2021, 410, 128303 CrossRef CAS.
- Z. Fan, Q. Zhang, M. Li, W. Sang, Y. Qiu, X. Wei and H. Hao, Removal behavior and mechanisms of Cd(II) by a novel MnS loaded functional biochar: Influence of oxygenation, J. Cleaner Prod., 2020, 256, 120672 CrossRef CAS.
- J. Jiang, R. K. Xu, T. Y. Jiang and Z. Li, Immobilization of Cu(II), Pb(II) and Cd(II) by the addition of rice straw derived biochar to a simulated polluted Ultisol, J. Hazard. Mater., 2012, 229–230, 145–150 CrossRef CAS PubMed.
- Y. Jia, L. Xu, X. Wang and G. P. Demopoulos, Infrared spectroscopic and X-ray diffraction characterization of the nature of adsorbed arsenate on ferrihydrite, Geochim. Cosmochim. Acta, 2007, 71, 1643–1654 CrossRef CAS.
- C. Wang, T. Hu, Z. Wen, J. Zhou, X. Wang, Q. Wu and C. Wang, Concentration-dependent color tunability of nitrogen-doped carbon dots and their application for iron(III) detection and multicolor bioimaging, J. Colloid Interface Sci., 2018, 521, 33–41 CrossRef CAS PubMed.
- W. Zhang, G. Zhang, C. Liu, J. Li, T. Zheng, J. Ma, L. Wang, J. Jiang and X. Zhai, Enhanced removal of arsenite and arsenate by a multifunctional Fe-Ti-Mn composite oxide: Photooxidation, oxidation and adsorption, Water Res., 2018, 147, 264–275 CrossRef CAS PubMed.
- Q. Zhou, B. Liao, L. Lin, W. Qiu and Z. Song, Adsorption of Cu(II) and Cd(II) from aqueous solutions by ferromanganese binary oxide-biochar composites, Sci. Total Environ., 2018, 615, 115–122 CrossRef CAS PubMed.
- S. Li, J. Tang, Q. Liu, X. Liu and B. Gao, A novel stabilized carbon-coated nZVI as heterogeneous persulfate catalyst for enhanced degradation of 4-chlorophenol, Environ. Int., 2020, 138, 105639 CrossRef CAS PubMed.
- S. Mortazavian, E. R. Bandala, J.-H. Bae, D. Chun and J. Moon, Assessment of p-nitroso dimethylaniline (pNDA) suitability as a hydroxyl radical probe: Investigating bleaching mechanism using immobilized zero-valent iron nanoparticles, Chem. Eng. J., 2020, 385, 123748 CrossRef CAS.
- T. Harada, T. Yatagai and Y. Kawase, Hydroxyl radical generation linked with iron dissolution and dissolved oxygen consumption in zero-valent iron wastewater treatment process, Chem. Eng. J., 2016, 303, 611–620 CrossRef CAS.
- X. Zhang, M. Wu, H. Dong, H. Li and B. Pan, Simultaneous Oxidation and Sequestration of As(III) from Water by Using Redox Polymer-Based Fe(III) Oxide Nanocomposite, Environ. Sci. Technol., 2017, 51, 6326–6334 CrossRef CAS PubMed.
- Q. Liu, L. B. Zhong, Q. B. Zhao, C. Frear and Y. M. Zheng, Synthesis of Fe3O4/Polyacrylonitrile Composite Electrospun Nanofiber Mat for Effective Adsorption of Tetracycline, J. Colloid Interface Sci., 2015, 7, 14573–14583 CAS.
- M. Zhu, K. W. Paul, J. D. Kubicki and D. L. Sparks, Quantum Chemical Study of Arsenic (III, V) Adsorption on Mn-Oxides: Implications for Arsenic(III) Oxidation, Environ. Sci. Technol., 2009, 43, 6655–6661 CrossRef CAS PubMed.
- O. R. Harvey, B. E. Herbert, R. D. Rhue and L. J. Kuo, Metal interactions at the biochar-water interface: energetics and structure-sorption relationships elucidated by flow adsorption microcalorimetry, Environ. Sci. Technol., 2011, 45, 5550–5556 CrossRef CAS PubMed.
- H. Peng, P. Gao, G. Chu, B. Pan, J. Peng and B. Xing, Enhanced adsorption of Cu(II) and Cd(II) by phosphoric acid-modified biochars, Environ. Pollut., 2017, 229, 846–853 CrossRef CAS PubMed.
- M. Stachowicz, T. Hiemstra and W. H. van Riemsdijk, Multi-competitive interaction of As(III) and As(V) oxyanions with Ca(2+), Mg(2+), PO(3-)(4), and CO(2-)(3) ions on goethite, J. Colloid Interface Sci., 2008, 320, 400–414 CrossRef CAS.
- B. Li, D. Wei, Y. Zhou, Y. Huang, B. Tie and M. Lei, Mechanisms of arsenate and cadmium co-immobilized on ferrihydrite inferred from ternary surface configuration, Chem. Eng. J., 2021, 424, 130410 CrossRef CAS.
Footnote |
† Electronic supplementary information (ESI) available. See DOI: 10.1039/d1en00722j |
|
This journal is © The Royal Society of Chemistry 2022 |