Toxic effects of carbon quantum dots on the gut–liver axis and gut microbiota in the common carp Cyprinus carpio†
Received
19th July 2021
, Accepted 16th November 2021
First published on 17th November 2021
Abstract
The potential toxicity of carbon quantum dots (CQDs) has received much attention because of their increasing biomedical applications. However, the impacts of CQDs on the gut–liver axis and gut microbiota of the host remain unclear. In this study, we investigated the effects of CQD chronic exposure (5 weeks) on the biodistribution, histopathology, antioxidant capacity, immune response, lipid metabolism, and gut microbiota composition in the common carp Cyprinus carpio at different concentrations (2 and 20 mg kg−1 body weight). The results demonstrated that CQDs heavily accumulated in the intestine and liver, severely damaging these organs. Superoxide dismutase and glutathione were reduced and malondialdehyde was increased in intestinal and liver tissues, and the nrf2/ho-1 signaling pathway was downregulated. In addition, CQDs activated toll-like receptor pathways to mediate inflammatory responses. Serum lipid levels were altered after CQD exposure, and srebp-1c, fas, hmgcr, and fxr expression was upregulated and cyp7a1 and cyp27a1 expression was downregulated in the intestine and liver. Interestingly, mttp and cpt-1α expression was upregulated and abca1, abcg8, and fgf19 expression was downregulated in the intestine, whereas their expression was opposite in the liver. Finally, 16S rRNA sequence analysis showed that gut microbiota diversity and richness were reduced, and the relative abundance of harmful bacteria increased and that of beneficial bacteria decreased. Bioinformatic analysis showed that the antioxidant capacity, immune response, and lipid metabolism also correlated with the relative abundance of gut microbial genera. In summary, this study provides reliable data for toxicological risk assessment of CQDs in aquatic ecosystems.
Environmental significance
As CQDs have several applications, they are inevitably released into the environment, increasing ecological risks to aquatic ecosystems and causing human health problems. Therefore, to better utilize CQDs, it is essential to focus on their toxic effects. The potential toxicological mechanisms of CQDs on common carp were investigated in this study. Five week CQD exposure resulted in oxidative stress accompanied by inflammatory responses, intestinal and liver damage, gut microbiota dysbiosis, and lipid metabolism disturbances in carp. Of these, the gut microbiota was the key factor associated with various toxicological indicators. In short, these findings provide more insights into the aquatic ecotoxicity of carbon nanomaterials.
|
1. Introduction
Carbon quantum dots (CQDs) are fluorescent carbon nanoparticles consisting of discrete quasi-spherical carbon nanoparticles smaller than 10 nm.1 Compared with other nanomaterials, CQDs have unique properties, such as small particle size, high water solubility, good fluorescence, low biotoxicity, and good biocompatibility.2–4 They are widely used in photocatalysis, biosensing, drug delivery, biodetection, and bioimaging.5 Therefore, thoroughly investigating the toxicity and environmental impact of CQDs is necessary to ensure biosafety.6
Studies on CQD toxicity in aquatic ecosystems are few and toxicological data are inconsistent. CQDs at low concentrations are biocompatible and environmentally safe; for example, in zebrafish (Danio rerio), embryos grown in CQD concentrations up to 5.0 mg L−1 did not affect their development.7 However, high CQD concentrations caused damage to the brain, gills, heart, liver, and intestinal tissues of adult zebrafish. When the CQD biodistribution in the embryos and larvae of zebrafish was analyzed, a strong fluorescence signal was observed in the embryos at 6 hpf, and CQD accumulation in the digestive system of larvae was most pronounced at 5 dpf.8 In addition, high CQD concentrations exhibited marked developmental toxicity via oxidative stress and DNA damage in the embryos and larvae of rare minnow (Gobiocypris rarus).9 CQDs at 1 mg mL−1 decreased the survival of bladder snail (Physa acuta), and different CQD concentrations were chronically toxic and not acutely toxic to fish.10 CQDs were also detrimental to microalgal growth; they caused reactive oxygen species production and inhibited photosynthesis-related gene transcription in Chlorella vulgaris.11 These studies suggest that CQD accumulation and toxic effects on organisms are dependent on species and their growth stage, and related to factors such as CQD particle size and exposure duration. However, further studies, particularly in vivo investigations, are required for other species. The common carp Cyprinus carpio is an abundant freshwater fish in China, and it can be used as a bioindicator to evaluate environmental pollution.12,13 Moreover, the effects of CQD toxicity on carp are unknown and can have important ecological implications in toxicological assessment.
The intestine and liver communicate through a bidirectional connection between the portal vein and bile duct known as the gut–liver axis.14 The gut–liver axis plays a vital role in both health and pathogenesis of the gut and liver, with the gut microbiota being a major determinant of this relationship.15 Intestinal bacteria are involved in the regulation of several physiological functions, and many diseases are associated with changes in the gut microbiota composition.16 Therefore, intestinal bacteria can serve as important toxicological indicators of environmental pollution.
CQDs are toxic to viruses, bacteria, and fungi.17–19 Thus, ingested CQDs may interact with the gut microbiota, affect the host metabolism, and indirectly cause a series of biological effects in the host organism. The liver, which plays a pivotal role in various metabolic processes, is a target organ of exogenous toxicants.20 Generally, exogenous chemicals can cause lipid peroxidation-derived free radical production, whereas overproduced oxidative products result in hepatic damage.21 Furthermore, lipid metabolism, which occurs in the liver, and its homeostasis is essential for maintaining the basic physiological functions of organisms.22 However, the potential CQD toxicity in terms of impacts on the gut microbiota, tissue injury, and lipid metabolism in carp remains ambiguous and needs further exploration.
In this study, the biodistribution and histopathology of CQDs in common carp were investigated; changes in antioxidant capacity, immune response, lipid metabolism, and gut microbiota in response to CQD exposure were assessed; the association of gut microbiota changes with toxicological responses was explored; and the possible mechanisms of CQD-induced toxicity from the viewpoint of the gut–liver axis were elucidated. These findings will not only enhance the knowledge of the biocompatibility and risk assessment of CQDs but also expand the understanding of the role of the gut flora in ecotoxicology.
2. Materials and methods
2.1 Chemicals
CQD aqueous solutions were purchased from Xingzi New Material Technology Development Co., Ltd. (Shanghai, China). CQD morphology was examined by transmission electron microscopy (TEM; JEOL, Japan), and the size of CQD nanoparticles was determined using dynamic light scattering (ELS-Z2; Otsuka Electronics Co., Ltd., Japan). The photoluminescence spectra were recorded on an RF-5301PC fluorophotometer (Shimadzu, Japan). The CQD solution was stored in an opaque glass bottle before the experiment.
2.2 Animal and experimental design
Young common carp (28.80 ± 0.59 g, 12.90 ± 0.64 cm) were obtained from a commercial farm in Xinxiang City, Henan Province, China. A total of 300 fish were kept in three 350 L tanks for 2 weeks to acclimatize. During acclimatization and exposure, carp were fed twice a day (8 a.m. and 6 p.m.) on a commercial basal diet (Tongwei, Henan Province, China) at 2% of body weight (BW), with daily aspiration to remove uneaten food, and one-third of the water was replaced. Water temperature was maintained at 23 ± 2 °C, pH was 7.2 ± 0.3, dissolved oxygen was 6.0 ± 0.3 mg L−1, ammonia nitrogen was <0.01 mg L−1, water hardness (230 mg CaCO3 per L) was 42.5 ± 1.2 calcium mg L−1, and photoperiod of light
:
dark was 12
:
12 h.
After acclimatization, the carp were randomly divided into three groups: control group CK [0.01 M sterile phosphate-buffered saline (PBS; Sigma-Aldrich)] and treatment groups C1 and C2 with CQD at concentrations of 2 and 20 mg kg−1 BW, respectively. Tanks (74 × 50 × 54 cm) with 100 L of water were used to keep 30 fish. Three replicates were performed for each group. Before dosing, CQD suspensions (i.e., 2 and 20 mg kg−1 BW concentrations) were prepared in 0.01 M PBS solution and sonicated for 15 min to avoid aggregation. An oral dose of 10 mL kg−1 BW was administered to each fish weekly, and samples were taken after 5 weeks.
In each tank, 25 fish were randomly collected and anesthetized with 100 mg L−1 ethyl 3-aminobenzoate methanesulfonate (MS-222). Six fish were randomly selected, and blood was collected from the caudal vein to test serum biochemical indicators; 5 fish midguts and livers were immersed in 10% buffered formalin (v/v) for histological analysis; 6 fish midguts and livers were frozen in liquid nitrogen and stored at −80 °C for evaluation of biochemical analysis and total RNA extraction; 8 fish were selected and scraped with a sterile slide and stored in liquid nitrogen for gut microbiota analysis.
All animal procedures were performed in accordance with the Guidelines for Care and Use of Laboratory Animals of Henan Normal University and approved by the Animal Ethics Committee of Henan Normal University (Approval ID: HNSD-SMKX-2120BS0716).
