Bi2O3/TiO2@reduced graphene oxide with enzyme-like properties efficiently inactivates Pseudomonas syringae pv. tomato DC3000 and enhances abiotic stress tolerance in tomato†
Received
18th June 2021
, Accepted 21st September 2021
First published on 1st October 2021
Abstract
This study aimed to evaluate the potential role of Bi2O3/TiO2@reduced graphene oxide (rGO) in the antibacterial activity of the typical plant pathogenic bacteria Pst.DC3000 and the enhancement of tomato resistance to pathogenic bacteria. The in vitro antibacterial test showed that 4%rGO-(Bi2O3–TiO2) with a minimum inhibitory concentration of 1280 μg mL−1 had an inactivation efficiency of 6
log (>99.9999%) for Pst.DC3000 within 150 min under visible light conditions, which far exceeded the other types of nanomaterials under study. Its good peroxidase-like and superoxide dismutase-like characteristics and ability to tightly bind to the lipopolysaccharide of the bacterial outer membrane made it adhere to the bacterial surface and produce a large amount of reactive oxygen species [˙O2−, hydroxyl (˙OH) and H2O2] for in situ sterilization. This feature changed the permeability of the cell membrane and significantly down-regulated the virulence genes (hrpS, corS, iaaL and flgG), destroying the pathogenicity of the bacteria. Incredibly, 1280 μg mL−1 (100 μL per 1 g leaf) 4%rGO-(Bi2O3–TiO2) foliar spray substantially alleviated the pathogenicity of Pst.DC3000, and both the growth and photosynthetic function were improved. Simultaneously, the increase in thiols and defense-related enzyme activities (PPO, PAL and POD increased to 4.5, 30 and 10 U g−1 FW) indicated that 4%rGO-(Bi2O3–TiO2) significantly enhanced the tomato resistance to Pst.DC3000, and the 2.39- and 3.35-fold up-regulation of PR-2 and PR-13 gene expression verified the enhancement of disease resistance. In conclusion, in vitro and in vivo experiments showed that 4%rGO-(Bi2O3–TiO2) stood out in plant resistance and disease resistance and has potential application value in agricultural nanotechnology.
Environmental significance
Nanoparticles with enzyme-like properties are promising in nano-enabled agriculture. An in-depth understanding of the interaction between nanomaterials, pathogenic bacteria, and plants is essential for the sustainable application of nanotechnology in agriculture. Here, we demonstrated the significant antibacterial activity of 4%rGO-(Bi2O3–TiO2) nanomaterials at an appropriate dose (1280 μg mL−1) against tomato pathogenic bacteria Pst.DC3000 in vitro. In addition, foliar spraying of the nanomaterials significantly improved the growth and photosynthetic function of tomatoes, triggering the up-regulation of endogenous antioxidant and disease-resistant genes, making them an effective regulator for enhancing tomato stress tolerance.
|
Introduction
Various plant pathogenic microorganisms, such as fungi and bacteria, cause numerous plant diseases, hindering plant growth, affecting the yield, production quality, and food safety of fruits, vegetables and crops, and resulting in significant economic losses.1Pseudomonas syringae pv. tomato DC3000 (Pst.DC3000) is a model pathogen capable of infecting Arabidopsis and tomato (Solanum lycopersicum).2 Tomato is one of the most highly consumed crops and the second most important vegetable crop in the world, according to statistics from the World Health Organization (WHO).3 The pathogen Pst.DC3000 grows on the surface of tomato leaves and colonizes through natural stomata or wounds on the leaves under favorable conditions (such as cool and humid). After rapidly multiplying to high density, it causes many brown necrotic spots on the leaves and fruits, that is, bacterial speck disease.4 Bacterial speck disease reduces the quality of tomatoes and leads to a reduction in their yield to 75%,5 which has a substantial economic impact globally. Given the significant harm of bacterial speck disease and the economic importance of crops, finding control strategies with high antibacterial activity is necessary. Control measures for bacterial speck disease are currently concentrated on biological control and chemical control.6 Plants have a relatively comprehensive defense mechanism in response to pathogenic bacteria to prevent them from invading.7 Endophytes such as Bacillus pumilus and Bacillus amyloliquefaciens promote systemic plant protection and induce tomato resistance to Pst.DC3000, thereby controlling this disease.8 Meanwhile, the resistant tomato with Pti 4/5/6-overexpression was also separated to combat the bacterial speck disease.9 However, restrictions on labor, economic costs, and environmental adaptation are not conducive to applying biological control. Also, chemical control mainly depends on applying pesticides, antibiotics and copper-based compounds.5 However, long-term use of streptomycin and copper hydroxide led to the emergence of tolerant populations of phytopathogenic bacteria in crops, making drug efficacy weaker.10 Also, pesticide residues in soil and crops can create new ecological risks.11 In addition, excessive use of chemical fertilizers and pesticides can damage the ecosystem and public health. As a result, Europe has issued a directive, restricting the use of chemicals and antibiotics in the agricultural control of bacterial diseases.6 Traditional methods such as soil solarization, fumigation and crop rotation are available, but the control effect is not complete.12 Therefore, exploring sustainable agricultural alternative strategies is essential to ensure the healthy growth and development of plants, efficiently replacing environment-friendly antibacterial agents.
Nanomaterials with antibacterial properties provide potential solutions for preventing plant diseases. Recently, the fast-developing nanotechnology has been widely used in various industries.13 In agriculture, nanoparticles (NPs) have been successfully used in plant protection, pollutant removal, plant breeding, fertilization, biosensing, food packaging, crop health management and prevention of plant diseases.14,72,73 In plants, the excessive production of reactive oxygen species (ROS) under stress conditions prevents chloroplasts from maintaining a high photosynthesis rate.15 NPs with antioxidant enzyme-like properties, nanozymes, can inactivate microorganisms by catalyzing ROS production and use their antioxidant capacity to enhance plant photosynthesis and enhance its stress resistance.16 The uptake and transport of Fe3O4 NPs (500 mg L−1) in barley and soybean have been reported to promote plant growth, gene expression and effective photosynthesis.14 A study also pointed out that V2O5, Fe3O4, graphene and other peroxidase-like nanomaterials can catalyze the generation of hydroxyl radicals (˙OH) from H2O2, avoid the toxicity of H2O2 and also have antibacterial properties.15 Therefore, nanozymes are very promising drugs in controlling plant pathogens.
It was reported that an appropriate concentration (40–160 mg L−1) of titanium dioxide (TiO2) improved obviously spinach growth by activating photosynthesis.42 Many studies have claimed that the photocatalytic activity of TiO2 NPs protects plants from pathogenic fungi such as Mucor circinelloides, Fusarium solani, and F. oxysporum.17 The excellent possibilities of TiO2 for environmental protection and plant protection are due to its excellent light reaction characteristics.18,74 However, TiO2 is limited by its excitation light wavelength and low applicability. Therefore, it needs to be doped with raw materials to enhance its applicability. Bismuth oxide (Bi2O3) NPs, (2 mM, 50 μL) due to their large surface area, non-toxicity, biocompatibility and high chemical stability, have been reported in the effective sterilization of Candida albicans and Streptococcus mutans.19 rGO (1 mg mL−1) can inhibit the mycelial growth of fungal pathogens, such as Aspergillus niger and Aspergillus oryzae.20 rGO (0.1 mg mL−1) promotes plant seed germination and seedling growth, indicating that rGO is an ideal composite material.21 TiO2-modified materials applied in preventing plant pathogens and controlling plant diseases are still insufficient.
In this study, due to the various enzyme activities of Bi2O3/TiO2-rGO,22 antibacterial experiments of Pst.DC3000 were conducted in vitro including the optimization of antibacterial conditions and the combination of materials and bacteria. The effect of bacterial metabolism, survival, and gene expression was explained by the antibacterial mechanism. The nanozymes were sprayed on the leaves of tomatoes to investigate their effects on the plant growth, photosynthetic function, stress resistance, and disease resistance.
Experimental
Bi2O3/TiO2@rGO nanomaterial preparation and enzyme-like activity
All chemicals were of analytical grade and used without further purification. A three-step process was used to synthesize the nanomaterial composite according to previous research.22 Firstly, a composite (Bi2O3–TiO2) of TiO2 nanoparticles (P25, supplied by Evonik-Degussa Germany) with bismuth oxide was prepared by a solution combustion method. Secondly, graphite oxide (GO) was made from graphite (325 mesh, supplied by Henan Yixiang New Material Co., Ltd) by Hummers' method. In the third and final step, Bi2O3/TiO2@rGO was prepared by a slightly modified hydrothermal synthesis method. For the determination of the thiol concentration of glutathione (GSH), peroxidase (POD)-like properties and superoxide dismutase (SOD)-like properties, please refer to Method S1.† A constant light intensity lamp was used to illuminate the mixed solution at room temperature. All measurements were performed in triplicate.
