Direct and nitrite-sensitized indirect photolysis of effluent-derived phenolic contaminants under UV254 irradiation†
Received
9th September 2021
, Accepted 2nd December 2021
First published on 4th December 2021
Abstract
UV254 photolysis has increasingly been utilized for disinfection of water-born pathogens in wastewater. During disinfection, wastewater-derived trace organic contaminants, such as pharmaceuticals and personal care products (PPCPs), may be subjected to direct photolysis and indirect photolysis sensitized by wastewater constituents such as nitrite (NO2−). Herein, we reported the direct photolysis and NO2−-sensitized indirect photolysis of four phenolic contaminants commonly observed in wastewaters (i.e., bisphenol A (BPA), acetaminophen (ATP), salbutamol (SAL), and 2,4-dihydroxybenzophenone (BP1)). Spectroscopic characterization and quantum yield measurement were carried out to evaluate the photochemical reactivity of these phenolic compounds. In NO2−-sensitized photolysis, the relative contribution of direct and indirect photolysis was quantified by light screening factor calculation and radical quenching studies. The experimental results highlight the important roles of HO˙ and NO2˙ in the NO2−-sensitized photolysis of phenolic compounds. A series of intermediate products, including hydroxylated, nitrated, nitrosated, dimerized, and alkyl chain cleavage products, were identified by solid phase extraction (SPE) combined with high-resolution mass spectrometry (HRMS) analyses. On the basis of identified products, the underlying mechanisms and transformation pathways for NO2−-sensitized photolysis of these phenolic compounds were elucidated. The second-order rate constants of BPA, SAL, BP1 with NO2˙ were calculated to be 2.25 × 104, 1.35 × 104 and 2.44 × 104 M−1 s−1, respectively, by kinetic modeling. Suwanee River natural organic matter (SRNOM) played complex roles in the direct and NO2−-sensitized photolysis of phenolic compounds by serving as a photosensitizer, light screening and radical quenching agent. Wastewater constituents, such as NO3− and EfOM, could accelerate direct and NO2−-sensitized photolysis of BPA, SAL, and BP1 in the wastewater matrix. Our results suggest that NO2− at the WWTP effluent-relevant level can sensitize the photolysis of effluent-derived phenolic contaminants during the UV254 disinfection process; however, the formation of potentially carcinogenic and mutagenic nitrated/nitrosated derivatives should be scrutinized.
Environmental significance
Phenolic compounds are trace organic contaminants (TrOCs) commonly encountered in wastewater effluents (PPCPs, endocrine disrupting chemicals, synthetic industrial chemicals, etc.). These chemicals may cause endocrinological abnormalities of aquatic species and are known to be neurotoxic and cytotoxic to humans. Abiotic processes such as photolysis (including both direct and indirect photolysis) play an important role in the transformation and fate of TrOCs such as phenolic contaminants. Exposure to UV254 radiation resulted in efficient removal of BPA, ATP, SAL and BP1 in aqueous solution in the absence or presence of NO2−. However, the potential formation of nitrated/nitrosated derivatives should be scrutinized due to their carcinogenicity and mutagenicity.
|
1. Introduction
Pharmaceuticals and personal care products (PPCPs) have become a group of emerging contaminants that attract great attention in recent years due to their widespread occurrence and potential ecotoxicity.1 PPCPs can enter municipal wastewater treatment systems via several routes such as secretion of feces and urine as well as disposal of expired drugs.2 Due to limited removal efficiency of conventional wastewater treatment plants (WWTPs), high concentrations of PPCPs are often detected in WWTP effluents.3 Therefore, WWTPs serve as an important collector of PPCPs as well as a transfer for their occurrence in the environment.4 For example, 2,4-dihydroxybenzophenone (BP1), a sunscreen agent, was present in WWTP effluent at concentrations as high as 155 ng L−1.5 Similarly, the concentrations of the analgesic drug acetaminophen (ATP) and the β2-adrenoceptor agonist salbutamol (SAL) were detected to be 97 and 0.17 ng L−1, respectively, in WWTP effluent.2,6 Once released into the environment, these PPCPs and their metabolites can pose potential risks to aquatic species even at concentrations as low as nanograms per liter.5 For example, yeast two-hybrid assay revealed that BP1 had estrogenic and anti-androgenic effects and thus could cause endocrinological abnormalities in aquatic species.7 Past studies have shown that biological processes alone cannot effectively remove these PPCPs.8 Other abiotic transformation processes, such as photochemical degradation, may also be responsible for their attenuation in natural environments.9–12
UV photolysis is an effective approach widely applied for drinking water purification and wastewater disinfection.13,14 Exposure to UV light can efficiently damage the genetic materials of bacteria and viruses (i.e., DNA and RNA), thus making them inactivated or dead.15 While UV254 water disinfection is usually very fast, sufficient UV fluence has potential to trigger important photochemical reactions.14 For example, during UV disinfection, some PPCPs can undergo direct photolysis through absorbing photons, triggering chemical reactions such as bond cleavage, intramolecular rearrangement, isomerization, and dimerization.16 In addition, some natural photosensitizers in the WWTP effluent, such as nitrate/nitrite (NO3−/NO2−), ferric (Fe3+) species, and effluent-derived organic matter (EfOM), can significantly promote the attenuation of organic contaminants through photochemically produced reactive intermediates (PPRI).17,18 Therefore, both direct and sensitized photolysis may play roles in the transformation and fate of PPCPs in WWTP effluent during UV disinfection.19,20 In particular, when the direct photolysis of PPCPs is weak, indirect photolysis mediated by photosensitizers will become the dominant mechanism accounting for their decay in the effluent.21
| NO2− + hν → NO˙ + O˙− | (R1) |
|  | (R2) |
| NO2− + HO˙·→ NO2˙ + OH−, k0 = 1.0 × 1010 M−1 s−1 | (R3) |
Previous studies have shown that NO3− has potential to sensitize the photolysis of PPCPs in WWTP effluent.22,23 However, the potential role of NO2− in photosensitization of PPCPs in WWTP effluent is largely unknown. In addition, NO2− has a high molar absorption coefficient and quantum yield at wavelength 200–400 nm (Φ254HO˙ = 0.11, and Φ365HO˙ = 0.025 for NO2− photolysis, while Φ254HO˙ = 0.09, and Φ310HO˙ = 0.01 for NO3− photolysis), which makes it a competitive photosensitizer.24–27 It was demonstrated that the photosensitization of NO2− plays an important role in the transformation of contaminants in surface waters, atmospheric waters, and aerosols.9,25 For instance, our recent study showed that trace level NO2− under UVA (365 nm) irradiation significantly sensitized the photolysis of the antibacterial agents chloroxylenol and chlorophene in water.28 As a photosensitizer, NO2− is capable of generating a series of radicals including hydroxyl radicals (HO˙) and reactive nitrogen species (RNS) such as NO2˙ and NO˙ (see ((R1)–(R3)). These reactive radicals are electrophiles that tend to attack electron-rich moieties of organic contaminants (e.g., phenolic and anilino groups), leading to the formation of hydroxylated, nitrated, and nitrosated byproducts.28,29 Since nitrated and nitrosated aromatic derivatives are phytotoxic, mutagenic, and potentially carcinogenic,27 their formation during NO2−-sensitized photolysis of organic contaminants should be carefully evaluated.