2.3
In vivo and ex vivo imaging of CQD biodistribution
Five fish of uniform size and shape were selected to evaluate CQD biodistribution. These fish were fasted for 24 h before the experiment. They were administered a single oral dose of CQDs at 20 mg kg−1 BW and were anesthetized using an overdose of MS-222. The time-dependent biodistribution in the fish was visualized by an in vivo fluorescence imaging system (IVIS Spectrum; Perkin Elmer, USA) with a light excitation source of 430 nm. In vivo spectral imaging was performed at 520 nm with a 150 ms exposure time for each image frame. Scans were performed on day 1, 4, 7, 12, and 14 post-treatment. The liver, intestine, brain, kidney, spleen, and gills were excised after day 14, and ex vivo fluorescence imaging was performed using the IVIS system.
2.4 Histological analysis
In the 5th week, liver and midgut tissues were collected and placed in 10% neutral formalin buffer for 12 h. The tissue samples were sectioned at a 3 μm thickness in a rotary microtome (Leica PM2245; Germany), stained with hematoxylin and eosin, and observed under a light microscope (Nikon Eclipse E400; Japan). Subsequently, the presence of CQDs (fluorescent blue) in fish tissues was determined using a fluorescence microscope (Zeiss Axio Vert.A1; Germany), and the fluorescence intensity was quantified using ImageJ software (version 1.51; NIH, Bethesda, MD, USA).
2.5 Biochemical analysis
Superoxide dismutase (SOD) activity, glutathione (GSH) content, and malondialdehyde (MDA) content in the intestine and liver were measured using the SOD Activity Assay Kit (BC0175; Solarbio), GSH Assay Kit (BC1175; Solarbio), and MDA Assay Kit (BC0025; Solarbio), respectively, following the manufacturer's instructions. Total triglyceride (TG), total cholesterol (CHO), low-density lipoprotein cholesterol (LDL-C), and high-density lipoprotein cholesterol (HDL-C) levels in serum were measured using matched reagents and an automatic biochemistry analyzer (BS-200; Shenzhen Mindray Bio-Medical Electronics Co., Ltd., Shenzhen, China) according to the manufacturer's protocol.
2.6 Quantitative RT-PCR analysis
Total RNA was isolated using TRIzol reagent (Invitrogen, USA), and cDNA was synthesized from 1 μg RNA using the PrimeScript Reverse Transcriptase Kit (Takara, Japan). Quantitative real-time PCR (qRT-PCR) was performed with 5 μL of SYBR Green Supermix, 0.2 μL of each primer, 0.5 μL of cDNA, and 4.1 μL of DNase/RNase-free water. The primer sequences are listed in Table S1.† All primers were designed using Primer Premier 5.0 software and synthesized by Sangon Biotech (Shanghai, China). Relative expression levels of target genes were determined by the 2−ΔΔCT method, and the 40S ribosomal subunit was used as a housekeeping gene.
2.7 Gut microbiota analysis
Intestinal microbial DNA was isolated using the QIAamp DNA Stool Mini Kit (Qiagen Inc., Hilden, Germany) according to the manufacturer's instructions. Bacterial 16S rRNA (V3–V4 region) was amplified by PCR using the universal primer 338F/806R (338F 5′-ACTCCTACGGGAGGCAGCAG-3′, 806R 5′-GGACTACGVGGGTWTCTAAT-3′), and the products were purified using the TIANgel Mini Purification Kit (Tiangen, Beijing, China). MiSeq library construction and sequencing were performed using the Illumina MiSeq PE300 platform (Illumina, San Diego, CA, USA). The reads were filtered by Quantitative Insights Into Microbial Ecology (QIIME, http://qiime.org/tutorials/processing_illumina_data.html) quality filters. All bioinformatics data were analyzed on the Majorbio cloud platform (www.majorbio.com).
2.8 Statistical analysis
One-way analysis of variance (ANOVA) in IBM SPSS Statistics 25.0 (Chicago, IL, USA) followed by Tukey's test was performed to determine the significant differences among the three groups. Differences were considered significant at P < 0.05. Graphs were created using GraphPad Prism (version 5; GraphPad Software Inc., USA). All data of the gut microbiota abundance were analyzed using the pheatmap package in R (version 3.1.1). Correlations between bacterial genera abundance and antioxidant, immune–inflammatory, and lipid metabolism parameters were analyzed using Spearman's correlation coefficients based on the heatmap analysis; r > 0.5 or r < −0.5 and P < 0.05 were considered to be significantly correlated.
3. Results
3.1 Characterization of CQDs
A uniform CQD morphology was observed by TEM (Fig. 1A), and CQDs had a diameter of approximately 3.1 nm (Fig. 1B). The emission spectrum was narrow and symmetric, with a maximum emission wavelength of CQDs at approximately 460 nm (Fig. 1C).
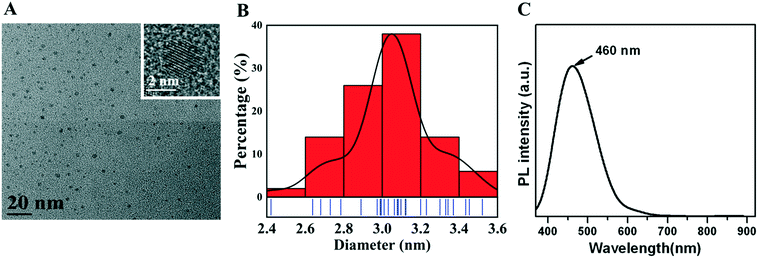 |
| Fig. 1 Characterization of CQDs. (A) CQDs under the transmission electron microscope (scale bar = 20 nm). (B) CQD diameter of 3.0–3.2 nm. (C) Maximum emission wavelength of CQDs is approximately 460 nm in the photoluminescence spectrum. | |
3.2
In vivo and ex vivo imaging of CQD biodistribution
Biodistribution was evaluated in carp after CQD oral administration (Fig. 2). On days 1, 4, 7, 12, and 14, fluorescent signals were detected in the carp digestive system, particularly near the anus. On day 14, the fluorescent signal decreased, and ex vivo fluorescence imaging showed that CQDs were distributed in intestinal and liver tissues, whereas no fluorescent signal was observed in other tissues.
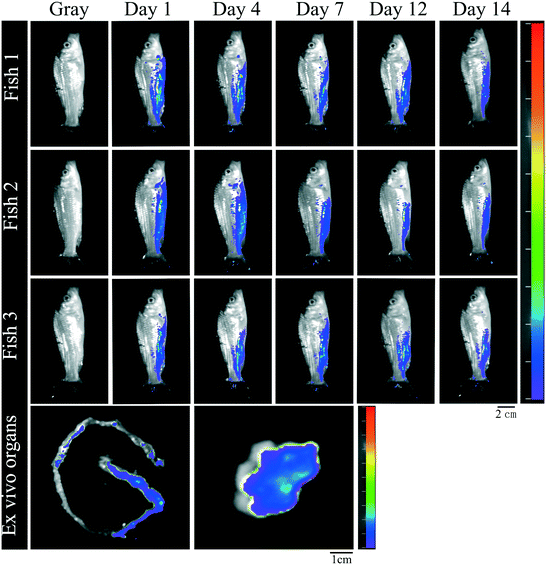 |
| Fig. 2
In vivo fluorescence in carp at different time points after CQD oral administration, and ex vivo fluorescence in the intestine and liver sacrificed on day 14 (λex = 460 nm, λem = 520 nm). | |
3.3 Toxic effects on the carp intestine after CQD exposure
3.3.1 CQD fluorescence distribution and morphology in intestinal tissue.
At the histological level, a visible blue fluorescence was clearly observed in the treatment groups (i.e., C1 and C2) compared with the control group (i.e., CK), and the fluorescence intensity in intestinal tissue was significantly higher in the C2 group than in the C1 group (Fig. 3A–C and S1†). In addition, multiple intestinal abnormalities were observed in the CQD treatment groups (Fig. 3D–F). The intestinal folds were significantly shortened and had infiltrating cells (Fig. 3E). Localized shedding of epithelial cells was also observed in the intestinal epithelial layer (Fig. 3F). Compared with the control group, the most significant histomorphological change in the CQD treatment groups was a reduction in fold height and muscle thickness (P < 0.01, P < 0.01; Fig. 3G and H); however, a significant increase was observed in villi width (P < 0.05, P < 0.01; Fig. 3I).
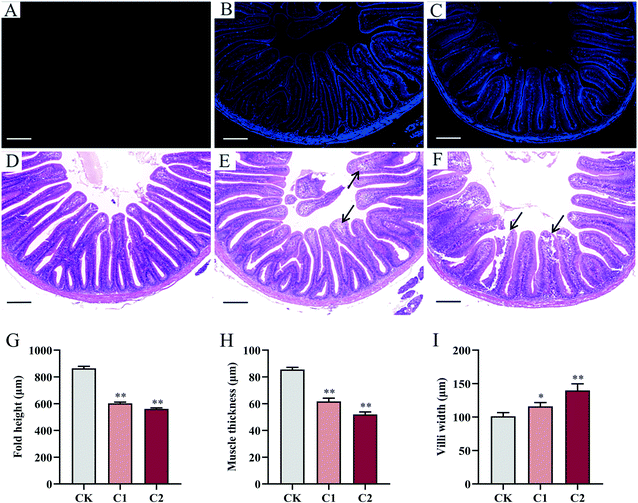 |
| Fig. 3 Fluorescence distribution and morphology of carp intestinal tissue after 5 week CQD exposure. (A–C) Fluorescent CQDs in the carp intestine. (D) Structure of normal carp intestine in the control group. (E) CQD exposure caused histopathological changes [e.g., inflammatory cell infiltration (arrows)] in intestinal tissue in group C1. (F) CQD exposure caused severe histopathological changes [e.g., necrosis of epithelial cells (left arrow) and inflammatory cell infiltration (right arrow)] in intestinal tissue in group C2 (scale bar = 200 μm). (G–I) Effects of CQD exposure on fold height, muscle thickness, and villi width in carp. Values are means ± SEM (n = 6). Statistical significance was analyzed by factorial ANOVA (*P < 0.05, **P < 0.01). | |
3.3.2 Changes in intestinal tight junctions, oxidative stress, and immune–inflammatory responses.
To investigate the effect of CQD exposure on intestinal integrity, the mRNA levels of intestinal mucosa tight junction genes were examined (Fig. 4A–C). Occludin, claudin-2, and zonula occludens-1 mRNA levels were significantly lower in the CQD treatment groups than in the control group (P < 0.05, P < 0.01). However, low CQD doses had no significant effect on the zonula occludens-1 mRNA level (P = 0.067).