Bacteria culture and in vitro antibacterial activity
Pst.DC3000 was employed as an antibacterial indicator. The optical density value was used to estimate the concentration of Pst.DC3000 at a wavelength of 600 nm (OD600 of 0.54 corresponded to 1010 CFU mL−1 by relating CFU–OD600 standard curves, please see Fig. S1†). According to the Kirby disc diffusion method, the zone of inhibition was determined. The antibacterial activity of the nanomaterials was tested by a colony counting method. The minimum inhibitory concentration (MIC) was used to determine the most suitable antibacterial conditions. For details, see Method S2.†
Interaction of bacteria and nanomaterials
To observe the interaction between Pst.DC3000 and nanomaterials, scanning electron microscopy (SEM) was used. The nanomaterials were dispersed and mixed with the bacterial suspension by ultrasonic treatment, and the zeta potential between the nanoparticles and the bacteria was measured with a Zetasizer Nano-Z (Malvern PANalytical Ltd, UK). The lipopolysaccharide (LPS) of Pst.DC3000 was extracted using an LPS extraction kit (iNtRON) according to the manufacturer's instructions. The nanomaterials were added into the LPS aqueous solution (1 mg mL−1 for both) and dispersed by ultrasonic treatment. The mixture was left at room temperature for 2 hours. The nanomaterials were collected by centrifugation and dried overnight at room temperature. The nanomaterials and KBr powder (infrared grade, Sigma-Aldrich) were mixed, and the adhesion of the LPS to the nanoparticles was studied by using Fourier transform infrared spectroscopy (FTIR, Shimadzu Co., Ltd., Japan) measurement.
Cytotoxicity assay
The bacterial suspension (10 mL, 1010 CFU mL−1) was mixed with different proportions of nanomaterials (10 mL, 1280 μg mL−1) in Petri dishes. The mixed solution was then incubated separately under visible light and dark conditions at 30 °C for 150 min and taken every 15 minutes for the following tests.
The relative conductivity of the bacteria was measured using a conductivity meter (DDS-307, INESA Scientific Instrument Co., Ltd, China) to evaluate the cell damage. The Bradford method was used to measure protein changes to evaluate changes in the cell membrane integrity. Bovine serum albumin (BSA) was maintained as the standard, and the results were expressed as μg of BSA per gram of sample, the standard curve is shown in Fig. S2.† For details, see Method S3 in the ESI.†
The cell viability was determined by the MTT reduction assay. The level of lipid peroxidation of the bacteria was determined by measuring the content of malondialdehyde (MDA) with thiobarbituric acid (TBA). FTIR was used to detect the molecular vibrations before and after the bacterial cell processing, to analyze the changes of biochemical components (lipids, carbohydrates, and proteins).
Moreover, a Holomonitor™ M4 microscope (Phase Holographic Imaging AB, Lund, Sweden) for digital holographic microscopy was utilized to observe and analyze the bacteria's morphological characteristics before and after treatment. The Holomonitor M4 is equipped with a ×20 magnification objective lens (NA-0.4) and 0.8 mW HeNe laser (633 nm, 100 μW cm−2, exposure time 5 ms). We focused on the area, irregularity, perimeter, roughness, thickness and volume and other parameter changes.
Biological effects of 4%rGO-(Bi2O3–TiO2) on tomato
Seed germination, plant growth and treatment.
For seed germination and plant cultivation, see Method S4.† The germination potential, germination rate and germination index of the seeds were measured in the dark for 6 days. After 2 weeks of normal growth, different concentrations (320, 640, 1280, 2560 μg mL−1) of nanomaterials were applied to tomato leaves by the foliar spray method, and 100 μL (equivalent to 32, 64, 128, 256 mg kg−1) was applied per 1 g of leaves. 10 mM MgCl2 and the bacterial suspension were mixed and sprayed evenly on the back of tomato leaves for inoculation and the surrounding environment was strictly controlled.
Disease control effect.
The control effect of the bacterial leaf spot caused by Pst.DC3000 on tomato directly reflected the 4%rGO-(Bi2O3–TiO2) antibacterial activity in vivo. When the tomato grew to the late seedling stage (3 real leaves), the same volume of nanomaterials at concentrations of 320, 640, 1280, and 2560 μg mL−1 was sprayed on the foliar surface. After 1 day, the spray Pst.DC3000 inoculation concentration was 1010 CFU mL−1. The lesion diameter and incidence rate at 5 dpi and 10 dpi were observed and the disease index and disease control effect were calculated.
Photosynthetic parameter determination and chlorophyll estimation.
After 5 days of nanomaterial foliar spray application, a portable photosynthesis system (3051D, Zhejiang Top Yunnong Technology Co., Ltd. China) was used to measure the net photosynthetic rate (Pn), stomatal conductance (Gs), transpiration rate (Tr) and water use efficiency (WUE). The second leaf of each treatment was placed in the leaf chamber of the instrument under the conditions that the photon flux (PPF) was 200 μmol m−2 s−1 and the carbon dioxide concentration was 375 μmol mol−1. The tomato leaves were cut into 1 cm sections, 0.2 g sample was taken into a 10 mL test tube with a stopper, 80% acetone extract was added to make the volume 10 mL, and the leaves were extracted in the dark until they turned white. The concentrations of chlorophyll a, chlorophyll b, and carotenoids were calculated by measuring the absorbance at 470, 646, and 663 nm wavelengths.
Assay of defense-related enzyme activities.
One day after spraying the nanomaterials, Pst.DC3000 (1010 CFU mL−1) was inoculated, and the untreated tomato plants were used as controls. The activity of phenylalanine ammonia-lyase (PAL), polyphenol oxidase (PPO) and peroxidase (POD) was measured. For specific operations, see Method S5.†
Gene expression analysis by qRT-PCR.
Quantitative real-time reverse-transcription polymerase chain reaction (qRT-PCR) was used to quantify the relative mRNA levels of the genes to be tested in the pathogen Pst.DC3000 and tomato leaves. The primer sequences used in the experiment are listed in Tables S1 and S2.† For pre-processing and specific parameters of the qRT-PCR reaction system, see Method S6.† Each sample was tested in triplicate. The relative expression data were calculated using the 2−ΔΔct method.23
Statistical analysis.
There were three repetitions for each experimental treatment, and the data were expressed as means ± standard deviation (SD). Duncan's multiple range test of one-way analysis of variance (ANOVA) was used to compare the significant level differences of the data (p < 0.05 and p < 0.01), using different capital letters within the group and different lowercase letters between groups, p < 0.01 used “**”, p < 0.05 used “*” to indicate the difference between data.
Results and discussion
Enzyme-like catalytic properties of nanomaterials
In this study, integrated biomarker response (IBRv2analysis was used to normalize the peroxidase (POD)-like properties, superoxide dismutase (SOD)-like properties, and glutathione (GSH) consumption (Fig. S3†) into a unified index to evaluate the comprehensive enzyme-like activity of the nanomaterials with different types and different concentrations. The comprehensive enzyme activity of nanomaterials with rGO (Fig. 1e–h) was higher than that of Bi2O3–TiO2 (Fig. 1a–d), and that of 4%rGO-(Bi2O3–TiO2) was the strongest, and the IBRv2 value was 6.33 under visible light. Fig. 1i–n show that as the concentration increased, the IBRv2 value of 4%rGO-(Bi2O3–TiO2) continued to increase to 8.89 under visible light. The POD-like activity among them increased significantly, which was presumed to be because of the addition of rGO. The POD-like activity was attributed to the generation of hydroxyl radicals (˙OH) in the presence of H2O2.24 A study reported that TiO2 could not catalyze the oxidation of 3,3′,5,5′-tetramethylbenzidine (TMB), but rGO itself had a better affinity for TMB.70 Moreover, compared with Pt NPs, which had stronger POD enzyme activity, rGO had an affinity to H2O2.25 Simultaneously, due to its high activity and biocompatibility, rGO was often used as a representative nanozyme to enhance the production of ˙OH in nanomaterials.26 As a reducing substance in bacteria, GSH could weaken the killing of bacteria caused by ROS.27 The results indicated that all nanomaterials had an enhanced GSH consumption ability under visible light compared with dark conditions and better antibacterial effects. The SOD-like activity eliminated excess oxide anion radicals (˙O2−) by dismutation of ˙O2− to H2O2 and O2.28 The results indicated that a large amount of ˙O2− was generated under the excitation of visible light, which generated H2O2 under SOD-like enzyme activity. H2O2 was converted into hydroxyl radicals under POD-like conditions, which accounted for the main enzyme activity characteristics so the system also generated a large amount of ˙O2− and ˙OH, which was consistent with our previous research results on the main effect of 4%rGO-(Bi2O3–TiO2) on free radicals.22 The IBRv2 index aggregated the response scores of multiple indicators into a single value and provided an intuitive explanation for the responding object's function. By weighting calculation, 4%rGO-(Bi2O3–TiO2) was seen to be excited by visible light (Fig. 1m and n) and had the most vital enzyme-like activity. The activity of 4%rGO-(Bi2O3–TiO2) became stronger with the increase in the dosage, leading to considerable antibacterial performance.