Many PPCPs such as ATP, SAL, and BP1 contain phenolic groups. In addition, human metabolism of PPCPs, in particular phase I metabolism, may also result in the formation of phenolic derivatives.30 Previous studies have shown that phenolic compounds are susceptible toward the attack of RNS, generating nitrated and nitrosated byproducts.28,31 The typical concentration of NO2− in conventional wastewater treatment plant effluent has been reported to be ∼2.5 μM;32 however, higher levels are likely encountered in nitrification/denitrification effluent.23 We speculated that NO2− in the wastewater matrix could sensitize the photolysis of some phenolic PPCPs during UV disinfection. However, knowledge on this topic is very scarce, which limits our understanding of the role of NO2− in photo-transformation of PPCPs and formation of toxic byproducts. It is well known that NO2˙ is an important nitrating agent that can cause the nitration of phenolic compounds through two stages.33,34 Firstly, the electrophilic attack of NO2˙ at a phenolic molecule is known to generate a phenoxyl radical through H-abstraction.35 Subsequently, a coupling reaction between the nascent phenoxyl radical and another NO2˙ gives rise to nitrated byproducts.36,37 The bimolecular rate constant between NO2˙ and several phenolic compounds (e.g., phenol and 4-chlorophenol) has been reported to be within 103–104 M−1 s−1;26,35 however, the reactivity of NO2˙ with many other phenolic compounds is unknown. Many phenolic PPCPs are photolabile compounds due to their electron-rich properties.38 Therefore, the presence of NO2− may change the photochemical behavior of PPCPs from direct to indirect photolysis, which will affect the removal efficiency and transformation mechanisms of PPCPs. However, kinetic information associated with the direct and NO2−-mediated indirect photolysis of phenolic PPCPs is still lacking, which warrants further investigations.
In this contribution, three typical phenolic PPCPs, including ATP, SAL, and BP1, were selected as target compounds for investigating their photochemical behavior in the absence (direct photolysis) and presence of NO2− (NO2−-sensitized photolysis). Ultraviolet light of 254 nm (UV254) was used as the irradiation source due to its widespread application for disinfection of pathogens in wastewater.13 ATP and SAL are antipyretic and antiasthmatic pharmaceuticals, respectively, and BP1 is a sunscreen agent. In addition, bisphenol A (BPA), a plasticizer frequently detected in WWTP effluent, was also included as a target compound (see Table S1 of the ESI† for physicochemical properties of the studied phenolic compounds). We attempted to: (i) investigate the photochemical reactivity of the four phenolic compounds and determine their molar absorption coefficients and quantum yields under UV254 irradiation; (ii) study the indirect photolysis of these phenolic compounds driven by NO2− sensitization, and quantify the relative contribution of direct and indirect photolysis; (iii) justify the roles of HO˙ and NO2˙ in the NO2−-sensitized photolysis and determine the second-order rate constants between NO2˙ and the phenolic compounds; (iv) identify the transformation products (TPs) generated during NO2−-sensitized photolysis of target compounds, and elucidate underlying mechanisms; (v) evaluate the effects of natural organic matter (NOM) and the wastewater matrix on the direct and NO2−-sensitized indirect photolysis of BPA, ATP, SAL, and BP1.
2. Materials and methods
2.1. Reagents and materials
Chemicals, suppliers, and purities are listed in Text S1 of the ESI.† Oasis hydrophilic–lipophilic balance (HLB) cartridges (6 cm3/200 mg, WAT106202) were purchased from Waters Corporation (Milford, MA, USA). All the stock solutions were prepared by dissolving reagents in Milli-Q water and used within one week.
2.2. Water sample
The wastewater sample was collected from the secondary sedimentation tank of a local municipal sewage treatment plant (Nanjing, China) and filtered using a 0.22 μm hydrophilic filter membrane (polyether sulfone, PES) prior to experiments. The characteristics of the wastewater, including TOC, Abs254, NO3−, NO2−, pH, and typical inorganic ions are provided in Table S2 of the ESI.†
2.3. Instrumentation
The photolysis experiments were performed in a BL-GHX-V photoreactor (Shanghai Bilon Instrument Co., Ltd, China) equipped with a 15 W low-pressure mercury vapor lamp (LP-Hg) emitting mainly 254 nm ultraviolet light. The irradiance of incident photons was determined to be 8.7 × 10−7 einstein L−1 s−1 using atrazine as an actinometer.14 The LP-Hg lamp was mounted in a quartz cooling jacket connected to a BL-T-1000S circulating cooler to maintain the temperature at 20 ± 1 °C.
The concentrations of phenolic compounds were quantitatively analyzed using a high-performance liquid chromatograph (HPLC) (Shimadzu, LC-16AD) equipped with a photodiode array detector (PAD-M30A) and an Agilent Zorbax Eclipse Plus C18 reverse-phase column (5 μm, 250 mm × 4.6 mm, i.d.). UV-visible (UV-Vis) absorption spectra were recorded using a Varian Cary 50 UV-Vis spectrophotometer (Varian, USA). Fluorescence emission spectra were recorded with an F-4500 fluorescence spectrometer. Product identifications were carried out using a HPLC (Shimadzu, Kyoto, Japan) coupled with a Triple TOF 5600+ mass spectrometer (LC-qTOF-MS/MS, AB Sciex, Boston, USA). Solid phase extractions (SPE) were performed with a Supelco Visiprep vacuum manifold (Sigma-Aldrich) using HLB cartridges. Solution pH was measured using a PHS3CW microprocessor pH/mV meter (Bante Instruments, Shanghai) coupled with an E201-C combined glass electrode.