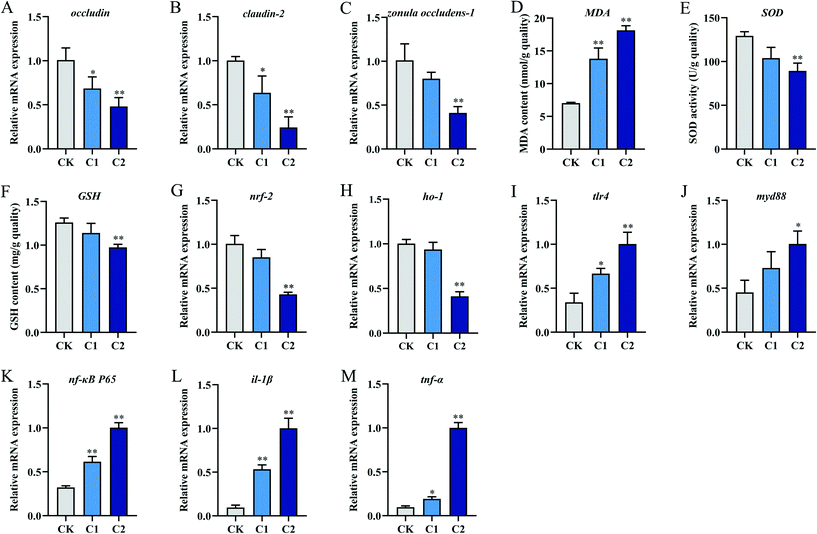 |
| Fig. 4 Changes in intestinal tight junctions, oxidative stress, and immune–inflammatory responses in carp after 5 week CQD exposure. Intestinal tight junctions [mRNA levels of occludin (A), claudin-2 (B), and zonula occludens-1 (C)], intestinal oxidative stress [MDA (D), SOD (E), GSH (F), nrf2 (G), and ho-1 (H)], and intestinal inflammation [mRNA levels of tlr4 (I), myd88 (J), nf-κB/p65 (K), il-1β (L), and tnf-α (M) in intestinal tissue]. Values are means ± SEM (n = 6). Values of the mRNA expression levels were normalized to 40S expressed as a ratio to the control. Statistical significance was analyzed by factorial ANOVA (*P < 0.05, **P < 0.01). | |
The intestinal antioxidant levels in the CQD treatment groups were different from those in the control group (Fig. 4D–F). With increasing CQD doses, MDA content significantly increased (P < 0.01, P < 0.01) and SOD activity significantly decreased (P < 0.05, P < 0.01). In addition, GSH levels were significantly inhibited in group C2 (P < 0.01) but not in group C1 (P = 0.124). Furthermore, the expression levels of the oxidative stress-related genes nuclear factor erythroid 2-related factor 2 (nrf2) and heme oxygenase-1 (ho-1) decreased in group C2 (Fig. 4G and H).
After CQD exposure, the mRNA levels of toll-like receptor 4 (tlr4), myeloid differentiation protein-88 (myd88), nuclear factor-κB/p65 (nf-κB/p65), interleukin-1β (il-1β), and tumor necrosis factor-α (tnf-α) were highly elevated compared with the control group (Fig. 4I–M).
3.4 Toxic effects on the carp liver after CQD exposure
3.4.1 Fluorescence distribution and morphology of CQDs in liver tissue.
CQD accumulation in the liver was confirmed by blue fluorescence in the CQD treatment groups (Fig. 5A–C), and the fluorescence intensity was the highest in group C2 (Fig. S2†). This result coincided well with the ex vivo fluorescence imaging results. CQDs caused histopathological changes such as hepatocyte swelling, cytoplasmic vacuolization, ballooning degeneration, and increased fatty changes (Fig. 5E and F).
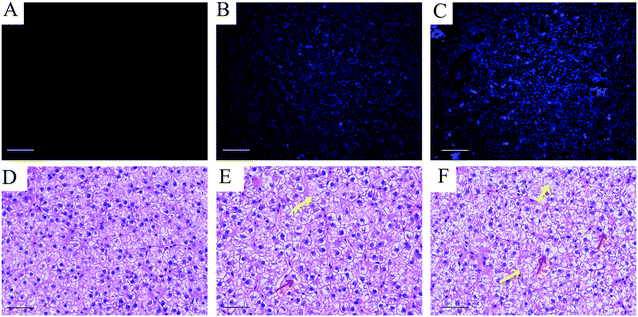 |
| Fig. 5 Fluorescence distribution and morphology of carp liver tissue after 5 week CQD exposure. (A–C) Fluorescent CQDs in the carp liver. (D–F) Normal hepatocyte morphology in group CK. (E) Liver morphology in group C1. (F) Liver morphology in group C2. Red and yellow arrow heads indicate hepatocyte swelling or cytoplasmic vacuolization and increased fatty changes, respectively (scale bar = 200 μm). | |
3.4.2 Changes in oxidative stress, detoxification metabolism, and immune–inflammatory responses.
The changes in MDA, SOD, and GSH levels in liver tissue are summarized in Fig. 6(A–C). MDA content was significantly higher in group C2 than in the control group (P < 0.01), but no statistical significance was reached for group C1 (P = 0.094). In contrast, SOD activity and GSH content in the CQD treatment groups were remarkably reduced, and both declined in a dose-dependent manner. This was consistent with the trend in antioxidant levels in intestinal tissue. Furthermore, after 5 weeks of CQD exposure, the expression levels of the oxidative stress-related genes nrf2 and ho-1 decreased (Fig. 6D and E).
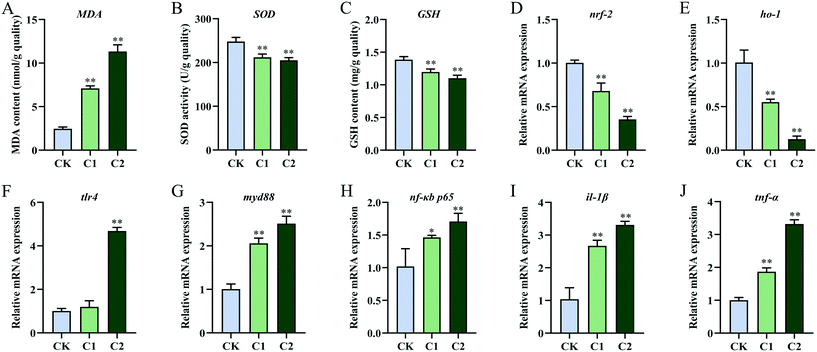 |
| Fig. 6 Changes in oxidative stress, detoxification metabolism, and immune–inflammatory responses in carp liver after 5 week CQD exposure. Liver oxidative stress and detoxification metabolism [MDA (A), SOD (B), GSH (C), nrf2 (D), and ho-1 (E)] and liver inflammation [mRNA levels of tlr4 (F), myd88 (G), nf-κB/p65 (H), il-1β (I), and tnf-α (J) in liver tissue]. Values are means ± SEM (n = 6). Values of the mRNA expression levels were normalized to 40S expressed as a ratio to the control. Statistical significance was analyzed by factorial ANOVA (*P < 0.05, **P < 0.01). | |
The expression levels of the immune–inflammation-related genes tlr4, myd88, nf-κB/p65, il-1β, and tnf-α were significantly higher in the CQD treatment groups than in the control group (Fig. 6F–J). However, no significant difference in gene expression was observed for tlr4 in group C1 (P = 0.11, P = 0.18).
3.5 Effect of CQDs on the gut microbiota of carp
3.5.1 Gut microbiota diversity.
The effects of different CQD concentrations on the gut microbiota of carp were analyzed using bacterial 16S rRNA high-throughput sequencing. The total bases, sequence number, and Good's coverage are listed in Table S2.† Good's coverage estimates showed that more than 99% of species were identified in the nine samples, indicating that sufficient sampling depth was achieved in all the samples. A Venn diagram indicated that 209 operational taxonomic units (OTUs) were present in the three groups, and the number of unique OTUs was 60, 30, and 19 in the CK, C1, and C2 groups, respectively (Fig. 7A). CQD exposure reduced gut microbiota OTUs—the higher the concentration, the lower the OTU. Rank-abundance curves indicated that the abundance and evenness of species in all the samples were reasonable (Fig. S1†).