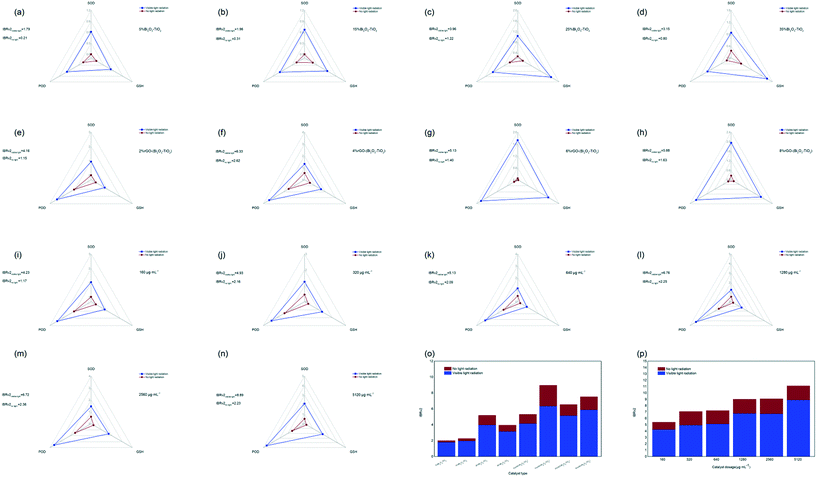 |
| Fig. 1 IBRv2 analysis of enzyme-like activity (a–p). | |
In vitro antibacterial activity of nanomaterials
4%rGO-(Bi2O3–TiO2) displayed more powerful antibacterial activity than the other nanomaterials (12.0 mm ZOI; Fig. S4†). The photographs of the Petri dish visualized the survival case of the bacteria. Fig. 2a shows that the distribution of colonies under visible light radiation was uneven, indicating the different antibacterial abilities of the nanomaterials. Among them, 4%rGO-(Bi2O3–TiO2) showed 1
log higher inactivation efficiency than Bi2O3–TiO2 (p < 0.01) (Fig. 2b), which was quantitatively evaluated by the plate counting method. After 150 min of incubation under visible light, no survival colonies were observed on the culture plate, which meant reaching 6
log (>99.9999%) inactivation efficiency (Fig. 2c and d). We tested the minimum inhibitory concentration of 4%rGO-(Bi2O3–TiO2), which was 1280 μg mL−1, to determine the most suitable antibacterial conditions for Pst.DC3000 (p < 0.05) (Fig. 2e). The SEM images (Fig. 2f) show the untreated bacteria, which were intact rod-shaped, and the cell wall was not damaged. 4%rGO-(Bi2O3–TiO2) was combined with Pst.DC3000 in a small amount under no light radiation, and the bacteria still remained rod-shaped. However, under visible light, a large amount of 4%rGO-(Bi2O3–TiO2) was clearly observed to adhere to the bacteria, the cell shape was severely deformed, the surface was rough, and the cell wall was ruptured. A study pointed out that TiO2 NPs had high surface-to-volume ratios and different particle shapes compared with organic NPs, and our modified 4%rGO-(Bi2O3–TiO2) also increased its lattice morphology irregularity.66 This allowed the nanomaterials to have more active sites to bind to the bacteria; compared with typical organic molecular antibacterial agents, they could minimize the risk of bacterial resistance.19 At the same time, reports pointed out that under the action of rGO and Bi2O3 NPs, the cell wall of the bacteria would shrink and degrade, and small-sized NPs could directly pass through the cell membrane, causing extravasation of the cell components.29,30 Therefore, we tried exploring the exact mechanism of 4%rGO-(Bi2O3–TiO2) on Pst.DC3000.
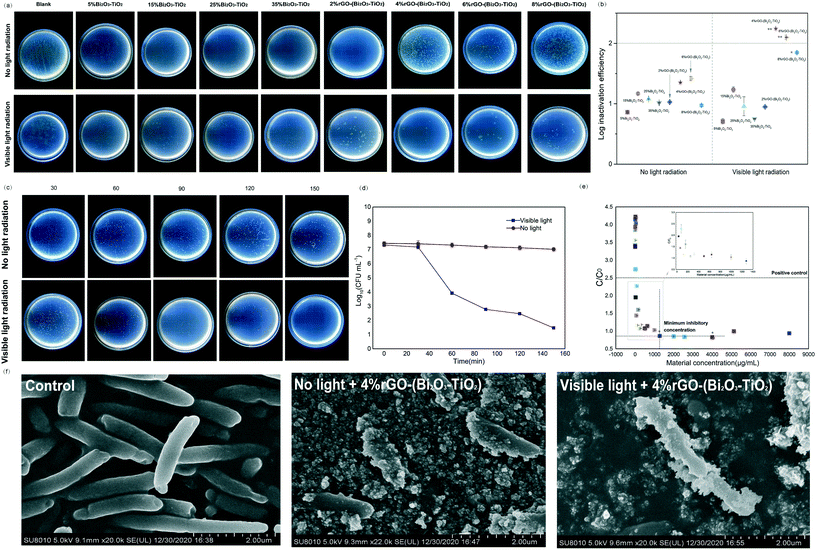 |
| Fig. 2 Antibacterial activity on different nanomaterials (a and b); time dependence of 4%rGO-(Bi2O3–TiO2) antibacterial activity (c and d); MIC for Pst.DC3000 (e); SEM images of Pst.DC3000 under different processes (f). | |
The bacteria-binding ability and cytotoxic effects of nanomaterials
Fig. 3a shows that under visible light, Pst.DC3000 (−5.12 mV) and 4%rGO-(Bi2O3–TiO2) (−12.53 mV) were both negatively charged, and the zeta potential increased to −14.23 mV after mixing. All negative values excluded the role of electrostatic attraction in the binding of nanomaterials to bacteria. Reports indicated that binding might occur through other mechanisms, such as van der Waals forces, hydrophobic interactions, or receptor–ligand interactions.30,31 However, the absolute value increase indicated that their stability was enhanced after mixing, and they were hard to separate afterward.
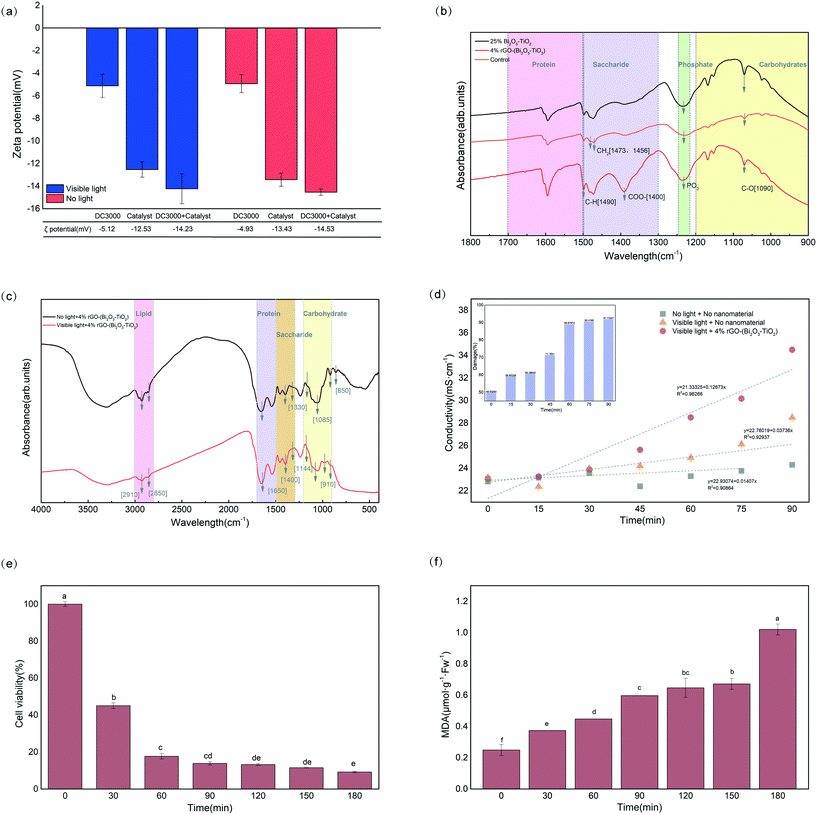 |
| Fig. 3
ζ potential value under different systems (a); FTIR spectrum of LPS exposed to 4%rGO-(Bi2O3–TiO2) (b); FTIR spectrum of Pst.DC3000 exposed to 4%rGO-(Bi2O3–TiO2) (c); the conductivity and damage of Pst.DC3000 (d); cell viability measured by the MTT assay (e); changes in MDA content (f). | |
Fig. 2f shows that the nanomaterials only adhered to the surface of Pst.DC3000 without entering the cells, indicating that 4%rGO-(Bi2O3–TiO2) might interact with individual-specific components on the bacterial surface. Lipopolysaccharide (LPS) with a hydrophobic lipid A region, a hydrophilic O-antigen, and oligosaccharides, usually with phosphate and carboxylic acid groups, was embedded on the outer membrane of Gram-negative bacteria.32 The spectrum in Fig. 3b shows that, compared with the control without NP treatment, the peak value of 4%rGO-(Bi2O3–TiO2) at 1490 cm−1 increased, corresponding to C–O vibration and C–H deformation. The regions 1473 cm−1 and 1456 cm−1 were assigned to the alkyl chain –CH2, and evident vibration occurred. These changes indicated that the outer leaflet of the amide group at the hydrophobic end of the phospholipid was damaged.33 An apparent new peak appeared at 1400 cm−1, relative to the carboxyl group COO−. The peak at 1232 cm−1 corresponding to –PO2– vibrated significantly. The carbohydrate region (C–O) relative to the O-antigen also had obvious deformation (1090 cm−1). It showed that the groups radiating to the outermost layer were easily oxidized.
FTIR was used to identify the changes in biomacromolecules in Pst.DC3000. In particular, the overall change of 4%rGO-(Bi2O3–TiO2) was the most obvious (Fig. S5†). Specifically, the main differences were that the relative strength of the carbohydrate region (850, 910, 1085, 1144 cm−1) and the lipid region (2850, 2910 cm−1) decreased (Fig. 3c). Lipids had characteristic C–H stretching vibrations in the 2800–3000 cm−1 region. The symmetric and asymmetric stretching vibrations of CH2 caused absorption peaks at 2850 and 2910 cm−1, and the peaks were significantly reduced. However, there was no noticeable change in the band caused by the stretching vibration of CH3 at 2880 and 2960 cm−1.34 A study pointed out that the ratio of the methyl band (CH3)/methylene band (CH2) could be used to determine the degree of fatty acid unsaturation.35 The observation results showed that 4%rGO-(Bi2O3–TiO2) increased the bacteria's fatty acid saturation. Due to the disordered structure of amide I, an energy band at 1650 cm−1 was formed.36 The increased peak at 1650 cm−1 was primarily due to the stretching vibration of amino acid residues and the amide's hydrogen bonds due to the C–O stretching vibration.37 The energy band of amide II (1540 cm−1) formed by N–H stretching in-plane bending had no apparent change. In the amide band, the frequency caused by the vibration of amides I and II was positively correlated with the secondary structure of the protein.38 Taking these observations into account, it was found that the ratio of amide II/amide I decreased on 4%rGO-(Bi2O3–TiO2), indicating that the nanomaterials significantly changed the secondary conformation of the protein in the bacteria, leading to inactivation of the protein.