2.4. Photochemical experiments
All photolysis experiments were carried out in the BL-GHX-V photoreactor under UV254 irradiation for 4 hours. For direct photolysis, a series of reaction solutions (50 mL Milli-Q water) containing 10 μM individual phenolic compound was prepared. Each reaction solution was phosphate buffered to circumneutral pH (7.0) and transferred to a cylindrical quartz reaction tube (2.2 cm i.d., 60 mL volume). The tubes were placed vertically around the cooling jacket at a fixed distance to ensure that each tube received uniform light radiation. For indirect photolysis, 100 μM NO2− was added to the above reaction solution, and the experimental procedure was identical. The effects of NOM on both direct and indirect photolysis were studied by adding different concentrations of SRNOM (1, 2, 5 mg L−1) to the reaction solutions. In some cases, the filtered wastewater was utilized as the water matrix to investigate its effects on both direct and sensitized photolysis of phenolic compounds. We also investigated the effects of i-PrOH (10 mM) on NO2−-mediated indirect photolysis to identify the contribution of HO˙ and RNS. Aliquot samples (1 mL) were withdrawn at predetermined time intervals and transferred to 1.5 mL amber HPLC vials. The samples were refrigerated at 4 °C in the dark and analyzed within 24 h by direct injection into the HPLC. Each experiment was repeated twice to ensure accurate data acquisition. Significant differences (p < 0.05) between different treatments were examined using one-way ANOVA via the SPSS statistical package (IBM SPSS Statistics 23).
2.5. Analytical methods
2.5.1. Spectroscopic characterization.
Aqueous solutions containing each phenolic pollutant (10 μM, buffered at pH 7.0) were scanned on a Varian Cary 50 UV-Vis spectrophotometer using 1 cm pathlength quartz cuvettes to obtain UV-Vis absorption spectra (200–450 nm). Fluorescence emission spectra were measured using an F-4500 fluorescence spectrophotometer using 1 cm pathlength fluorescent quartz cuvettes. The maximum absorption wavelength of each compound was used as the excitation wavelength of fluorescence.
2.5.2. HPLC-PAD analyses.
The concentrations of BPA, ATP, SAL and BP1 were measured using a Shimadzu HPLC. The mobile phase consisted of eluent A (H2O with 0.1% formic acid) and eluent B (MeOH with 0.1% formic acid) at a flow rate of 1 mL min−1. Detailed analytical parameters can be found in Table S3 of the ESI.†
2.5.3. LC-qTOF-MS/MS analyses.
Four reaction solutions each one (50 mL) containing 10 μM of certain phenolic compound and 100 μM NO2− were prepared and subjected to UV254 irradiation. The irradiation times for BPA, ATP, SAL, and BP1 were 8, 5, 6 and 8 h, respectively, to ensure more than 70% transformation percentage. The irradiated solutions were then subjected to SPE enrichment using HLB cartridges. Detailed SPE procedures can be referred to our previous studies.39
The SPE-concentrated samples were analyzed by LC-qTOF-MS/MS to identify the phenolic compounds and their TPs. Detailed analytical parameters are presented in Text S2 of the ESI.†
2.6. Calculations
The quantum yield (Φi, mol einstein−1) of direct photolysis of phenolic compounds was calculated using the following equation:40 | 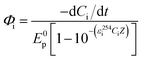 | (1) |
where Ci represents the concentration of target compound i (M), ε254i represents the molar absorption coefficient of target compound i at 254 nm (M−1 cm−1), E0p represents the incident photon irradiance of the LP-Hg lamp (einstein L−1 s−1), and Z represents the optical pathlength of the reaction tube (cm).
In the NO2−-sensitized photolysis, the rate constants of direct photolysis of phenolic compounds were normalized by light screening factor Sλ:41
| 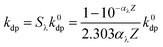 | (2) |
where
k0dp and
kdp represent the rate constant (h
−1) of direct photolysis of target compound
i in the absence and presence of NO
2−, respectively.
αλ represents the beam attenuation coefficient (cm
−1).
3. Results and discussion
3.1. Spectroscopic characterization
The UV-Vis absorption spectra of the aqueous solutions (10 μM, pH = 7) containing each phenolic compound (BPA, ATP, SAL, and BP1) are shown in Fig. S1 of ESI.† As can be seen, BPA has two absorption bands with maximum wavelengths (λmax) at ∼225 and ∼278 nm, respectively, corresponding to S0 → S2 (ππ*) and S0 → S1 (ππ*) transitions. ATP has an appreciable absorbance at ∼244 nm and an ill-defined shoulder peak at ∼275 nm, corresponding to S0 → S2 (ππ*) and S0 → S1 (ππ*) transitions, respectively.42 The UV-Vis absorption spectrum of SAL is similar to that of BPA, showing two absorption peaks at ∼225 and ∼278 nm, respectively. The electronic transition of SAL appears to be similar to that of BPA, probably because both of them contain phenolic structures with alkyl side chains and no other chromophores or conjugated structures. BP1 has three absorption bands with λmax at ∼243, ∼290, and ∼338 nm, respectively, corresponding to three π → π* transitions with different energy. The lowest energy absorbance corresponds to charge transfer from the hydroxyl oxygen to the carbonyl, and the higher energy absorbances to π → π* transitions localized on the phenyl moieties.43
The fluorescence properties of the four phenolic compounds were also studied; however, only SAL has measurable fluorescence (Fig. S2 of the ESI†). The fluorescence emission spectrum of SAL is consistent with that reported by Dodson et al.44 The unmeasurable fluorescence of BPA, ATP, and BP1 can be explained by their unique photophysical properties, as detailed in Text S3 of the ESI.†
3.2. Direct photolysis
Exposure to UV254 radiation resulted in the direct photolysis of phenolic compounds (10 μM) in neutral aqueous solutions (Fig. 1). The photolability of these phenolic compounds is consistent with their high electron density which favors electronic excitation.38 After 4 h of irradiation, the removal percentages of these phenolic compounds followed the order of ATP (80.6%) > BP1 (64.4%) > SAL (57.7%) > BPA (34.4%). The photolysis reactions followed pseudo-first-order kinetics, and the observed reaction rate constants (kobs) were 0.44, 0.24, 0.22, and 0.11 h−1, respectively. It is apparent that the photochemical reactivity of ATP was the highest, followed by BP1 and SAL, and the reactivity of BPA was the lowest.