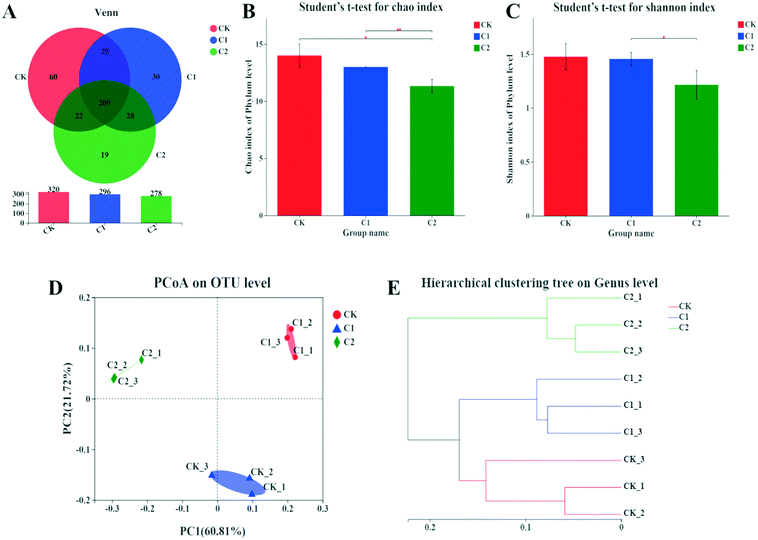 |
| Fig. 7 Comparisons of α-diversity and β-diversity of the gut microbiota between the control and CQD treatment groups. (A) Venn diagram of bacterial OTUs shared and unique to the intestinal microbiota in different groups. (B) Microbiota richness (Chao1 index). (C) Microbiota diversity (Shannon index). (D) Unweighted UniFrac PCoA of the gut microbiota in carp. (E) Hierarchical cluster analysis was performed using the community distance matrix and shows the OTU similarity of the gut microbiota between the different groups. Values are means ± SEM (n = 3). Asterisks indicate significant differences (*P < 0.05). | |
The α-diversity of the gut microbiota in the three groups was evaluated by the Chao1 richness index and the Shannon diversity index. The Chao1 richness index was significantly lower in group C2 than in groups CK and C1 (P < 0.05, P < 0.01; Fig. 7B). The Shannon diversity index was significantly lower in group C2 than in group C1 (P < 0.05; Fig. 7C). In addition, the β-diversity of the gut microbiota in the three groups was assessed by principal coordinates analysis (PCoA) and hierarchical cluster analysis. Based on the PCoA of unweighted UniFrac distances, the gut microbiota samples in the three groups were categorized into three distinct groups (Fig. 7D). The hierarchical cluster tree shows that the gut microbiota in the three groups was divided into two clusters, with groups CK and C1 in one cluster and group C2 in a separate cluster (Fig. 7E). This suggested a concentration-dependent effect of CQDs on the gut microbes of carp.
3.5.2 Gut microbiota composition.
Gut microbes were categorized at the phylum and genus levels (i.e., 19 phyla and 221 genera) according to the default settings in Mothur. The phyla with more than 1% abundance in all the samples were Proteobacteria, Actinobacteriota, Fusobacteriota, Bacteroidota, Firmicutes, Chloroflexi, Verrucomicrobiota, and Planctomycetota. The remaining phyla (Others) were present in low abundance (<1%; Fig. 8A). A Kruskal–Wallis H test showed significant differences in seven of the 15 phyla (false discovery rate-corrected P < 0.05; Fig. 8B). On exposure to high CQD doses, the relative abundance of Actinobacteriota and Chloroflexi increased and that of Proteobacteria decreased in the carp intestine. In addition, the relative abundance of Verrucomicrobiota and Bacteroidota in the C2 group decreased compared with the control group, but neither of them was significant.
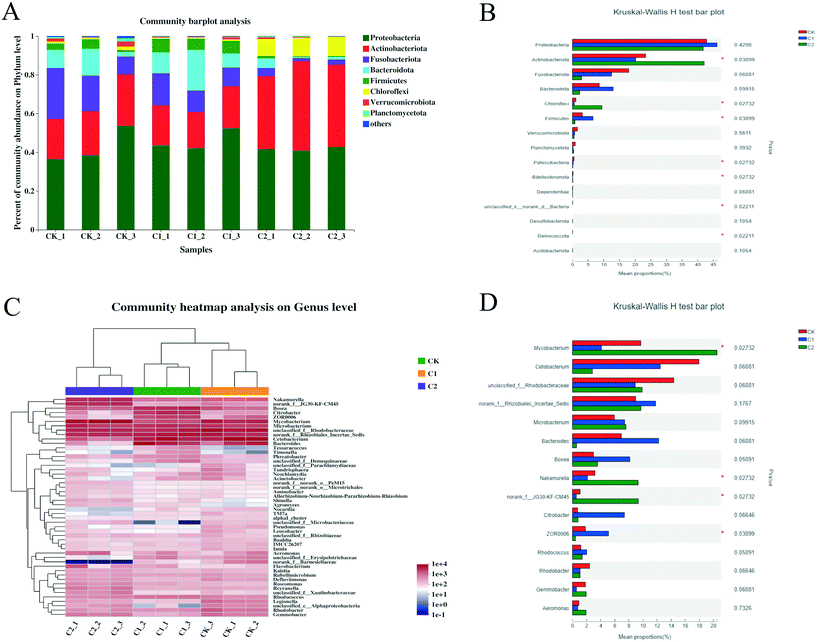 |
| Fig. 8 Effect of CQDs on the gut microbiota composition at the phylum and genus levels. Community abundance (A) and differential analysis (B) at the phylum level. Community abundance (C) and differential analysis (D) at the genus level. Values are means ± SEM (n = 3). Asterisks indicate significant differences (*P < 0.05). | |
A hierarchical cluster analysis of the top 50 most abundant bacteria was also performed to evaluate changes in the gut microbiota at the genus level (Fig. 8C). The heatmap showed the different microbial communities, as well as their abundant and non-abundant genera, among the three groups. The Kruskal–Wallis H test indicated four differentially distributed genera among the three groups (Fig. 8D). The percentage of Mycobacterium, Nakamurella, and norank_f_JG30-KF-CM45 was remarkably higher in group C2 than in groups CK and C1 (P < 0.05). In addition, the proportion of ZOR0006 was significantly lower in groups CK and C2 than in group C1 (P < 0.05).
3.6 Changes in lipid metabolism after CQD exposure
Plasma lipid levels were measured to investigate whether CQD exposure affected lipid metabolism in carp. The changes in serum TG, CHO, HDL-C, and LDL-C levels after 5 week CQD exposure are listed in Fig. 9A. TG, CHO, and LDL-C levels were significantly higher in group C2 than in the control group (P < 0.05, P < 0.01, P < 0.01). However, the HDL-C level was remarkably decreased (P < 0.01).
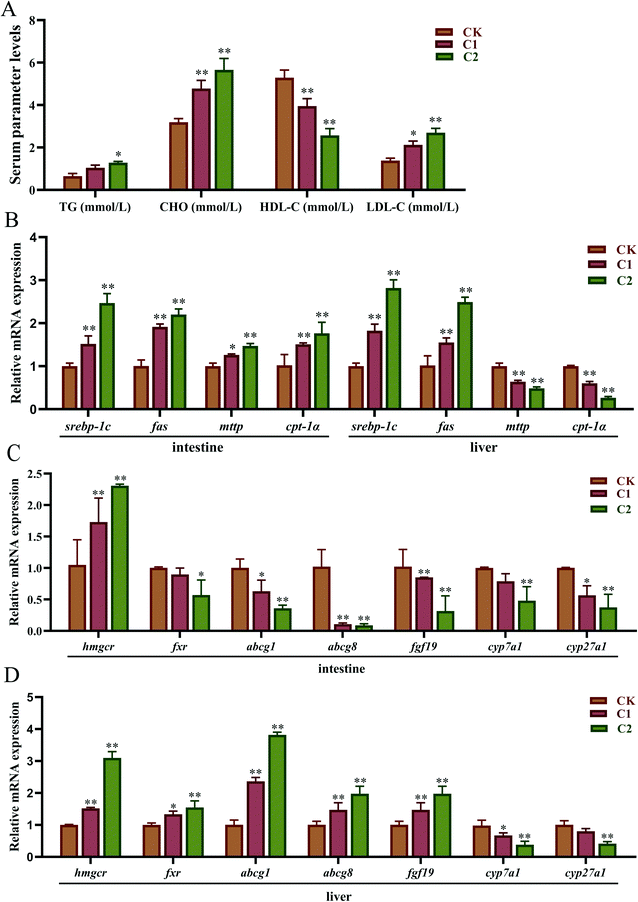 |
| Fig. 9 Disruption of lipid metabolism in carp caused by CQD exposure. (A) Serum lipid levels (TG, CHO, HDL-C, and LDL-C). (B) Triglyceride metabolism in the intestine and liver (mRNA levels of srebp-1c, fas, mttp, and cpt-1α). (C) Cholesterol metabolism in the intestine (mRNA levels of hmgcr, fxr, abcg1, abcg8, fgf19, cyp7a1, and cyp27a1). (D) Cholesterol metabolism in the liver (mRNA levels of hmgcr, fxr, abcg1, abcg8, fgf19, cyp7a1, and cyp27a1). Values are means ± SEM (n = 6). Values of the mRNA expression levels were normalized to 40S expressed as a ratio to the control. Statistical significance was analyzed by factorial ANOVA (*P < 0.05, **P < 0.01). | |
Lipid metabolism-related genes were measured to further assess the effect of CQDs on lipid metabolism. First, the expression of TG metabolism-related genes was investigated (Fig. 9B). In the intestine, CQD exposure increased the transcriptional levels of sterol regulatory element-binding protein-1 c (srebp-1c), fatty acid synthase (fas), microsomal TG transfer protein (mttp), and carnitine palmitoyltransferase-1α (cpt-1α). In the liver, srebp-1c and fas expression was significantly upregulated in a concentration-dependent manner (P < 0.01). In contrast, mttp and cpt-1α expression was significantly downregulated in a concentration-dependent manner (P < 0.01). Second, the expression of CHO metabolism-related genes was assessed (Fig. 9C and D). CQD exposure upregulated the expression of 3-hydroxy-3-methylglutaryl-coA reductase (hmgcr) and farnesoid X receptor (fxr) and downregulated the expression of cholesterol-7alpha-hydroxylase (cyp7a1) and cholesterol-27 alpha-hydroxylase (cyp27a1) in the intestine and liver (P < 0.01). The mttp and cpt-1α expression was upregulated in the intestine, and ATP-binding cassette subfamily A member 1 (abca1), ATP-binding cassette subfamily G member 8 (abcg8), and fibroblast growth factor 19 (fgf19) expression was downregulated in the liver (P < 0.01), whereas the opposite was true in the liver.