From Fig. 3d, the deterioration of 4%rGO-(Bi2O3–TiO2) to Pst.DC3000 increased with time and reached 91.37% at 90 min. The results showed that the photocatalysis of 4%rGO-(Bi2O3–TiO2) helped increase the permeability of the cell membrane. The extravasation of the contents led to an increase in electrolytes, causing greater damage. Fig. S6† shows that the extracellular protein content of Pst.DC3000 reflected the permeability of the cell membranes and cell walls. The MTT assay was performed to analyze the cytotoxicity. Under the treatment of 4%rGO-(Bi2O3–TiO2), the cell viability at different times was significantly different, and the cell survival rate at 180 min was only 9.8% (Fig. 3e). As shown in (Fig. 3f), as the treatment time increased, the accumulation of malondialdehyde (MDA) increased dramatically to 1.0. The enzyme-like activities pointed out that 4%rGO-(Bi2O3–TiO2) releasing H2O2, hydroxyl and superoxide anions under visible light caused membrane lipid oxidation. The hydroxyl groups produced under photocatalysis could extract hydrogen atoms from the allyl groups of unsaturated fatty acids from the cells.39 The allyl groups and oxygen molecules formed lipid peroxide radicals (LOO˙), finally rearranging to form MDA.40 The MDA precursor had a higher degree of unsaturated fatty acids, which also validated the determination of cell methyl and methylene by FTIR.
The formation of cell aggregates is closely related to the pathogenesis of plant pathogens. Time-dependent changes in the aggregation of Pst.DC3000 were observed using a digital holographic microscope (DHM). Fig. S7(a-l)† shows the changes in various optical characteristics of bacterial aggregation. Bacterial aggregation gradually weakened, the area and volume were dramatically reduced, but the thickness and roughness significantly increased within 150 min of 4%rGO-(Bi2O3–TiO2) treatment compared with untreated cells. Moreover, the irregularity of the cell populations increased significantly in the first 120 min and remained unchanged after 120 min. An increase in thickness usually indicated apoptosis. In general, the morphological and optical changes of bacterial aggregates were closely related to the catalytic activity of 4%rGO-(Bi2O3–TiO2).
Effect of nanomaterials on bacterial speck disease in vivo
The experiment of controlling bacterial speck disease affecting tomato plants in vivo was also further studied. The disease symptoms of untreated inoculated plants first appeared at 5 dpi, black spots were observed, and the leaves faded and withered at 10 dpi (Fig. 4a). In contrast, the tomatoes treated with 4%rGO-(Bi2O3–TiO2) had no obvious black spots and yellow leaves, which greatly delayed the disease symptoms. From Fig. 4b, it was observed that the disease control effect under the treatment of 1280 μg mL−1 4%rGO-(Bi2O3–TiO2) was significantly enhanced to 60.75%. The number of colonies in the control group was 1.72 × 106 CFU mL−1, while the number of colonies under the treatment of 1280 μg mL−1 4%rGO-(Bi2O3–TiO2) decreased by 5
log. rGO had strong functionality against plant pathogens and fungi.20 Our results showed that the method of foliar spraying prevented the proliferation of Pst.DC3000 in the plants. The concentration of nanomaterials was too low to accumulate on the leaves to trigger the antibacterial effect on pathogenic bacteria. Higher concentrations might cause stress to plants, leading to reduced plant autoimmunity and damage to defense mechanisms. Therefore, further discussion was needed to understand the mechanism of 4%rGO-(Bi2O3–TiO2) inducing resistance to Pst.DC3000 in tomatoes. In response to tomato bacterial disease, a study used DNA synthesized AgNPs on the GO surface to control the disease, but there was a high-cost defect.71 After conversion, the amount sprayed in this study is 128 mg kg−1, and the average value of 4%rGO-(Bi2O3–TiO2) is 16 Yuan per kg. There are 1800–2000 tomatoes planted per mu, the leaves of each plant in the later stage of development are about 20 and the material cost per mu is 0.073–0.082 Yuan.
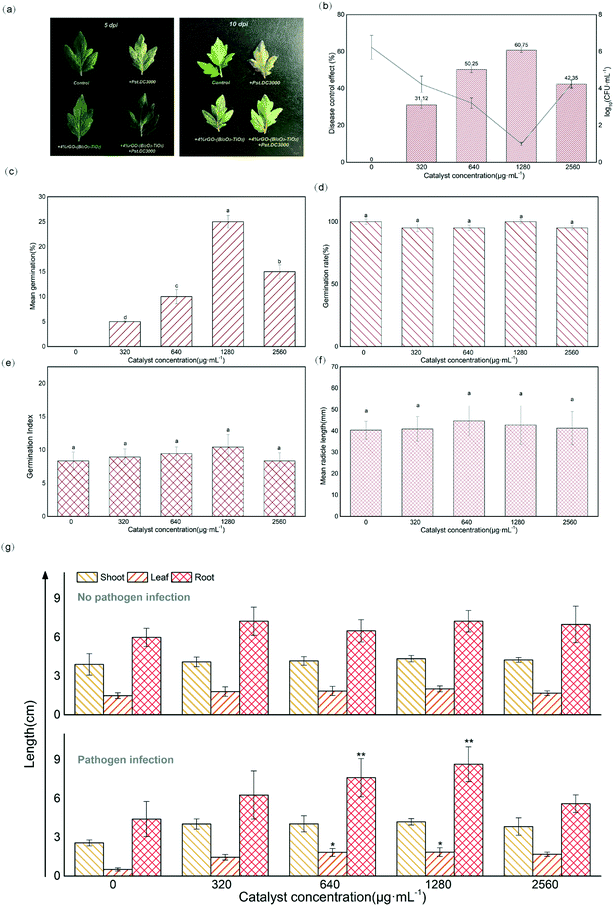 |
| Fig. 4 Images of tomato leaf diseases under different treatments (a); changes of disease control and colony number in the body under different treatments (b); changes of seed germination under different treatments (c–f); changes in growth and development under different treatments (g). | |
Biological effects of 4%rGO-(Bi2O3–TiO2) on tomato
In addition, Fig. 4c–f show that the germination potential of tomato seeds under the treatment of 4%rGO-(Bi2O3–TiO2) increased significantly, while no significant effect was observed on the germination rate, germination index and radicle length (p > 0.05). The germination potential determined the order of germination. The seed germination index was almost the same, indicating that the seed germination had reached the natural threshold,41 and that 4%rGO-(Bi2O3–TiO2) had no adverse effects on tomato seeds. A study showed that carbon nanotubes had a positive effect on the increase in the germination rate of tomato seeds,42 and TiO2 with different particle sizes also had differences in the growth of rice and wheat, indicating that the germination of plants depended on the particle size, dosage and chemical ingredients of NPs.21 No difference was observed in the growth of tomato stems, leaves and roots under 4%rGO-(Bi2O3–TiO2) treatment without inoculation (p > 0.05), while 640 and 1280 μg mL−1 4%rGO-(Bi2O3–TiO2) treatment after inoculation with Pst.DC3000 significantly promoted the growth of plant leaves (p < 0.05) and roots (p < 0.01) (Fig. 4g). Reports pointed out that the titanium base had a positive effect on plant growth. TiO2 increased nitrogen utilization and the photosynthesis rate to improve plant growth.43 The reason might be the higher light absorption capacity of TiO2 and the transmission of light energy or the generation of oxidative stress increased the cell wall pores, thereby increasing the absorption of water and nutrients and promoting root growth.44 The requirements of roots for other elements or changes in surface area increase the need for TiO2 treatment.45 As for the effect of concentration, similar to our previous work, higher concentrations caused the plant biomass to remain unchanged or even decrease because the dose and size of NPs caused them to aggregate into clusters and not act in plants.26