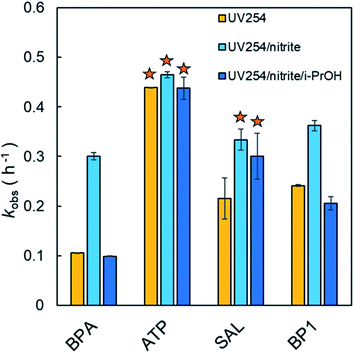 |
| Fig. 1 The observed pseudo-first-order rate constant (kobs) of direct (UV254) and indirect (NO2− sensitized, UV254/nitrite) photolysis of phenolic compounds under UV254 irradiation, as well as the effects of radical scavenger i-PrOH on the indirect photolysis (UV254/nitrite/i-PrOH). Experimental conditions: 10 μM phenolic compound BPA/ATP/SAL/BP1, 100 μM NO2−, 10 mM i-PrOH, solution pH 7.0. Error bars represent the standard deviation of duplicates. Asterisks represent no statistically significant difference (p > 0.05) was observed between data sets. | |
As shown in eqn (1), the photolysis rate −dCi/dt is a function of ε254i and Φi. Therefore, the different photoreactivity of phenolic compounds is due to their distinct values of ε254i and Φi. According to the Lambert–Beer law, the ε254i values of phenolic compounds were determined to be BP1 (8700 M−1 cm−1) > ATP (7650 M−1 cm−1) > BPA (680 M−1 cm−1) > SAL (460 M−1 cm−1). In general, a molecule with chromophore and/or strong conjugation tends to have a large ε254i value. BP1 has the largest ε254i value which can be explained by the benzophenone chromophore in its molecular structure. Benzophenone derivatives are known to have strong absorbances due to the conjugation between the diaryl and ketone group.45 In addition, the charge transfer from the hydroxyl oxygen to the carbonyl increases the light absorption capacity.43 The high ε254i value of ATP may be due to the presence of the phenolic chromophore and the electron-donating secondary amine. The lone pair electrons in the amine group can be delocalized to the benzene ring, thus enhancing the light-absorbing ability. The lower ε254i values of BPA and SAL are mainly attributed to the lack of conjugation in their molecular structures. As expected, the alkyl side chains of BPA and SAL cannot form conjugation with the phenolic moiety, resulting in their weak absorbances at 254 nm.
According to eqn (1), Φi values of BPA, ATP, SAL and BP1 under UV254 irradiation were calculated to be 0.001, 0.0044, 0.029, and 0.0014 mol einstein−1, respectively. The Φi values of BPA and ATP were of the same order of magnitude as those reported in the literature ((0.655 ± 0.276) × 10−2 and 0.18 × 10−2 mol einstein−1, respectively).46 The low Φi value of BPA may be ascribed to the “loose bolt” and “free rotor” effects of the isopropyl side chain, which makes the excited state easily deactivated.45 For example, the C–H bonds of the isopropyl group can increase the nonradiative relaxation of the excited state through stretching vibration or rotation, thereby reducing the possibility of photochemical reactions. The higher Φi value of ATP may be attributed to the fact that excited ATP is susceptible to bond cleavage followed by rearrangement (photo-Fries rearrangement) under UV254 irradiation.42,47 Among the phenolic compounds studied, SAL has the highest quantum yield. While the reason is unclear, one possible explanation is that the population of the SAL triplet state occurs at a fast rate constant (i.e., ∼1.7 × 1010 s−1), which may be favorable for further chemical reactions.44 The lowest Φi of BP1 is possibly due to the formation of intra- or intermolecular hydrogen bonds, which favors the deactivation via excited state proton transfer (ESPT) as well as the formation of the charge transfer state (CT state).48 Interestingly, efficient deactivation of the BP1 excited state is beneficial for its use as a sunscreen active ingredient.49
3.3. NO2−-sensitized indirect photolysis
In the presence of 100 μM NO2−, the photolysis rate constants of the phenolic compounds were accelerated to a different degree (except for ATP, p > 0.05) (Fig. 1). The enhanced photolysis rate constants in the presence of NO2− could be attributed to the involvement of reactive species (e.g., HO˙, NO2˙, NO˙, etc.) generated under UV254 irradiation ((R1)–(R3)).24,26 The phenolic compounds used in this study have high electron density and therefore appear to be susceptible toward electrophilic attack by reactive species. As can be seen from Fig. 1, the most obvious photosensitizing effect was observed for BPA, followed by SAL and BP1. Compared with direct photolysis, the kobs values of BPA, SAL, and BP1 were increased by 2.83- (0.106 to 0.300 h−1), 1.55- (0.215 to 0.334 h−1), and 1.50-fold (0.241 to 0.362 h−1), respectively, in the presence of NO2−. NO2− had no statistically significant photosensitizing effect on ATP degradation (p > 0.05), as kobs was only increased by 1.06-fold (0.439 to 0.465 h−1). These results suggest that the sensitizing effect of NO2− on the photolysis of phenolic compounds is species-dependent, which warrants further investigations.
The direct photolysis rate constants of phenolic compounds in the presence of NO2− could be corrected using light screening factor Sλ.41 The amount of indirect photolysis was calculated as the difference between kobs (total photolysis in the presence of NO2−) and Sλk0dp (Sλ normalized direct photolysis in the absence of NO2−). Therefore, the relative contributions of direct and indirect photolysis could be distinguished. The results reveal that ∼99.7% of light was transmitted and only a small amount (∼0.3%) was screened out by NO2−. As illustrated in Table 1, in the presence of NO2−, both direct and indirect photolysis contributed to the degradation of BPA (35% direct and 65% indirect photolysis) and BP1 (43% direct and 57% indirect photolysis). Among them, indirect photolysis was greater than direct photolysis. This finding suggests that the promoting effect of indirect photolysis driven by NO2− photosensitization overwhelmed the light screening effect of NO2−. This conclusion is in accordance with the product distribution as various hydroxylated and nitrated products were detected by HRMS (Fig. 2). While the addition of NO2− also had a promoting effect on SAL photolysis, direct photolysis was observed to be predominant (64% direct and 36% indirect photolysis). This may be related to the fact that SAL has the largest Φi, indicating that the substance is capable of utilizing photons efficiently for photochemical reactions. In contrast, direct photolysis was the dominant pathway for ATP degradation even in the presence of NO2− (94% direct and 6% indirect photolysis), which was most likely due to its high ε254i and moderate Φi. It should be noted that while NO2− did not show significant photosensitizing effect on the degradation of ATP, it could alter the product distribution of this compound (Fig. S3, ESI†).