3.7 Correlation between the gut microbiota and toxicological responses after CQD exposure
Significant correlations were observed between intestine and liver injury-related indicators (i.e., SOD, GSH, MDA, nrf2, ho-1, tlr4, myd88, nf-κB/p65, il-1β, and tnf-α) and the gut microbiota (i.e., Cetobacterium, norank_f__Barnesiellaceae, Rhodobacter, and Roseomonas) in the heatmap (Fig. 10A). Cetobacterium, norank_f__Barnesiellaceae, and Rhodobacter were positively correlated with nrf2, ho-1, SOD, and GSH levels in the intestine and liver (R > 0.5, P < 0.01 or P < 0.05) but negatively correlated with tlr4, myd88, nf-κB/p65, il-1β, and tnf-α levels and MDA content (R < −0.5, P < 0.01 or P < 0.05). Roseomonas was strongly positively correlated with tlr4, myd88, nf-κB/p65, il-1β, and tnf-α levels and MDA content (R > 0.7, P < 0.01 or P < 0.05) and negatively correlated with nrf2, ho-1, SOD, and GSH levels (R < −0.7, P < 0.01 or P < 0.05). This indicated that Roseomonas may cause toxic effects in the intestine and liver. Spearman correlation was performed between the lipid metabolism parameters and the top 30 intestinal microorganisms (Fig. 10B). The relative abundance of Cetobacterium, norank_f__Barnesiellaceae, and Rhodobacter was significantly positively correlated with serum TG, CHO, HDL-C, and LDL-C levels; intestinal fxr, cyp27a1, abcga1, and fgf19 mRNA levels; and liver cpt-1α, cyp7a1, mttp, and cyp27a1 mRNA levels (P < 0.05). However, their relative abundance was significantly negatively correlated with intestinal cpt-1α, srebp-1c, mttp, hmgcr, and fas mRNA levels and liver fxr, srebp-1c, fas, abcg1, abcg8, fgf19, and hmgcr mRNA levels (P < 0.05). Roseomonas showed the opposite correlation, suggesting that it caused intestinal and liver damage.
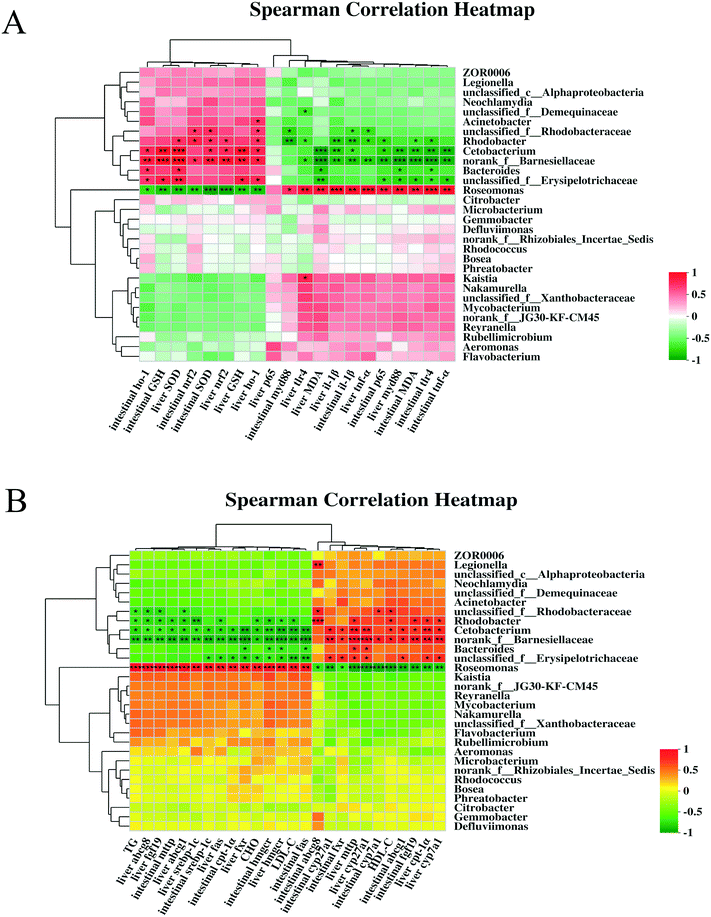 |
| Fig. 10 Correlation analysis between bacterial genera abundance with antioxidant and immune–inflammatory parameters (A). Correlation analysis between bacterial genera abundance and lipid metabolism parameters (B). | |
4. Discussion
The applications of manufactured nanomaterials are growing exponentially, and so is the research on the possible consequences of intentional or unintentional release of nanomaterials into the environment. However, there is a lack of information on the ecological impact and risk of carbon nanomaterials, in general, and on the aquatic ecotoxicity of CQDs, in particular.23 The present study provides insights into the effects of CQD toxicity on the gut–liver axis and gut microbiota of common carp, as well as explores the lipid metabolism disorders induced by CQD exposure and the potential role of the gut–liver axis in intestinal and liver damage.
Ex vivo imaging results showed that the CQD fluorescent signals gradually disappeared in carp within 2 weeks of a single oral dose, indicating that CQDs were excreted gradually and were only distributed in the intestine and liver after 2 weeks (Fig. 2). Quantum dots can cross the fish intestinal barrier and accumulate in various tissues upon sequential doses.24 In this study, CQD accumulation was observed by the fluorescence distribution in intestinal and liver tissues after multiple oral doses for 5 weeks. Moreover, higher CQD concentrations may lead to higher CQD accumulation in the organism (Fig. 2). These results confirm that CQDs possess high penetrability and can easily cross physical barriers.25 Histological analysis showed that CQD exposure caused intestinal and liver damage, which was manifested by reduced fold height and muscle thickness and increased villi width in the intestine, and swelling and cytoplasmic vacuolization of hepatocytes in the liver. This result was similar to previous studies. In zebrafish exposed to CQDs, the intestine showed cupped cell proliferation, microvilli shedding, and cell necrosis, whereas the liver showed abnormal hepatocyte morphology, nuclear consolidation, vacuole formation, and cell rupture, with high doses even leading to massive liver necrosis.25 Therefore, CQDs could directly penetrate the intestinal barrier or enter the blood via the enterohepatic circulation through the portal vein. In addition, CQD accumulation in the intestine and liver can directly induce toxicity.
CQD exposure induces oxidative stress in cells, animals, and plants.9,26,27 In the present study, SOD and GSH levels were significantly reduced and MDA content was significantly increased in carp intestinal and liver tissues after 5 week CQD exposure at 20 mg kg−1 BW, indicating that CQDs induced oxidative damage in the intestine and liver. In addition, nrf2, a master regulator of redox-sensitive transcription factors, plays a key role in redox homeostasis.28 The activation of nrf2 can trigger the transcription of downstream proteins to protect cells from oxidative damage, which is an important protective mechanism against oxidative stress.23,29 The nrf2/ho-1 pathway plays an essential role in combating oxidative stress. Here, the expression levels of nrf2 and its downstream gene ho-1 were significantly decreased, further indicating the induction of oxidative stress. This may be because CQDs stimulated the expression of endogenous antioxidant enzyme genes early on to resist adverse stress, but these antioxidant enzymes rapidly depleted and the corresponding gene expression levels were suppressed by persistent CQD exposure. Moreover, oxidative stress was the main mechanism of QD toxicity.30 Combined with changes in inflammatory and lipid metabolism parameters, it was evident that oxidative stress is at the root of subsequent pathological events.