Compared with the nonpathogenic control, the photosynthetic pigment in the leaves after inoculation was significantly reduced, but it increased after 4%rGO-(Bi2O3–TiO2) treatment, which was most significant at 1280 μg mL−1 concentration (p < 0.01). The content of chlorophyll a, chlorophyll b and carotenoids increased by 0.009, 0.008 and 0.004 mg g−1 FW, respectively (Fig. 5a). At the same time, the photosynthetic indices of tomatoes from 11 to 13 time points were measured. The results showed that the Pn, Tr, Gs and WUE of tomato seedlings all decreased under pathogenic bacteria. After 4%rGO-(Bi2O3–TiO2) treatment, each index improved to varying degrees. In particular at a dose of 1280 μg mL−1, the Tr and Gs we messured at 12 o'clock were significantly increased to 0.5 mmol H2O m−2 s−1 and 11.05 mol m−2 s−1 (p < 0.01), respectively (Fig. 5c and d). The photosynthetic rate and WUE we messured at 13 o'clock in leaves increased significantly (Fig. 5b–e). The content of photosynthetic pigment is closely related to plant growth and positively related to the photosynthetic rate,46 which was consistent with the results of this study. According to a report, TiO2 increased the content and absorption of the light-supplementing complex (LHCII) in plants, enhancing the rates of photosynthesis and the oxygen release rate in photosystem II (PSII).26 Studies showed that the enhancement of photosynthesis stems from the antioxidant enzyme-like activity of NPs. A study proved that in photosynthesis, the oxidation of water and the reduction of plastoquinone led to the accumulation of ROS in PSII, and nanomaterials enhanced the photosynthesis of plants by removing excess ROS in chloroplasts.67 This was also consistent with our previous enzyme-like results. Ti, as a beneficial element in crop production, has a positive impact on plant growth and crop quality.74 For example, 4000 mg L−1 and 2000 mg L−1 TiO2 NPs have a positive effect on the root length of cucumber and seed germination of canola.75,76
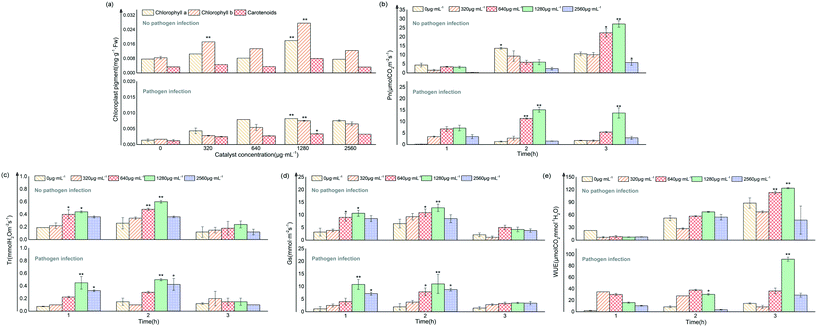 |
| Fig. 5 Changes of photosynthetic pigments under different treatments (a); changes in photosynthetic parameters (Pn, Tr, Gs and WUE) under different treatments (b–e). | |
Effect of nanomaterials on plant resistance and disease resistance
After infection by the pathogen Pst.DC3000, the MDA content in the leaves increased significantly (p < 0.05), and the combined application of 4%rGO-(Bi2O3–TiO2) reduced the MDA content (Fig. 6a). In contrast, the thiol content significantly decreased in the leaves after inoculation. After 4%rGO-(Bi2O3–TiO2) treatment, the thiol content significantly increased, with a significant concentration dependence (Fig. 6b). However, in tomato leaves without 4%rGO-(Bi2O3–TiO2) pretreatment, the electrolyte leakage (EL) (%) after inoculation was significantly higher than that of the control, while the EL (%) after pretreatment decreased from 28.06% to 15.32% (Fig. 6c). The MDA content was used to evaluate the degree of lipid peroxidation (LP). The quantification of thiols and LP was used as a promising biomarker of oxidative stress to assess the oxidation state of cells, including proteins and nonprotein molecules.47 The MDA results showed that 4%rGO-(Bi2O3–TiO2) slowed down the oxidative damage to leaf cells under Pst.DC3000 infection. In antioxidant molecules, thiol compounds also occupied a dominant position, usually including glutathione, cysteine, methionine and plant chelator.48 A study showed that Zn Nps protected thiol oxidation by protecting phospholipids and generating –SH groups with membrane proteins, thereby reducing heavy metal stress.49 Another study also pointed out that plants under oxidative stress could isolate heavy metals in vacuoles through ionized chelation of glutathione and metal-bound thiol metabolism, thereby slowing down heavy metal absorption.50 At the same time, thiols could promote the activity of plant antioxidants and produce cysteine during thiol metabolism, thereby promoting the synthesis of phytochelatin and glutathione.51 The increase of thiol content in this study indicated that the induction of 4%rGO-(Bi2O3–TiO2) enhanced the plant's oxidative stress ability. Melatonin could play a vital role in reducing EL (%) by increasing the proton pump activity of the plant, thereby reducing H2O2 production to improve the integrity of the biofilm of the damaged plant effectively.68 This coincided with our study, indicating that 4%rGO-(Bi2O3–TiO2) induced tolerance to pathogenic bacteria by enhancing the ability of antioxidants. Therefore, the effect of 4%rGO-(Bi2O3–TiO2) on the pathogen-related activity of defense enzymes was further determined.
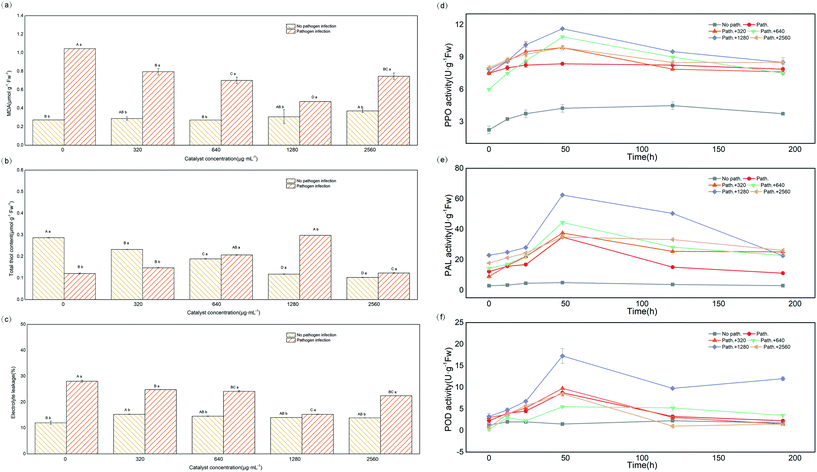 |
| Fig. 6 Changes of oxidative stress indicators (MDA, thiol content and electrolyte leakage) under different treatments (a–c); changes in defense enzyme activity (PPO, PAL and POD) under different treatments (d–f). | |
Fig. 6d–f show that before infection, the activities of PPO, PAL and POD were low. In contrast, after inoculation with Pst.DC3000, the three enzymes' activities were all significantly enhanced, with the largest increased values of 4.5, 30 and 10 U g−1 FW, respectively. The pretreatment of 4%rGO-(Bi2O3–TiO2) further increased the PPO, PAL and POD activities to 8, 60 and 15 U g−1 FW, respectively, compared with the control. PPO enzymes are ubiquitous in higher plants and could catalyze various reactions, such as catalyzing the conversion of oxygen-dependent phenols to quinones, leading to the formation of lignin, quinones and other phenols.36 Quinine substances are aggressive to Monilinia laxa, while quinones might have direct antibiotic and cytotoxic activity against pathogens.52 PAL directly participated in salicylic acid-mediated pathogen defense as a critical enzyme of plant propane metabolism.53 The study reported that the enhancement of PAL activity indicated turmeric extract triggered the plant's immune function against F. solani.69 A previous study also reported that Zn enhanced the PAL activity in the seedlings of clustered beans infected with Rhizoctonia.54 POD was used to remove excess ROS as an endogenous ROS scavenger to maintain plant metabolic balance, and at the same time, like PPO, could participate in the oxidation of plant phenols.55 A study showed that POD played a positive role in the tomato defense system.3 POD was an essential antioxidant enzyme downstream of H2O2 signal transduction. H2O2 acted as an activator of the MAPK cascade. The activation of mitogen-activated protein kinase (MAPK) increased H2O2, which ultimately enhanced the effect of tomato on B. cinerea invasion. The results showed that the enhanced pathogen resistance induced by 4%rGO-(Bi2O3–TiO2) originated from the up-regulation of the defense enzyme activity.