Table 1 Kinetic parameters accounting for the influence of NO2− on the photolysis of phenolic compounds under UV254 irradiationh
Compound |
k
0dp (h−1)a |
S
λ
|
k
obs
(h−1) |
k
dp
(h−1) |
k
ip
(h−1) |
k
dp
(%) |
k
ip
(%) |
k
0dp represents the direct photolysis rate constant of phenolic compounds in the absence of NO2−.
Light screening factor as calculated from eqn (2).
k
obs represents the observed pseudo-first-order rate constant for the photolysis of phenolic compounds in the presence of NO2−.
k
dp represents the direct photolysis rate constant of phenolic compounds in the presence of NO2−.
k
ip represents the rate constant of indirect photolysis of phenolic compounds in the presence of NO2−.
The contribution percentage of direct photolysis.
The contribution percentage of indirect photolysis, which is calculated as a difference between kobs (total photolysis in the presence of NO2−) and Sλk0dp (Sλ normalized direct photolysis in the absence of NO2−).
Data represent the mean values of duplicate experiments, and uncertainties are generally within 10% unless otherwise stated.
|
BPA |
0.1059 |
0.9954 |
0.3001 |
0.1054 |
0.1947 |
35.11 |
64.89 |
ATP |
0.4388 |
0.9969 |
0.4646 |
0.4368 |
0.0278 |
94.01 |
5.99 |
SAL |
0.2151 |
0.9967 |
0.3339 |
0.2141 |
0.1198 |
64.12 |
35.88 |
BP1 |
0.2414 |
0.9970 |
0.3621 |
0.2403 |
0.1218 |
66.36 |
33.64 |
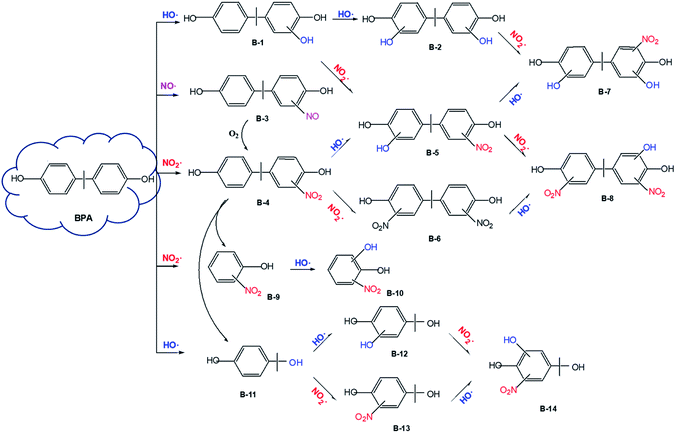 |
| Fig. 2 Transformation pathways for the NO2−-sensitized photolysis of BPA in aqueous solution under UV254 irradiation. | |
To further discern the relative contribution of HO˙ and RNS to the NO2−-sensitized photolysis of phenolic compounds, excess i-PrOH (10 mM) was spiked into the reaction solutions. i-PrOH is a well-known quencher of HO˙ (kHO˙,i-PrOH = 1.9 × 109 M−1 s−1).50 In addition, since NO2˙ is mainly derived from the reaction of HO˙ with NO2− in the UV254/NO2− system, the scavenging of HO˙ by i-PrOH results in the elimination of NO2˙.28 As shown in Fig. 1, the inhibitory effects of i-PrOH were found to be significant for BPA and BP1, suggesting that HO˙ and NO2˙ were leading oxidants responsible for their indirect photolysis. The lack of a strong inhibitory effect on ATP photolysis in the presence of i-PrOH was conceivable since direct photolysis was the predominant pathway (Fig. 1). In the case of SAL, no statistically significant difference (p > 0.05) was observed for NO2−-sensitized photolysis in the absence and presence of i-PrOH. Considering the uncertainties in these measurements, it is inconclusive that HO˙ and NO2˙ were the dominant species during NO2− photosensitization. Other reactive species such as NO˙ might also be involved.28
3.4. Reactivity of phenolic compounds with HO˙ and NO2˙
According to the above results, reactive radicals such as HO˙ and NO2˙ played critical roles in the NO2−-sensitized photolysis of phenolic compounds, including BPA, and BP1. In general, the second-order rate constants between phenolic compounds and HO˙ approach the diffusion-controlled limit (∼7.4 × 109 M−1 s−1) in water (Table 2), consistent with the non-selectivity of HO˙.51
Table 2 Second order rate constants for reactions of phenolic compounds with HO˙ or NO2˙
Phenolic compound |
k
HO˙,PC (M−1 s−1) |
k
NO2˙,PC (M−1 s−1) |
From Xiao et al., (2017).51
From de Laurentiis et al., (2014).10
From Zhou et al., (2017).60
From Ge et al., (2019).62
From Wang et al., (2021).52
From this work.
|
BPA |
7.20 × 109a |
2.25 × 104f |
ATP |
1.87 × 109b |
1.48 × 103e |
SAL |
5.30 × 109c |
1.35 × 104f |
BP1 |
4.16 × 109d |
2.44 × 104f |
The steady-state concentration of NO2˙, [NO2˙]SS, could be calculated from the following equation (for detailed derivation, please refer to Text S4 of the ESI†):
| 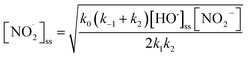 | (3) |
where
k1,
k−1, and
k2 are the rate constants of forward, reverse, and hydrolysis reactions of N
2O
4, respectively.
26k0 is the second-order rate constant between HO˙ and NO
2− (see
(R3)), [NO
2−] is the molar concentration of NO
2−, and [HO˙]
SS is the steady-state concentration of HO˙. Through kinetic modeling, we calculated the second-order rate constant for reaction of NO
2˙ with phenolic compounds (
kNO2˙,PC =
kNO2˙/[NO
2˙]
ss,
kNO2˙ is the reaction rate constant contributed by NO
2˙), as tabulated in
Table 2.