Tight junction proteins (i.e., occludin, claudin, and zonula occludens-1) are important components of the intestinal mucosal barrier,31 and the disruption of tight junctions within the intestinal barrier increases intestinal permeability.32 In this study, CQD exposure suppressed the expression of occludin, claudin-2, and zonula occludens-1, indicating that it could impair the intestinal physical barrier function in carp, resulting in increased intestinal permeability. This result was consistent with the morphological assessment of the intestinal structure. Increased intestinal permeability and bacterial translocation can facilitate microbial metabolite entry into the liver, leading to impaired bile acid metabolism and promoting systemic inflammation.33
The innate immune system is the first line of defense against invasive pathogens. Toll-like receptors (TLRs) are widely recognized as upstream membrane recognition receptors in innate immune cells and are used to detect pathogen-related biochemical molecules and chemical substances.34 TLRs (other than TLR3) can activate NF-κB by mediating myd88 to enhance the formation of pro-inflammatory factors such as TNF-α, IL-1β, and IL-6.35 CQD exposure upregulated the gene expression of tlr4, myd88, and downstream inflammatory factors (i.e., il-1β and tnf-α) in the intestine and liver. These data indicated that CQDs can induce immune–inflammatory responses, resulting in intestinal and hepatic toxicity. Hence, the intestinal and hepatic toxicity caused by long-term CQD oral administration may be mainly through indirect mechanisms, such as oxidative stress and inflammatory responses.
In addition, inflammatory mediators are associated with abnormal lipid metabolism.36 Inflammatory cytokines can affect CHO hydroxylase activity, which can increase CHO levels by inhibiting the CHO catabolic pathway.37 In the present study, the TG, CHO, HDL-C, and LDL-C levels in carp serum were measured to determine whether CQDs affect lipid metabolism. In the CQD treatment groups, the TG, CHO, and LDL-C levels were significantly high, but the HDL-C levels were significantly low. HDL-C plays a vital role in transporting LDL-C, TG, and CHO from the peripheral cells and blood vessels to the liver for excretion and catabolism.38 Low HDL-C levels may lead to increased serum LDL-C, TG, and CHO levels. These results indicate that CQD exposure interfered with lipid metabolism. Chronic inflammatory stimulation also leads to the disruption of lipid metabolism in hepatocytes.36 In addition, CQDs induced TG accumulation by mainly enhancing fatty acid biosynthesis.17 In the present study, CQD exposure led to changes in TG metabolism. The expression of srebp-1c, which regulates TG synthesis, and fas was significantly upregulated in the intestine and liver. In addition, the expression of cpt-1α, which transports MTTP and is involved in fatty acid β-oxidation, was significantly upregulated in the gut but downregulated in the liver. These results explain the possible molecular mechanisms underlying the increased TG levels. Furthermore, CQD exposure led to altered CHO metabolism; for example, the expression of the CHO synthesis genes hmgcr and fxr was upregulated and that of the CHO degradation genes cyp7a1 and cyp27a1 was significantly downregulated in the intestine and liver, but the expression of the CHO transport genes abca1, abcg8, and fgf19 was downregulated in the intestine and upregulated in the liver. These results explain the increased CHO levels. Inhibition of CHO catabolism would mean that bile acid synthesis was blocked. However, bile acids function as essential mediators in the gut–liver axis. Reduced bile acid flow could lead to abnormal intestinal flora and an increase in secondary bile acids. This could further lead to a reduction in intestinal FXR signaling, impairing mucus and antimicrobial peptide synthesis and intestinal vascular barrier integrity, and promoting bacterial permeability and pathogen interaction with mucosal immune cells, which led to intestinal inflammation.39 Therefore, CQD exposure could disrupt the function of the gut–liver axis.
Orally ingested CQD antimicrobial activity occurred primarily in the gut; thus, CQDs may affect the gut microbiota. A change in the gut microbiota composition is associated with disease etiology.40 Gut microbiota richness can reflect the physiological status of the organism,41 and gut microbiota diversity can be used to assess gut inflammatory conditions.42 In this study, the values of Chao1 and Shannon indices were significantly low in the CQD treatment groups, indicating that CQD exposure may lead to changes in the physiological conditions and affect the inflammatory response in carp. Similar to other fish, the gut microbiota of carp mainly includes Proteobacteria, Actinobacteria, Fusobacteria, and Bacteroidetes.43,44
CQD exposure resulted in significant changes in the gut microbiota composition at the phylum level. In addition, treatment with low CQD concentrations can significantly increase Firmicutes, a gut bacterial community in carp and humans.45 The presence of Firmicutes is related to disease etiology (e.g., obesity) in humans.46 An increase in these bacteria may also cause metabolic disorders in fish and humans. However, studies have shown that Firmicutes mainly provides nutrition and energy to epithelial and gastrointestinal cells, improves mucus production, acts as an anticancer and anti-inflammatory agent, and its consumption may damage intestinal barrier integrity.47 Consistent with previously demonstrated results, high CQD concentrations significantly reduced Firmicutes, indicating that CQD exposure may disrupt the intestinal barrier.
The abundance of Rhodobacter was lower in the CQD treatment groups than in the control group at the genus level. Rhodobacter is a potential probiotic and contributes to the growth of grass carp (Ctenopharyngodon idella).48 Infection with cyprinid herpesvirus 2 caused a significant reduction in Rhodobacter in the gut of gibel carp (Carassius gibelio), indicating that Rhodobacter is important for disease resistance.49Cetobacterium, a probiotic prevalent in the gut of freshwater fish, produces vitamin B12 and is associated with nutrient absorption in fish.50 Its abundance in the gut of Nile tilapia (Oreochromis niloticus) was reduced after cadmium exposure.51 In this study, the relative abundance of Cetobacterium in the gut decreased after CQD exposure. Hence, changes in the gut microbiota caused by CQD exposure may affect normal nutrient absorption in the carp intestine. Compared with the control group, mycobacterial abundance in the high concentration CQD treatment group (i.e., C2) was significantly high (P < 0.05). Mycobacterium can cause necrosis of the skin and bones in humans and fish.52 Thus, CQD exposure could decrease probiotics in the gut of fish and cause infection by an increase in pathogenic microorganisms.
The gut microbiota regulates immunity and lipid metabolism via the gut–liver axis.53,54 In the carp intestine, the beneficial microbiota, including bacterial species from Cetobacterium, norank_f__Barnesiellaceae, and Rhodobacter, was positively associated with antioxidant parameters but negatively related to lipid metabolism-related genes, lipid peroxidation, and immune–inflammatory parameters (P < 0.05; Fig. 10). Roseomonas displayed an opposite correlation, which was similar to previous studies. The fermentation products of Cetobacterium can improve gut and liver health and reduce lipid deposition in carp.55 The decrease in Barnesiellaceae causes impairment of immune function.56,57Rhodobacter extracts (e.g., lycogen) activate immunity and prolong survival in mice with severe colitis.58 Thus, these potential probiotics play a significant role in promoting the proper functioning of the gut–liver axis. However, Roseomonas, a conditional pathogen,59 has not been studied for its harmful effects on fish health. In the present study, Roseomonas was considered an important factor contributing to an impaired gut–liver axis. In addition, the PICRUSt analysis based on the clusters of orthologous groups revealed the highest category as “lipid transport and metabolism” (Fig. S2†). Although most mechanisms are not yet known, the correlations between these factors may inform further studies.
5. Conclusions
After 5 weeks of CQD exposure by oral administration, oxidative stress induced by CQDs triggered an inflammatory response and eventually led to lipid metabolism disorders, resulting in intestine and liver damage. Changes in the gut microbiota of carp were associated with increased antioxidant capacity, immune response, and lipid metabolism, whereas the gut–liver axis may play an essential role in the underlying mechanisms. However, in-depth studies are required to determine whether the damage to host health is due to changes in the gut microbiota or the direct effects of CQD exposure. The results of this study provide reliable data for the toxicological risk assessment of CQDs in an ecological context.
Author contributions
Jianjun Chen: writing – review & editing, project administration, and funding acquisition. Dandan Sun: writing – original draft, data curation, formal analysis, conceptualization, methodology, and software. Han Cui, Chenyang Rao, Lulu Li, Suqi Guo, and Shuai Yang: sampling, lab measurements, and data analysis. Yuru Zhang: investigation and validation. Xianglin Cao: writing – review & editing and supervision.
Conflicts of interest
There are no conflicts to declare.
Acknowledgements
This work was supported by the Henan Province Science and Technology Development Plan Project in China (no. 212102110359).
References
- G. E. LeCroy, S. T. Yang, F. Yang, Y. M. Liu, K. A. S. Fernando, C. E. Bunker, Y. Hu, P. G. Luo and Y. P. Sun, Functionalized carbon nanoparticles: Syntheses and applications in optical bioimaging and energy conversion, Coord. Chem. Rev., 2016, 320, 66–81 CrossRef.
- S. T. Yang, X. Wang, H. F. Wang, F. S. Lu, P. J. G. Luo, L. Cao, M. J. Meziani, J. H. Liu, Y. F. Liu, M. Chen, Y. P. Huang and Y. P. Sun, Carbon Dots as Nontoxic and High-Performance Fluorescence Imaging Agents, J. Phys. Chem. C, 2009, 113, 18110–18114 CrossRef CAS.