Gene expression and description of antibacterial and disease resistance mechanisms in vivo and in vitro
Fig. 7a shows that the relative expression levels of virulence genes (hrpS, corS, iaaL and flgG) in bacteria were down-regulated, mostly corS was significantly down-regulated by a 10.28-fold change. In contrast, the expression levels of genes related to oxidative stress were all up-regulated, and the expression of sodC, katB and gshB increased to a 1.75-, 2.04- and 1.98-fold change, respectively. The expression levels of genes related to cell damage (recO and omlA) were also down-regulated, representing a 2.78- and 1.80-fold change, respectively. The key virulence determinant of the plant pathogen P. syringae was the type III secretion system (T3SS), which delivered effector proteins to host cells to inhibit the defense signal and other defense responses of PAMP to infect plants.56 T3SS was mainly encoded by hypersensitivity and pathogenic hrp genes, among which hrpS could activate the expression of hrpL and directly affect pathogenicity.57 The plant toxins, plant hormones, and adhesin produced by P. syringae could also affect the virulence effect of the bacteria. For example, coronatine (COR) was mainly responsible for the chlorosis caused by Pst.DC3000, and corS was essential in the synthesis of COR.58iaaL metabolized indole-3-acetic acid (IAA) into the less active 3-indole-acetyl-ε-L-lysine, which reduced Pst.DC3000 toxicity.59 Type IV fimbriae represent one of the main adhesins, and the role of the related gene flgG during bacterial colonization cannot be ignored.60 The results showed that the concentration of 4%rGO-(Bi2O3–TiO2) in plants preferentially inhibited the expression of Pst.DC3000 virulence-related genes and reduced cytotoxicity. The results of MDA and other results showed that the up-regulation of bacterial oxidation defense gene expression resulted from 4%rGO-(Bi2O3–TiO2) induction. Many studies have demonstrated that the production of large amounts of ROS could cause changes in intracellular macromolecules. For example, the unsaturated fatty acids in membrane lipids caused membrane damage, protein denaturation, and cell death.61 Interestingly, according to some studies, the expression of the T3SS gene of Pseudomonas alumina with gshB deletion decreased.62 Also, omlA was usually a regulatory factor that maintained the integrity of the outer cell membrane,63 and its down-regulation was also in line with our results. Fig. 7b clearly shows PR-2, PR-10, PR-13, GSTZ1 and SOD genes in tomato resistance to Pst.DC3000, which were significantly up-regulated by 2.39-, 2.31-, 3.35-, 1.96- and 1.66-fold. According to previous reports, the lack of PR-2 (β-1,3-glucanase) reduced the virus susceptibility, and overexpression speeded up the spread of the virus. PR-2 was reflected in A. thaliana, tobacco and tomato infection processes, improving plant resistance.64 The main feature of PR-13 (thionins) was extensive in vitro antibacterial activity, like plant defensins, leading to cell membrane permeability. Many studies found that transgenic plants expressing thionin genes were more resistant to fungal or bacterial pathogens, such as tomatoes, tobacco and rice.65
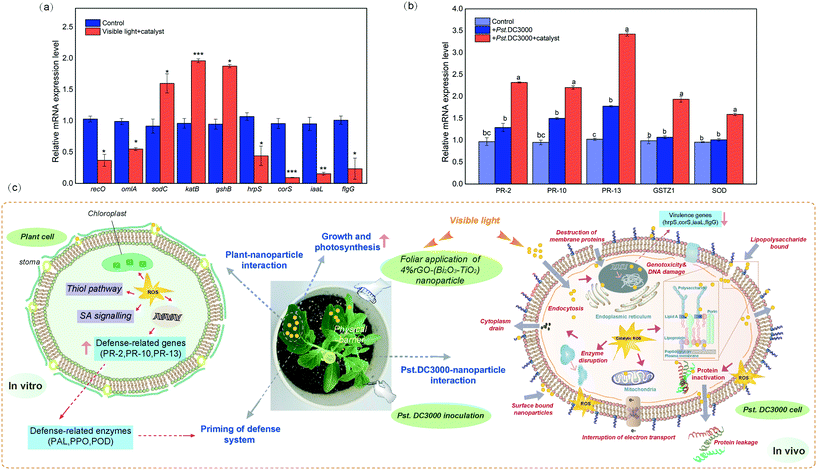 |
| Fig. 7 Relative gene expression levels in treated and untreated Pst.DC3000 (a) and inoculated tomatoes (b); schematic diagram of the antibacterial mechanism of 4%rGO-(Bi2O3–TiO2) on Pst.DC3000 and tomato resistance (c). | |
Fig. 7c summarizes the mechanism of 4%rGO-(Bi2O3–TiO2) against pathogenic bacteria Pst.DC3000 in vitro to enhance the disease resistance of tomatoes. The antibacterial mechanism of Pst.DC3000 was mainly ROS-induced oxidative stress. ROS mainly included 4%rGO-(Bi2O3–TiO2) generated ˙O2− and ˙OH radicals under light radiation and H2O2 generated by the enzyme-like activity. The feature of tight binding to the outer membrane of the cell wall reduced the ROS released by the diffusion distance and also reduced their sterilization limitations. Excessive ROS led to the oxidation of the lipid membrane in the cell wall, increased the permeability of the cell membrane, made the cell membrane become porous, and promoted the internalization of NPs. Afterwards, the cytoplasm and protein in the cell were damaged under ROS, and they were lost through the broken cell membrane, resulting in a decrease in cell viability and eventually death. 4%rGO-(Bi2O3–TiO2) applied on tomato leaves might have accumulated at the penetration site of the pathogenic bacteria to form a physical barrier to avoid the Pst.DC3000 invasion and colonization. Due to the mechanical strength of plant cell walls, plant cells are protected from ROS. At the same time, it enhanced plant growth and primary metabolism (photosynthesis). The enzyme-like properties enhanced the plant's thiol metabolism pathway and promoted its antioxidant function. Besides, phenol metabolism was also enhanced, leading to a significant increase in defense functions (PAL and PPO).
Conclusions
In short, 4%rGO-(Bi2O3–TiO2) in this study effectively adhered to the wall of bacterial cells for in situ sterilization. By changing the cell membrane permeability and protein activity in the cell, the bacteria eventually were inactivated. Importantly, spraying on tomato leaves also significantly inhibited the occurrence of bacterial spot disease and enhanced the critical role of tomato resistance to pathogens. 4%rGO-(Bi2O3–TiO2) stood out in terms of plant resistance and disease resistance. It has potential application value in agricultural nanotechnology, and this is a new method of crop protection worth considering.
Author contributions
Hui Yu: conceptualization, methodology, investigation, writing – original draft, writing – review & editing, project administration. Lei Wang: data curation, writing – review & editing. Jianhua Qu: formal analysis. Xu Wang: resources, investigation. Fuxin Huang: software. Yaqi Jiao: data curation. Ying Zhang: supervision, funding acquisition.
Conflicts of interest
All authors declare no competing interests.
Acknowledgements
This work was supported by the Application Technology Research and Development Projects of Heilongjiang Province (GA19B105), the National Natural Science Fund for Distinguished Young Scholars (41625002), the Natural Science Foundation of Heilongjiang Province of China (ZD2020C001), the National Natural Science Foundation of China (41961144030) and the Heilongjiang Provincial Key Laboratory of Soil Protection.
Notes and references
- Y. F. Neves, A. C. L. Eloi, H. S. Schrekker and J. L. Badel, Imidazolium salts as alternative compounds to control diseases caused by plant pathogenic bacteria, J. Appl. Microbiol., 2020,(128), 1236–1247 CrossRef CAS.
- F. Betoudji, T. Abd El Rahman, M. J. Miller, M. Ghosh, M. Jacques and K. Bouarab,
et al., A Siderophore Analog of Fimsbactin from Acinetobacter Hinders Growth of the Phytopathogen Pseudomonas syringae and Induces Systemic Priming of Immunity in Arabidopsis thaliana, Pathogens, 2020, 9(10), 806 CrossRef CAS PubMed.
- S. C. Chun and M. Chandrasekaran, Chitosan and chitosan nanoparticles induced expression of pathogenesis-related proteins genes enhances biotic stress tolerance in tomato, Int. J. Biol. Macromol., 2019,(125), 948–954 CrossRef CAS.
- R. Roberts, S. Mainiero, A. F. Powell, A. E. Liu, K. Shi and S. R. Hind,
et al., Natural variation for unusual host responses and flagellinmediated immunity against Pseudomonas syringae in genetically diverse tomato accessions, New Phytol., 2019,(223), 447–461 CrossRef CAS.
- K. Griffin, C. Gambley, P. Brown and Y. Li, Copper-tolerance in Pseudomonas syringae pv. tomato and Xanthomonas spp. and the control of diseases associated with these pathogens in tomato and pepper. A systematic literature review, Crop Prot., 2017,(96), 144–150 CrossRef CAS.
- L. Trdá, M. Janda, D. Macková, R. Pospíchalová, P. I. Dobrev and L. Burketová,
et al. Dual Mode of the Saponin Aescin in Plant Protection: Antifungal Agent and Plant Defense Elicitor. Frontiers in, Plant Sci., 2019,(10), 1448 Search PubMed.
- R. Chakraborty, S. Uddin, D. M. Macoy, S. O. Park, D. T. Van Anh and G. R. Ryu,
et al., Inositol-requiring enzyme 1 (IRE1) plays for AvrRpt2-triggered immunity and RIN4 cleavage in Arabidopsis under endoplasmic reticulum (ER) stress, Plant Physiol. Biochem., 2020, 156, 105–114 CrossRef CAS PubMed.
- R. Lanna-Filho, R. M. Souza and E. Alves, Induced resistance in tomato plants promoted by two endophytic bacilli against bacterial speck, Trop. Plant Pathol., 2017, 42(2), 96–108 CrossRef.
- Y. Wang, C. Chen, D. Zhang and J. Wang, Bifunctionalized novel Co-V MMO nanowires: Intrinsic oxidase and peroxidase like catalytic activities for antibacterial application, Appl. Catal., B, 2020, 261, 118256 CrossRef CAS.
- S. Park, K. S. Choi, S. Kim, Y. Gwon and J. Kim, Graphene Oxide-Assisted Promotion of Plant Growth and Stability, Nanomaterials, 2020, 10(4), 758 CrossRef CAS.
- A. Ambrico, M. Trupo, R. Magarelli, R. Balducchi, A. Ferraro and E. Hristoforou,
et al., Effectiveness of Dunaliella salina Extracts against Bacillus subtilis and Bacterial Plant Pathogens, Pathogens, 2020, 9(8), 613 CrossRef CAS.
- S. E. El-Abeid, Y. Ahmed, J.-A. Daròs and M. A. Mohamed, Reduced Graphene Oxide Nanosheet-Decorated Copper Oxide Nanoparticles: A Potent Antifungal Nanocomposite against Fusarium Root Rot and Wilt Diseases of Tomato and Pepper Plants, Nanomaterials, 2020, 10(5), 1001 CrossRef CAS.
- S. T. Khan, J. Ahmad, M. Ahamed and A. Jousset, Sub-lethal doses of widespread nanoparticles promote antifungal activity in Pseudomonas protegens CHA0, Sci. Total Environ., 2018,(627), 658–662 CrossRef CAS.
- H. Tombuloglu, Y. Slimani, T. M. AlShammari, M. Bargouti, M. Ozdemir and G. Tombuloglu,
et al., Uptake, translocation, and physiological effects of hematite (α-Fe2O3) nanoparticles in barley (Hordeum vulgare L.), Environ. Pollut., 2020,(266), 115391 CrossRef CAS.
- L. Lu, M. Huang, Y. Huang, P. F.-X. Corvini, R. Ji and L. Zhao, Mn3O4 nanozymes boost endogenous antioxidant metabolites in cucumber (Cucumis sativus) plant and enhance resistance to salinity stress, Environ. Sci.: Nano, 2020, 7(6), 1692–1703 RSC.