It should be noted that we did not determine the second-order rate constant of ATP with NO2˙ since ATP was predominantly attenuated by direct photolysis (Fig. 1). The kNO2˙,PC value of ATP shown in Table 2 was obtained from the literature.52 As can be seen, the kNO2˙,PC values of the other three studied phenolic compounds are of the same order of magnitude as that of 4-chlorophenol (1.1 × 104 M−1 s−1),26,35 but are one-order of magnitude higher than that of phenol (3.2 × 103 M−1 s−1) and the antibiotic sulfamethoxazole (6.2 × 103 M−1 s−1).29 The higher reactivity of phenolic compounds with NO2˙ is likely due to the electron-rich phenolic moiety, which is conducive to the electrophilic attack of NO2˙. Therefore, our results highlight the potential photosensitizing role of NO2− in the attenuation of effluent-derived phenolic contaminants during the UV254 disinfection process.
3.5. Products and mechanisms
NO2−-sensitized photolysis of phenolic compounds often produces carcinogenic/mutagenic nitrated and nitrosated byproducts, which should be scrutinized.12,28,29 In this study, the intermediate products produced by NO2−-sensitized photolysis under UV254 irradiation were concentrated by SPE and then identified by HRMS. The assignment of photoproducts was based on the following criteria: (i) the detected exact masses with errors less than 5 ppm of the theoretical exact masses, (ii) the fragmentation patterns of molecular ions (MS2 data) (if available due to the information-dependent acquisition, IDA-MS/MS), and (iii) comparison with mass spectrometric data reported in the literature. Note that for those photoproducts whose MS2 data were not available, their structural assignment was dependent on retention times and the corresponding MS1 spectra, which was highly tentative and uncertain. Overall, a series of transformation products including hydroxylated, nitrated, nitrosated, dimerized, and bond-cleavage products were identified (Tables S4–S7, ESI†). The detection of these products generally supports the argument in favor of HO˙ and RNS (NO2˙, NO˙) as reactive species. However, it is worth noting that the formation of dimeric products also suggests the occurrence of direct photolysis, at least to some extent ((R4) and (R5)). On the basis of the identified products, we attempted to elucidate the underlying mechanisms for NO2−-sensitized photolysis of phenolic compounds. | HPhOH + hv → HPhO˙ + H˙ (e− + H+) | (R4) |
3.5.1. Bisphenol A (BPA).
Four reaction pathways are proposed for NO2−-sensitized photolysis of BPA, as shown in Fig. 2. As expected, HO˙ produced by NO2− photolysis leads to the hydroxylation of phenolic compounds. The electrophilic attack of HO˙ at the benzene ring can generate the monohydroxylated product (B-1). The initial hydroxylation increases the electron density of the molecule, which is conducive to further attack by HO˙, giving rise to the dihydroxylated product (B-2). Subsequent attack by HO˙ may generate polyhydroxylated intermediates or ring opening products such as carboxylic acids, but their identification is out of the scope of this work.
On the other hand, NO2˙ generated in the UV254/NO2− system is responsible for the nitration of the parent compound to form B-4 (Fig. S4, ESI†).53,54 The nitration process is likely initiated through H-abstraction, which generates the phenoxyl radical as a critical intermediate.35,37 Subsequently, another NO2˙ couples with the nascent phenoxyl radical to generate nitrocyclohexa-2,5-dienone, which undergoes rearrangement with the assistance of H2O, resulting in the formation of a nitro derivative.55 Furthermore, the nitrated intermediate undergoes continuous electrophilic substitution under the attack of HO˙ or NO2˙, generating hydroxyl-nitro- or dinitro-derivatives (B-5 and B-6).53,54 Further hydroxylation or nitration of B-5 or B-6 leads to the formation of dihydroxyl-nitro- or hydroxyl-dinitro-derivatives (B-7 and B-8). These intermediates have previously been identified during electrochemical oxidation of BPA in the presence of nitrite.56
HRMS analyses also identified a nitrosated product (B-3), indicating the involvement of NO˙. The nitrosation process is expected to proceed through coupling between NO˙ and the phenoxyl radical, a way very similar to the above nitration process. It is noteworthy that the nitrosated product is chemically unstable and can be oxidized to the nitrated product in the presence of O2.25
The cleavage of the C–C bond between the isopropyl chain and benzene ring of BPA is another important pathway for NO2−-sensitized photolysis of BPA. The detection of α-methyl-α-(4-hydroxylphenyl) ethanol (B-11) supports the C–C bond cleavage mechanism.54,56 As expected, B-11 undergoes further hydroxylation/nitration under the attack of HO˙ or NO2˙, resulting in the formation of B-12, B-13, and B-14. Another product of C–C bond cleavage should be phenol or hydroquinone; however, we did not detect these compounds. The failure to detect phenol or hydroquinone was possibly due to their quick transformation under the attack of HO˙ or NO2˙. C–C bond cleavage also likely occurrs in nitro-BPA (B-4) since nitrophenol (most likely 2-nitrophenol, B-9) was identified by HRMS.56B-9 can be converted to B-10 under the electrophilic attack of HO˙.