- J. Peng, W. Gao, B. K. Gupta, Z. Liu, R. Romero-Aburto, L. H. Ge, L. Song, L. B. Alemany, X. B. Zhan, G. H. Gao, S. A. Vithayathil, B. A. Kaipparettu, A. A. Marti, T. Hayashi, J. J. Zhu and P. M. Ajayan, Graphene Quantum Dots Derived from Carbon Fibers, Nano Lett., 2012, 12, 844–849 CrossRef CAS PubMed.
- V. N. Mochalin and Y. Gogotsi, Wet Chemistry Route to Hydrophobic Blue Fluorescent Nanodiamond, J. Am. Chem. Soc., 2009, 131, 4594–4595 CrossRef CAS PubMed.
- X. T. Zheng, A. Ananthanarayanan, K. Q. Luo and P. Chen, Glowing Graphene Quantum Dots and Carbon Dots: Properties, Syntheses, and Biological Applications, Small, 2015, 11, 1620–1636 CrossRef CAS PubMed.
- Y. D. Li, X. K. Xu, Y. Wu, J. L. Zhuang, X. J. Zhang, H. R. Zhang, B. F. Lei, C. F. Hu and Y. L. Liu, A review on the effects of carbon dots in plant systems, Mater. Chem. Front., 2020, 4, 437–448 RSC.
- Y. F. Kang, Y. H. Li, Y. W. Fang, Y. Xu, X. M. Wei and X. B. Yin, Carbon Quantum Dots for Zebrafish Fluorescence Imaging, Sci. Rep., 2015, 5, 11835 CrossRef.
- W. Liu, G. Huang, X. Su, S. Li, Q. Wang, Y. Zhao, Y. Liu, J. Luo, Y. Li, C. Li, D. Yuan, H. Hong, X. Chen and T. Chen, Zebrafish: A Promising Model for Evaluating the Toxicity of Carbon Dot-Based Nanomaterials, ACS Appl. Mater. Interfaces, 2020, 12, 49012–49020 CrossRef CAS PubMed.
- Y. Y. Xiao, L. Liu, Y. Chen, Y. L. Zeng, M. Z. Liu and L. Jin, Developmental Toxicity of Carbon Quantum Dots to the Embryos/Larvae of Rare Minnow (Gobiocypris rarus), BioMed Res. Int., 2016, 2016, 4016402 Search PubMed.
- X. B. Sun, X. Z. Jin, W. Pan, E. M. Guo, W. J. Liu, D. H. Li, K. C. Lu, S. X. Si, N. X. Zhang, Z. Z. Jia, Y. P. Shi, Q. D. Li and J. P. Wang, Highly Luminescent Carbon Dots Synthesized by Microwave-Assisted Pyrolysis and Evaluation of Their Toxicity to Physa acuta, J. Nanosci. Nanotechnol., 2016, 16, 648–653 CrossRef CAS.
- M. L. Zhang, H. B. Wang, P. P. Liu, Y. X. Song, H. Huang, M. W. Shao, Y. Liu, H. Li and Z. H. Kang, Biotoxicity of degradable carbon dots towards microalgae Chlorella vulgaris, Environ. Sci.: Nano, 2019, 6, 3316–3323 RSC.
- G. Rafiee, K. R. Tavabe, M. Frinsko and H. Daniels, Effects of various sodium adsorption ratio (SAR) mediums on larval performance of the freshwater prawn Macrobrachium rosenbergii (de Man), Aquacult. Res., 2015, 46, 725–735 CrossRef CAS.
- S. Vali, G. Mohammadi, K. R. Tavabe, F. Moghadas and S. S. Naserabad, The effects of silver nanoparticles (Ag-NPs) sublethal concentrations on common carp (Cyprinus carpio): Bioaccumulation, hematology, serum biochemistry and immunology, antioxidant enzymes, and skin mucosal responses, Ecotoxicol. Environ. Saf., 2020, 194, 110353 CrossRef CAS.
- A. Tripathi, J. Debelius, D. A. Brenner, M. Karin, R. Loomba, B. Schnabl and R. Knight, The gut-liver axis and the intersection with the microbiome, Nat. Rev. Gastroenterol. Hepatol., 2018, 15, 397–411 CrossRef CAS.
- P. Kanmani, K. Suganya and H. Kim, The Gut Microbiota: How Does It Influence the Development and Progression of Liver Diseases, Biomedicines, 2020, 8, 501 CrossRef CAS.
- Y. X. Jin, S. S. Wu, Z. Y. Zeng and Z. W. Fu, Effects of environmental pollutants on gut microbiota, Environ. Pollut., 2017, 222, 1–9 CrossRef CAS.
- Q. Q. Zhang, R. Shi, Q. Li, T. Maimaiti, S. K. Lan, O. Y. Peng, B. W. Ouyang, Y. T. Bai, B. W. Yu and S. T. Yang, Low toxicity of fluorescent carbon quantum dots to white rot fungus Phanerochaete chrysosporium, J. Environ. Chem. Eng., 2021, 9, 104633 CrossRef CAS.
- T. Du, J. G. Liang, N. Dong, L. Liu, L. R. Fang, S. B. Xiao and H. Y. Han, Carbon dots as inhibitors of virus by activation of type I interferon response, Carbon, 2016, 110, 278–285 CrossRef CAS.
- X. L. Dong, W. X. Liang, M. J. Meziani, Y. P. Sun and L. J. Yang, Carbon Dots as Potent Antimicrobial Agents, Theranostics, 2020, 10, 671–686 CrossRef CAS.
- C. Fu, L. Liu and F. Li, Acetate alters the process of lipid metabolism in rabbits, Animal, 2018, 12, 1895–1902 CrossRef CAS PubMed.
- D. X. Li, J. Qin, Q. Xu and G. Q. Yan, A room-temperature phosphorescence sensor for the detection of alkaline phosphatase activity based on Mn-doped ZnS quantum dots, Sens. Actuators, B, 2018, 274, 78–84 CrossRef CAS.
- P. Romani, I. Brian, G. Santinon, A. Pocaterra, M. Audano, S. Pedretti, S. Mathieu, M. Forcato, S. Bicciato, J. B. Manneville, N. Mitro and S. Dupont, Extracellular matrix mechanical cues regulate lipid metabolism through Lipin-1 and SREBP, Nat. Cell Biol., 2019, 21, 338–347 CrossRef CAS.
- J. F. Luo, X. Y. Shen, C. K. Lio, Y. Dai, C. S. Cheng, J. X. Liu, Y. D. Yao, Y. Yu, Y. Xie, P. Luo, X. S. Yao, Z. Q. Liu and H. Zhou, Activation of Nrf2/HO-1 Pathway by Nardochinoid C Inhibits Inflammation and Oxidative Stress in Lipopolysaccharide-Stimulated Macrophages, Front. Pharmacol., 2018, 9, 911 CrossRef PubMed.
- C. M. Lavelle, J. H. Bisesi, M. A. Hahn, K. J. Kroll, T. Sabo-Attwood and D. Denslow, Oral bioavailability and sex specific tissue partitioning of quantum dots in fathead minnows, Environ. Sci.: Nano, 2015, 2, 583–593 RSC.
- K. Yao, X. H. Lv, G. Q. Zheng, Z. H. Chen, Y. L. Jiang, X. S. Zhu, Z. Y. Wang and Z. H. Cai, Effects of Carbon Quantum Dots on Aquatic Environments: Comparison of Toxicity to Organisms at Different Trophic Levels, Environ. Sci. Technol., 2018, 52, 14445–14451 CrossRef CAS PubMed.
- T. Zhang, J. Qu, Y. Yao, Y. E. Zhang, Y. Ma, D. R. N. Wu, Y. N. Cao, M. R. Yang, Y. J. Zhang, M. Tang and Y. P. Pu, N-doped carbon dots triggered the induction of ROS-mediated cytoprotective autophagy in Hepal-6 cells, Chemosphere, 2020, 251, 126440 CrossRef CAS.
- J. Chen, R. Dou, Z. Yang, X. Wang, C. Mao, X. Gao and L. Wang, The effect and fate of water-soluble carbon nanodots in maize (Zea mays L.), Nanotoxicology, 2016, 10, 818–828 CrossRef CAS PubMed.
- L. Y. Wang, R. F. Fan, D. B. Yang, D. Zhang and L. Wang, Puerarin reverses cadmium-induced lysosomal dysfunction in primary rat proximal tubular cells via inhibiting Nrf2 pathway, Biochem. Pharmacol., 2019, 162, 132–141 CrossRef CAS PubMed.
- M. U. Rehman, S. Rashid, A. Arafah, W. Qamar, R. M. Alsaffar, A. Ahmad, N. M. Almatroudi, S. M. A. Alqahtani, S. M. Rashid and S. B. Ahmad, Piperine Regulates Nrf-2/Keap-1 Signalling and Exhibits Anticancer Effect in Experimental Colon Carcinogenesis in Wistar Rats, Biology, 2020, 9, 302 CrossRef CAS PubMed.
- K. G. Li, J. T. Chen, S. S. Bai, X. Wen, S. Y. Song, Q. Yu, J. Li and Y. Q. Wang, Intracellular oxidative stress and cadmium ions release induce cytotoxicity of unmodified cadmium sulfide quantum dots, Toxicol. In Vitro, 2009, 23, 1007–1013 CrossRef CAS PubMed.