- X. Wang, L. Fan, L. Cheng, Y. Sun, X. Wang and X. Zhong,
et al., Biodegradable Nickel Disulfide Nanozymes with GSH-Depleting Function for High-Efficiency Photothermal-Catalytic Antibacterial Therapy, iScience, 2020, 23(7), 101281 CrossRef CAS PubMed.
- I. Muzzalupo, G. Badolati, A. Chiappetta, N. Picci and R. Muzzalupo, In vitro Antifungal Activity of Olive (Olea europaea) Leaf Extracts Loaded in Chitosan Nanoparticles, Front. Bioeng. Biotechnol., 2020,(8), 151 CrossRef PubMed.
- L. Kőrösi, B. Pertics, G. Schneider, B. Bognár, J. Kovács and V. Meynen,
et al., Photocatalytic Inactivation of Plant Pathogenic Bacteria Using TiO2 Nanoparticles Prepared Hydrothermally, Nanomaterials, 2020, 10(9), 1730 CrossRef PubMed.
- R. Hernandez-Delgadillo, D. Velasco-Arias, J. J. Martinez-Sanmiguel, D. Diaz, I. Zumeta-Dube and K. Arevalo-Niño,
et al., Bismuth oxide aqueous colloidal nanoparticles inhibit Candida albicans growth and biofilm formation, Int. J. Nanomed., 2013,(8), 1645–1652 Search PubMed.
- F. A. Bezza, S. M. Tichapondwa and E. M. N. Chirwa, Fabrication of monodispersed copper oxide nanoparticles with potential application as antimicrobial agents, Sci. Rep., 2020, 10(1), 16680 CrossRef CAS PubMed.
- Z. A. Siddiqui, A. Parveen, L. Ahmad and A. Hashem, Effects of graphene oxide and zinc oxide nanoparticles on growth, chlorophyll, carotenoids, proline contents and diseases of carrot, Sci. Hortic., 2019, 249, 374–382 CrossRef CAS.
- Y. Zhang, H. Yu, S. Li, L. Wang, F. Huang and R. Guan,
et al., Rapidly degradation of di-(2-ethylhexyl) phthalate by Z-scheme Bi2O3/TiO2@reduced graphene oxide driven by simulated solar radiation, Chemosphere, 2021, 272, 129631 CrossRef CAS.
- C. Zhang, P. Howlader, T. Liu, X. Sun, X. Jia and X. Zhao,
et al., Alginate Oligosaccharide (AOS) induced resistance to Pst DC3000 via salicylic acid-mediated signaling pathway in Arabidopsis thaliana, Carbohydr. Polym., 2019, 225, 115221 CrossRef CAS.
- J. Wu, X. Wang, Q. Wang, Z. Lou, S. Li and Y. Zhu,
et al., Nanomaterials with enzyme-like characteristics (nanozymes): next-generation artificial enzymes (II), Chem. Soc. Rev., 2019, 48(1004), 74 Search PubMed.
- M. S. Kim, S. Cho, S. H. Joo, J. Lee, S. K. Kwak and M. I. Kim,
et al., N- and B-Codoped Graphene: A Strong Candidate to Replace Natural Peroxidase in Sensitive and Selective Bioassays, ACS Nano, 2019, 13(4), 4312–4321 CrossRef CAS.
- J. Hu, X. Wu, F. Wu, W. Chen, J. C. White and Y. Yang,
et al., Potential application of titanium dioxide nanoparticles to improve the nutritional quality of coriander (Coriandrum sativum L.), J. Hazard. Mater., 2020,(389), 121837 CrossRef CAS.
- W. Wang, J. Yang, J. Zhang, Y.-X. Liu, C. Tian and B. Qu,
et al., An Arabidopsis Secondary Metabolite Directly Targets Expression of the Bacterial Type III Secretion System to Inhibit Bacterial Virulence, Cell Host Microbe, 2020, 27(4), 601–613. e7 CrossRef CAS PubMed.
- Á. Martínez-Camarena, J. M. Llinares, A. Domenech-Carbó, J. Alarcón and E. García-España, A step forward in the development of superoxide dismutase mimetic nanozymes: the effect of the charge of the surface on antioxidant activity, RSC Adv., 2019, 9(71), 41549–41560 RSC.
- T. Alam, R. A. A. Khan, A. Ali, H. Sher, Z. Ullah and M. Ali, Biogenic synthesis of iron oxide nanoparticles via Skimmia laureola and their antibacterial efficacy against bacterial wilt pathogen Ralstonia solanacearum, Mater. Sci., 2019,(98), 101–108 CAS.
- P. Eramabadi, M. Masoudi, A. Makhdoumi and M. Mashreghi, Microbial cell lysate supernatant (CLS) alteration impact on platinum nanoparticles fabrication, characterization, antioxidant and antibacterial activity, Mater. Sci. Eng., C, 2020,(117), 111292 CrossRef CAS.
- Y. H. Leung, X. Xu, A. P. Y. Ma, F. Liu, A. M. C. Ng and Z. Shen,
et al., Toxicity of ZnO and TiO2 to Escherichia coli cells, Sci. Rep., 2016, 6(1), 35243 CrossRef CAS PubMed.
- M. A. Ansari, H. M. Khan, A. A. Khan, S. S. Cameotra, Q. Saquib and J. Musarrat, Interaction of Al2O3 nanoparticles with Escherichia coli and their cell envelope biomolecules, J. Appl. Microbiol., 2014, 116(4), 772–783 CrossRef CAS PubMed.
- Z. Qu, P. Liu, X. Yang, F. Wang, W. Zhang and C. Fei, Microstructure and Characteristic of BiVO4 Prepared under Different pH Values: Photocatalytic Efficiency and Antibacterial Activity, Materials, 2016, 9(3), 129 CrossRef PubMed.
- R. Lahlali, Y. Jiang, S. Kumar, C. Karunakaran, X. Liu and F. Borondics,
et al., ATR–FTIR spectroscopy reveals involvement of lipids and proteins of intact pea pollen grains to heat stress tolerance, Front. Plant Sci., 2014, 22, 5 Search PubMed.
- S. Sharma and K. N. Uttam, Investigation of the manganese stress on wheat plant by attenuated total reflectance Fourier transform infrared spectroscopy, Spectrosc. Lett., 2016, 49(8), 520–528 CrossRef CAS.
- C. Guo, X. Guo, W. Chu, N. Jiang and H. Li, FTIR-ATR study for adsorption of trypsin in aqueous environment on bare and TiO2 coated ZnSe surfaces, Chin. Chem. Lett., 2020, 31(1), 150–154 CrossRef CAS.
- P. Ju, Y. Zhang, Y. Zheng, F. Gao, F. Jiang and J. Li,
et al., Probing the toxic interactions between polyvinyl chloride microplastics and Human Serum Albumin by multispectroscopic techniques, Sci. Total Environ., 2020,(734), 139219 CrossRef CAS.
- S. Suresh, S. Karthikeyan and K. Jayamoorthy, Effect of bulk and nano-Fe2O3 particles on peanut plant leaves studied by Fourier transform infrared spectral studies, J. Adv. Res., 2016, 7(5), 739–747 CrossRef CAS.
- F. Zhao, S. Wang, Z. Zhu, S. Wang, F. Liu and G. Liu, Effects of oxidation degree on photo-transformation and the resulting toxicity of graphene oxide in aqueous environment, Environ. Pollut., 2019,(249), 1106–1114 CrossRef CAS PubMed.
- R. K. Dutta, B. P. Nenavathu, M. K. Gangishetty and A. V. R. Reddy, Studies on antibacterial activity of ZnO nanoparticles by ROS induced lipid peroxidation, Colloids Surf., B, 2012,(94), 143–150 CrossRef CAS.
- E. S. Melby, A. C. Mensch, S. E. Lohse, D. Hu, G. Orr and C. J. Murphy,
et al., Formation of supported lipid bilayers containing phase-segregated domains and their interaction with gold nanoparticles, Environ. Sci.: Nano, 2016, 3(1), 45–55 RSC.
- M. V. Khodakovskaya, B.-S. Kim, J. N. Kim, M. Alimohammadi, E. Dervishi and T. Mustafa,
et al., Carbon Nanotubes as Plant Growth Regulators: Effects on Tomato Growth, Reproductive System, and Soil Microbial Community, Small, 2013, 9(1), 115–123 CrossRef CAS PubMed.
- S. Lyu, X. Wei, J. Chen, C. Wang, X. Wang and D. Pan, Titanium as a Beneficial Element for Crop Production, Front. Plant Sci., 2017,(8), 597 CrossRef PubMed.
- S. Ullah, M. Adeel, M. Zain, M. Rizwan, M. K. Irshad and G. Jilani,
et al., Physiological and biochemical response of wheat (Triticum aestivum) to TiO2 nanoparticles in phosphorous amended soil: A full life cycle study, J. Environ. Manage., 2020,(263), 110365 CrossRef CAS PubMed.
- R. Szymańska, K. Kołodziej, I. Ślesak, P. Zimak-Piekarczyk, A. Orzechowska and M. Gabruk,
et al., Titanium dioxide nanoparticles (100–1000 mg/l) can affect vitamin E response in Arabidopsis thaliana, Environ. Pollut., 2016, 213, 957–965 CrossRef.
- A. Movafeghi, A. Khataee, M. Abedi, R. Tarrahi, M. Dadpour and F. Vafaei, Effects of TiO2 nanoparticles on
the aquatic plant Spirodela polyrrhiza: Evaluation of growth parameters, pigment contents and antioxidant enzyme activities, J. Environ. Sci., 2018,(64), 130–138 CrossRef CAS PubMed.