3.5.2. Acetaminophen (ATP).
ATP was transformed mainly through direct photolysis pathway even in the presence of NO2−. The detection of A-1, a photo-Fries rearrangement product, evidenced the direct photolysis of ATP under UV254 irradiation.42,47 In addition, hydroxylated, nitrated, nitrosated, amide chain cleavage, and dimerized products were also detected. These products showed that although the addition of NO2− did not appreciably promote the photolysis of ATP (Fig. 1), it obviously altered the distribution of products. According to these detected products, we deduce the photolysis pathway of ATP in the UV254/NO2− system (Fig. S3, ESI†). As expected, HO˙, NO˙, and NO2˙ mediate the transformation of ATP to produce hydroxylated (A-2), nitrosated (A-4), and nitrated (A-5, Fig. S5 of ESI†) derivatives respectively. These products can then be hydroxylated or nitrated again to form dihydroxyl-, hydroxyl-nitro- or dinitro-products (A-3, A-6, and A-7). HRMS analyses also identified p-aminophenol (A-8), p-nitrophenol (A-9) and its hydroxylated/nitrated products (A-10, A-11, and A-12), which was consistent with a previous study by Moctezuma et al.57 Under UV254 irradiation, the amide chain of the excited ATP is subjected to cleavage (possibly through the lowest lying singlet excited state), generating a geminate radical pair (RP)gem in a solvent cage (i.e., anilino and acetyl radicals). The (RP)gem can either recombine in the solvent cage (i.e., photo-Fries rearrangement) or escape from the cage to form free radicals.42 The free anilino radical can extract a H-atom from H-donors to produce p-aminophenol (A-8). Further attack of HO˙ can oxidize the amino group to nitro group to produce p-nitrophenol (A-9).57,58 Finally, we also detected the dimer of ATP (A-13). This is possibly because the active species produced by UV254/NO2− react with ATP through H-abstraction to form phenoxyl radicals.11 Note that, the direct photolysis of ATP may also likely yield phenoxyl radicals.42 The phenoxyl radical can couple either with itself or with its resonance radicals (radicals with unpaired electrons located at different positions) to generate dimers. According to different coupling modes (i.e., C–C or C–O coupling), there may be different isomers of the dimer.59 Structural assignments of the dimers are beyond the scope of this study. The dimers may be further hydroxylated, nitrosated, and nitrated to produce a series of dimer derivatives (A-14–A-19).
3.5.3. Salbutamol (SAL).
The transformation pathways of SAL in the UV254/NO2− system include hydroxylation, nitration, nitrosation, and alkyl chain cleavage (Fig. 3). The molecular structure of SAL contains hydroxymethyl (–CH2OH) and 2-(tert-butylamino) ethanol (–CH(OH)CH2NHC(CH3)3) side chains. Both of them can activate the benzene ring via the electron-donating effect,60 which is conducive to the addition of HO˙, thus generating the hydroxylated product (S-1). However, HRMS analyses only detected one dihydroxylated product. The absence of the monohydroxylated product indicates a rapid transformation of this intermediate under the attack of reactive species. Similar to other phenolic compounds, SAL reacts with NO2˙ and NO˙ to generate nitrated (S-2, Fig. S6 of the ESI†) and nitrosated (S-4) products, respectively. Further attack of HO˙ at S-2 leads to the formation of the hydroxyl-nitro-derivative S-3. As mentioned above, the presence of dissolved oxygen may facilitate the transformation of nitrosated product S-4 to generate nitrated product S-2.25
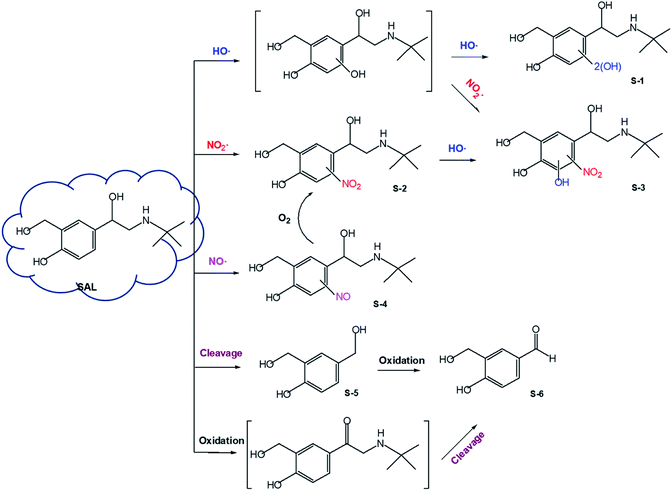 |
| Fig. 3 Transformation pathways for the NO2−-sensitized photolysis of SAL in aqueous solution under UV254 irradiation. The molecules in square brackets represent the products not identified in this work but were possibly generated. | |
SAL also undergoes the cleavage of the alkyl side chain, resulting in the formation of S-5. Such a reaction may be initiated by HO˙ attack at the C2 position of the 2-(tert-butylamino) ethanol side chain, which produces an ethylene glycol intermediate. The C1–C2 bond of the intermediate is unstable and may break under further attack by free radicals, giving rise to S-5. Another product of the alkyl chain cleavage is expected to be (tert-butylamino) methanol that was not detected by HRMS analyses. The nascent hydroxymethyl group of S-5 may undergo H-extraction under the attack of HO˙, and therefore, be oxidized to an aldehyde group (S-6). It should be noted that the alcohol group in the 2-(tert-butylamino) ethanol chain of SAL is likely to be initially oxidized to a carbonyl group (2-(tert-butylamino)-1-(-4-hydroxyl-3-hydroxymethy-phenyl) ethanone) by free radicals or photolysis.61 The carbonyl intermediate can undergo the Norrish I reaction to produce S-6 as well.61
3.5.4. 2,4-Dihydroxybenzophenone (BP1).