- G. J. Yu, W. H. Ou, Z. B. Liao, H. G. Xu, M. Q. Liang, Y. J. Zhang and K. S. Mai, Intestinal homeostasis of juvenile tiger puffer Takifugu rubripes was sensitive to dietary arachidonic acid in terms of mucosal barrier and microbiota, Aquaculture, 2019, 502, 97–106 CrossRef CAS.
- L. Niklasson, H. Sundh, F. Fridell, G. L. Taranger and K. Sundell, Disturbance of the intestinal mucosal immune system of farmed Atlantic salmon (Salmo salar), in response to long-term hypoxic conditions, Fish Shellfish Immunol., 2011, 31, 1072–1080 CrossRef CAS PubMed.
- I. Milosevic, A. Vujovic, A. Barac, M. Djelic, M. Korac, A. R. Spurnic, I. Gmizic, O. Stevanovic, V. Djordjevic, N. Lekic, E. Russo and A. Amedei, Gut-Liver Axis, Gut Microbiota, and Its Modulation in the Management of Liver Diseases: A Review of the Literature, Int. J. Mol. Sci., 2019, 20, 395 CrossRef PubMed.
- K. A. Fitzgerald and J. C. Kagan, Toll-like Receptors and the Control of Immunity, Cell, 2020, 180, 1044–1066 CrossRef CAS PubMed.
- Z. Gu, R. Jia, Q. He, L. Cao, J. Du, W. Feng, G. Jeney, P. Xu and G. Yin, Alteration of lipid metabolism, autophagy, apoptosis and immune response in the liver of common carp (Cyprinus carpio) after long-term exposure to bisphenol A, Ecotoxicol. Environ. Saf., 2021, 211, 111923 CrossRef CAS.
- V. A. Sevastianos, T. A. Voulgaris and S. P. Dourakis, Hepatitis C, systemic inflammation and oxidative stress: correlations with metabolic diseases, Expert Rev. Gastroenterol. Hepatol., 2019, 14, 27–37 CrossRef.
- G. Zhang, L. Qiu, W. Li, Y. Chen, L. Wang, Z. J. M. Wei and C. Biochemistry, Interleukin-1β enhances the intracellular accumulation of cholesterol by up-regulating the expression of low-density lipoprotein receptor and 3-hydroxy-3-methylglutaryl coenzyme A reductase in podocytes, Mol. Cell. Biochem., 2011, 346, 197–204 CrossRef CAS.
- A. S. Shah, L. Tan, J. L. Long and W. S. Davidson, Thematic Review Series: High Density Lipoprotein Structure, Function, and Metabolism Proteomic diversity of high density lipoproteins: our emerging understanding of its importance in lipid transport and beyond, J. Lipid Res., 2013, 54, 2575–2585 CrossRef CAS PubMed.
- A. Albillos, A. de Gottardi and M. Rescigno, The gut-liver axis in liver disease: Pathophysiological basis for therapy, J. Hepatol., 2020, 72, 558–577 CrossRef CAS.
- K. Lange, M. Buerger, A. Stallmach and T. Bruns, Effects of Antibiotics on Gut Microbiota, J. Dig. Dis., 2016, 34, 260–268 CrossRef.
- H. X. Gu, S. X. Wang, X. H. Wang, X. Yu, M. H. Hu, W. Huang and Y. J. Wang, Nanoplastics impair the intestinal health of the juvenile large yellow croaker Larimichthys crocea, J. Hazard. Mater., 2020, 397, 122773 CrossRef CAS.
- S. J. Ott, M. Musfeldt, D. F. Wenderoth, J. Hampe, O. Brant, U. R. Folsch, K. N. Timmis and S. Schreiber, Reduction in diversity of the colonic mucosa associated bacterial microflora in patients with active inflammatory bowel disease, Gut, 2004, 53, 685–693 CrossRef CAS.
- T. T. Li, M. Long, F. J. Gatesoupe, Q. Q. Zhang, A. H. Li and X. N. Gong, Comparative Analysis of the Intestinal Bacterial Communities in Different Species of Carp by Pyrosequencing, Microb. Ecol., 2015, 69, 25–36 CrossRef CAS.
- G. Roeselers, E. K. Mittge, W. Z. Stephens, D. M. Parichy, C. M. Cavanaugh, K. Guillemin and J. F. Rawls, Evidence for a core gut microbiota in the zebrafish, ISME J., 2011, 5, 1595–1608 CrossRef CAS.
- X. M. Li, Q. Y. Yan, S. Q. Xie, W. Hu, Y. H. Yu and Z. H. Hu, Gut Microbiota Contributes to the Growth of Fast-Growing Transgenic Common Carp (Cyprinus carpio L.), PLoS One, 2013, 8, e64577 CrossRef CAS.
- N. A. Ismail, S. H. Ragab, A. Abd ElBaky, A. R. S. Shoeib, Y. Alhosary and D. Fekry, Frequency of Firmicutes and Bacteroidetes in gut microbiota in obese and normal weight Egyptian children and adults, Arch. Med. Sci., 2011, 7, 501–507 CrossRef CAS.
- E. Collinder, M. E. Cardona, G. N. Berge, E. Norin, S. Stern and T. Midtvedt, Influence of zinc bacitracin and Bacillus licheniformis on microbial intestinal functions in weaned piglets, Vet. Res. Commun., 2003, 27, 513–526 CrossRef CAS PubMed.
- E. M. Yu, J. Xie, J. L. Wang, H. Ako, G. J. Wang, Z. H. Chen and Y. F. Liu, Surface-attached and suspended bacterial community structure as affected by C/N ratios: relationship between bacteria and fish production, World J. Microbiol. Biotechnol., 2016, 32, 116 CrossRef PubMed.
- S. Rong, T. T. Li, D. Luo, J. B. Li and Q. G. J. C. M. Yan, Changes in the Intestinal Microbiota of Gibel Carp (Carassius gibelio) Associated with Cyprinid herpesvirus 2 (CyHV-2) Infection, Curr. Microbiol., 2017, 74, 1130–1136 CrossRef PubMed.
- C. Tsuchiya, T. Sakata and H. Sugita, Novel ecological niche of Cetobacterium somerae, an anaerobic bacterium in the intestinal tracts of freshwater fish (vol 46, pg 43, 2008), Lett. Appl. Microbiol., 2008, 46, 282–282 CrossRef CAS.
- Q. X. Zhai, L. L. Yu, T. Q. Li, J. M. Zhu, C. C. Zhang, J. X. Zhao, H. Zhang and W. Chen, Effect of dietary probiotic supplementation on intestinal microbiota and physiological conditions of Nile tilapia (Oreochromis niloticus) under waterborne cadmium exposure, Anton. Leeuw. Int. J. G., 2017, 110, 501–513 CrossRef CAS PubMed.
- M. Ucko and A. Colorni, Mycobacterium marinum infections in fish and humans in Israel, J. Clin. Microbiol., 2005, 43, 892–895 CrossRef CAS PubMed.
- R. Wang, R. Q. Tang, B. Li, X. Ma, B. Schnabl and H. Tilg, Gut microbiome, liver immunology, and liver diseases, Cell. Mol. Immunol., 2021, 18, 4–17 CrossRef CAS PubMed.
- S. Z. Wang, Y. J. Yu and K. Adeli, Role of Gut Microbiota in Neuroendocrine Regulation of Carbohydrate and Lipid Metabolism via the Microbiota-Gut-Brain-Liver Axis, Microorganisms, 2020, 8, 527 CrossRef CAS PubMed.
- C. Mxa, Z. A. Wei, A. Yx, L. A. Yu, Z. B. Zhen, B. Yy, C. Reo, R. B. Chao and Z. A. J. A. Zhuang, Effects of Cetobacterium somerae fermentation product on gut and liver health of common carp (Cyprinus carpio) fed diet supplemented with ultra-micro ground mixed plant proteins, Aquaculture, 2021, 543, 736943 CrossRef.
- E. A. Carlson, Y. Li and J. T. Zelikoff, Benzo[a]pyrene-induced immunotoxicity in Japanese medaka (Oryzias latipes): relationship between lymphoid CYP1A activity and humoral immune suppression, Toxicol. Appl. Pharmacol., 2004, 201, 40–52 CrossRef CAS PubMed.
- S. Reynaud and P. Deschaux, The effects of polycyclic aromatic hydrocarbons on the immune system of fish: a review, Aquat. Toxicol., 2006, 77, 229–238 CrossRef CAS PubMed.
- W. S. Liu, M. C. Chen, K. H. Chiu, Z. H. Wen and C. H. Lee, Amelioration of dextran sodium sulfate-induced colitis in mice by Rhodobacter sphaeroides extract, Molecules, 2012, 17, 13622–13630 CrossRef CAS PubMed.
- J. D. Rihs, D. J. Brenner, R. E. Weaver, A. G. Steigerwalt, D. G. Hollis and V. L. Yu, Roseomonas, a new genus associated with bacteremia and other human infections, J. Clin. Microbiol., 1994, 31, 3275–3283 CrossRef PubMed.
Footnote |
† Electronic supplementary information (ESI) available. See DOI: 10.1039/d1en00651g |
|
This journal is © The Royal Society of Chemistry 2022 |