- S. Spormann, C. Soares and F. Fidalgo, Salicylic acid alleviates glyphosate-induced oxidative stress in Hordeum vulgare L, J. Environ. Manage., 2019, 241, 226–234 CrossRef CAS.
- N. Shabnam and H. Kim, Non-toxicity of nano alumina: A case on mung bean seedlings, Ecotoxicol. Environ. Saf., 2018,(165), 423–433 CrossRef CAS PubMed.
- A. A. Shah, S. Aslam, M. Akbar, A. Ahmad, W. U. Khan and N. A. Yasin,
et al., Combined effect of Bacillus fortis IAGS 223 and zinc oxide nanoparticles to alleviate cadmium phytotoxicity in Cucumis melo, Plant Physiol. Biochem., 2021, 158, 1–12 CrossRef CAS.
- S. Awasthi, R. Chauhan, S. Dwivedi, S. Srivastava, S. Srivastava and R. D. Tripathi, A consortium of alga (Chlorella vulgaris) and bacterium (Pseudomonas putida) for amelioration of arsenic toxicity in rice: A promising and feasible approach, Environ. Exp. Bot., 2018,(150), 115–126 CrossRef CAS.
- S. Awasthi, R. Chauhan, S. Srivastava and R. D. Tripathi, The Journey of Arsenic from Soil to Grain in Rice, Front. Plant Sci., 2017,(8), 1007 CrossRef.
- Y. Zheng, Y. Yang, C. Liu, L. Chen, J. Sheng and L. Shen, Inhibition of SlMPK 1, SlMPK 2, and SlMPK 3 Disrupts Defense Signaling Pathways and Enhances Tomato Fruit Susceptibility to Botrytis cinerea, J. Agric. Food Chem., 2015, 63(22), 5509–5517 CrossRef CAS PubMed.
- B. Liu, X. Han and J. Liu, Iron oxide nanozyme catalyzed synthesis of fluorescent polydopamine for light-up Zn2+ detection, Nanoscale, 2016, 8(28), 13620–13626 RSC.
- N. Wadhwa, U. N. Joshi and N. Mehta, Zinc Induced Enzymatic Defense Mechanisms in Rhizoctonia Root Rot Infected Clusterbean Seedlings, J. Bot., 2014,(2014), 1–7 Search PubMed.
- M. Mohammadi and H. Kazemi, Changes in peroxidase and polyphenol oxidase activities in susceptible and resistant wheat heads inoculated with Fusarium graminearum and induced resistance, Plant Sci., 2002, 162(4), 491–498 CrossRef CAS.
- Q. Yan, C. J. Rogan, Y.-Y. Pang, E. W. Davis and J. C. Anderson, Ancient co-option of an amino acid ABC transporter locus in Pseudomonas syringae for host signal-dependent virulence gene regulation. Mackey D, editor, PLoS Pathog., 2020, 16(7), e1008680 CrossRef CAS.
- Y. Wang, G. Feng, Z. Zhang, Y. Liu, Y. Ma and Y. Wang,
et al., Overexpression of Pti4, Pti5, and Pti6 in tomato promote plant defense and fruit ripening, Plant Sci., 2020, 302(6), 110702 Search PubMed.
- A. V. Smirnova, Y. Braun and M. S. Ullrich, Site-directed mutagenesis of the temperature-sensing histidine protein kinase CorS from Pseudomonas syringae: Site-directed mutagenesis of CorS, FEMS Microbiol. Lett., 2008, 283(2), 231–238 CrossRef CAS.
- M. G. Castillo-Lizardo, I. M. Aragón, V. Carvajal, I. M. Matas, M. L. Pérez-Bueno and M.-T. Gallegos,
et al., Contribution of the non-effector members of the HrpL regulon, iaaL and matE, to the virulence of Pseudomonas syringae pv. tomato DC3000 in tomato plants, BMC Microbiol., 2015, 15(1), 165 CrossRef.
- J. Chen, S. Li, J. Luo, Y. Zhang and W. Ding, Graphene Oxide Induces Toxicity and Alters Energy Metabolism and Gene Expression in Ralstonia solanacearum, J. Nanosci. Nanotechnol., 2017, 17(1), 186–195 CrossRef CAS PubMed.
- Y. Xie, Y. He, P. L. Irwin, T. Jin and X. Shi, Antibacterial Activity and Mechanism of Action of Zinc Oxide Nanoparticles against Campylobacter jejuni, Appl. Environ. Microbiol., 2011, 77(7), 2325–2331 CrossRef CAS.
- Y. Zhang, C. Zhang, X. Du, Y. Zhou, W. Kong and G. W. Lau,
et al., Glutathione Activates Type III Secretion System Through Vfr in Pseudomonas aeruginosa, Front. Cell. Infect. Microbiol., 2019,(9), 164 CrossRef PubMed.
- M. Fuangthong, R. Sallabhan, S. Atichartpongkul, N. Rangkadilok, R. Sriprang and J. Satayavivad,
et al., The omlA gene is involved in multidrug resistance and its expression is inhibited by coumarins in Xanthomonas campestris pv. phaseoli, Arch. Microbiol., 2008, 189(3), 211–218 CrossRef CAS PubMed.
- G. A. Abo-Zaid, S. M. Matar and A. Abdelkhalek, Induction of Plant Resistance against Tobacco Mosaic Virus Using the Biocontrol Agent Streptomyces cellulosae Isolate Actino 48, Agronomy, 2020, 10(11), 1620 CrossRef CAS.
- J. Sels, J. Mathys, B. M. A. De Coninck, B. P. A. Cammue and M. F. C. De Bolle, Plant pathogenesis-related (PR) proteins: A focus on PR peptides, Plant Physiol. Biochem., 2008, 46(11), 941–950 CrossRef CAS.
- K. Rambabu, G. Bharath, F. Banat and P. L. Show, Green synthesis of zinc oxide nanoparticles using Phoenix dactylifera waste as bioreductant for effective dye degradation and antibacterial performance in wastewater treatment, J. Hazard. Mater., 2021,(402), 123560 CrossRef CAS PubMed.
- Y. Meng, Q. Yin, Z. Yan, Y. Wang, J. Niu and J. Zhang,
et al., Exogenous Silicon Enhanced Salt Resistance by Maintaining K+/Na+ Homeostasis and Antioxidant Performance in Alfalfa Leaves, Front. Plant Sci., 2020,(11), 1183 CrossRef PubMed.
- S. Tousi, P. Zoufan and A. R. Ghahfarrokhie, Alleviation of cadmium-induced phytotoxicity and growth improvement by exogenous melatonin pretreatment in mallow (Malva parviflora) plants, Ecotoxicol. Environ. Saf., 2020,(206), 111403 CrossRef CAS.
- A. A. Alsahli, I. A. Alaraidh, Y. M. Rashad and E. S. Abdel Razil, Extract from Curcuma longa L. triggers the sunflower immune system and induces defense-related genes against Fusarium root rot, Phytopathol. Mediterr., 2018, 57(1), 26–36 CAS.
- S. Liu, X. Shao, Y. Wei, Y. Li, F. Xu and H. Wang, Solidago canadensis L. Essential Oil Vapor Effectively Inhibits Botrytis cinerea Growth and Preserves Postharvest Quality of Strawberry as a Food Model System, Front. Microbiol., 2016, 07, 1179 Search PubMed.
- I. Ocsoy, M. L. Paret, M. A. Ocsoy, S. Kunwar, T. Chen and M. You,
et al., Nanotechnology in Plant Disease Management: DNA Directed Silver Nanoparticles on Graphene Oxide as an Antibacterial against Xanthomonas Perforans, ACS Nano, 2013, 7(10), 8972–8980 CrossRef CAS PubMed.
- Y. Su, V. Ashworth, C. Kim, A. S. Adeleye, P. Rolshausen and C. Roper,
et al., Delivery, uptake, fate, and transport of engineered nanoparticles in plants: a critical review and data analysis, Environ. Sci.: Nano, 2019, 6(8), 2311–2331 RSC.
- L. M. Gilbertson, L. Pourzahedi, S. Laughton, X. Gao, J. B. Zimmerman and T. L. Theis,
et al., Guiding the design space for nanotechnology to advance sustainable crop production, Nat. Nanotechnol., 2020, 15(9), 801–810 CrossRef CAS.
- C. Larue, J. Laurette, N. Herlin-Boime, H. Khodja, B. Fayard and A.-M. Flank,
et al., Accumulation, translocation and impact of TiO2 nanoparticles in wheat (Triticum aestivum spp.): Influence of diameter and crystal phase, Sci. Total Environ., 2012,(431), 197–208 CrossRef CAS.
- H. Mahmoodzadeh, M. Nabavi and H. Kashefi, Effect of nanoscale titanium dioxide particles on the germination and growth of canola (Brassica napus), J. Ornam. Hortic. Plants, 2013, 3, 25–32 Search PubMed.
- A. D. Servin, H. Castillo-Michel, J. A. Hernandez-Viezcas, B. C. Diaz, J. R. Peralta-Videa and J. L. Gardea-Torresdey, Synchrotron Micro-XRF and Micro-XANES Confirmation of the Uptake and Translocation of TiO 2 Nanoparticles in Cucumber (Cucumis sativus) Plants, Environ. Sci. Technol., 2012, 46(14), 7637–7643 CrossRef CAS.
Footnote |
† Electronic supplementary information (ESI) available: Methods S1–S6, Tables S1 and S2, and Fig. S1–S7. See DOI: 10.1039/d1en00558h |
|
This journal is © The Royal Society of Chemistry 2022 |
Click here to see how this site uses Cookies. View our privacy policy here.