Based on the identified products, we propose that there are five transformation pathways for NO2−-sensitized photolysis of BP1, including hydroxylation (P-1, P-2), nitration (P-3 (Fig. S7, ESI†), P-4), nitrosation (P-5), dimerization (P-8), and chain cleavage (P-6, P-7), respectively (Fig. S8, ESI†). The mechanisms for hydroxylation, nitration, and nitrosation are believed to be similar to other phenolic compounds and therefore are not discussed further. It should be noted that the benzene ring and resorcinol moiety of BP1 have different reactivity. The latter contains two phenolic hydroxyl groups with electron-donating effect, making it more electron-rich than the former. Thus, the resorcinol moiety is more vulnerable toward electrophilic attack by HO˙, NO2˙, and NO˙.62
The photoinduced chain cleavage of BP1 under UV245 irradiation is a typical Norrish I reaction. The Norrish I reaction of aromatic ketones, also known as the α-cleavage reaction, begins with the electronic overlap between the σ orbital of the α-C–C bond (occupied by two electrons) and the n orbital of the excited carbonyl bond ([C
O]*, occupied by a single electron).45 Due to the electron-rich properties of the phenolic group, the α-C–C bond between C
O and resorcinol has a higher electronic overlap, which is more susceptible toward α-cleavage. Therefore, the α-cleavage reaction prefers to occur at the resorcinol side.63 This speculation is further supported by the detection of benzoic acid (P-6) and resorcinol (P-7) by HRMS analyses. As discussed earlier, the dimerized product was probably derived from the cross-coupling of BP1, with phenoxyl radical and its resonance phenyl radical being important intermediates.62 In the present study, HRMS only detected the hydroxylated dimer but not the dimer. This result can be rationalized by the high electron density on the benzene ring of the dimer, which was conducive to HO˙ attack.62
3.6. Influences of NOM
Natural organic matter (NOM) that is ubiquitously present in natural waters plays dual roles in the photochemical degradation of phenolic contaminants.64 On the one hand, NOM serves as a photosensitizer that mediates the generation of PPRI, such as the triplet excited state NOM (3NOM*), singlet oxygen (1O2), and HO˙, which promote the photodegradation of pollutants.64,65 On the other hand, NOM also competes with target pollutants for absorbing photons, quenching reactive species (e.g., HO˙, 3NOM*, etc.), thus showing inhibitory effects.66
In this experiment, SRNOM was selected as a representative aquatic NOM, and its effects on both direct and NO2−-sensitized photolysis of the four phenolic contaminants under UV254 irradiation were systematically studied. As can be seen, the presence of SRNOM exhibited a non-measurable promoting effect on the direct photolysis of BPA under UV254 irradiation (Fig. 4a, left panel). This result indicates that 3NOM* and/or ROS as active species had no significant effects on the photolysis of BPA (p > 0.05). It is possible that light-screening and radical-quenching effects of SRNOM became dominant which inhibited the photolysis of BPA.54 For NO2−-sensitized photolysis of BPA, the presence of SRNOM showed an inhibitory effect, and the inhibition was exacerbated with increasing concentration of SRNOM (Fig. 4a, right panel). This may be because SRNOM competed with BPA and NO2− for photon absorption, thus inhibiting both the direct photolysis of BPA and the generation of active species (HO˙, NO2˙, NO˙, etc.) by NO2− photolysis. Calculation of the screening factor Sλ using eqn (2) suggested that 5.82 to 23.11% of light could be filtered as the SRNOM concentration increased from 1 to 5 mg L−1 (Table S8, ESI†). Furthermore, the electron-donating phenolic moieties in SRNOM molecules could result in back-reduction of the radical intermediates (e.g., radical cations) to their parent compounds, which might also contribute to the inhibition to some extent.67
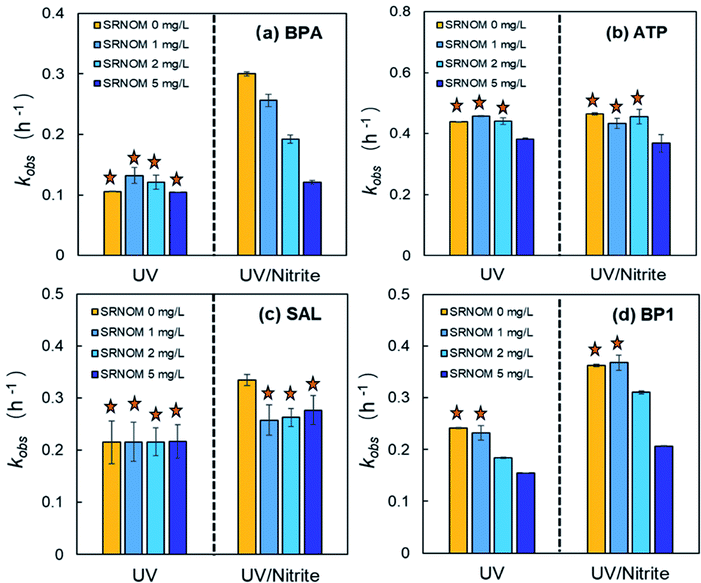 |
| Fig. 4 Effects of SRNOM (as a representative of aquatic NOM) with varying concentrations on the direct and NO2−-sensitized photolysis of phenolic compounds ((a) BPA, (b) ATP, (c) SAL, and (d) BP1) under UV254 irradiation. Experimental conditions: 10 μM phenolic compound BPA/ATP/SAL/BP1, 100 μM NO2−, 0–5 mg L−1 SRNOM, solution pH 7.0. Error bars represent the standard deviation of duplicates. Asterisks represent no statistically significant difference (p > 0.05) was observed between data sets. | |
Due to the dual roles of NOM, SRNOM at low concentrations (0–2 mg L−1) had no significant difference on the direct photolysis of ATP under UV254 irradiation (p > 0.05), which was consistent with previous reports.10,11 The direct photolysis of ATP was slightly inhibited in the presence of high concentration NOM (5 mg L−1) (Fig. 4b, left panel). Similarly, SRNOM at low concentrations had no measurable effect on the NO2−-sensitized photolysis (Fig. 4b, right panel), which was mainly attributed to its light-screening effect considering that direct photolysis was the dominant transformation pathway.
Interestingly, the addition of SRNOM had no impact on the direct photolysis rate constant of SAL under UV254 irradiation (p > 0.05) (Fig. 4c, left panel). This may be because the promoting effect of SRNOM offset the inhibiting effect. In contrast, the presence of SRNOM showed an inhibitory impact on NO2−-sensitized photolysis of SAL. However, no statistically significant difference was observed with increasing concentration of SRNOM (p > 0.05) (Fig. 4c, right panel). The reason for this phenomenon is unclear which needs to be further explored.
Regardless of direct or NO2−-sensitized photolysis, the presence of SRNOM showed an inhibiting effect on the photolysis of BP1, and the inhibition was increased with increasing SRNOM concentration (Fig. 4d), supporting the roles of SRNOM as a light-screening and radical-quenching agent. Interestingly, the limited effect of SRNOM seemed similar to the inhibitory effect of i-PrOH on the same phenolic compound (Fig. 1). This result possibly indicates that SRNOM inhibited the NO2−-sensitized photolysis mainly through radical quenching, analogous to i-PrOH.
3.7. Photolysis in the wastewater matrix
The kobs values corresponding to both direct and NO2−-sensitized photolysis of BPA, SAL and BP1 in wastewater were all higher than those in Milli-Q water (p < 0.05, Fig. 5). This is possibly because the presence of photosensitizers, such as NO3− and effluent-derived organic matter (EfOM), promoted the indirect photolysis of pollutants by PPRI (e.g., 3EfOM*, 1O2, HO˙, etc.).68 In the case of ATP, the wastewater matrix inhibited its direct photolysis but increased its indirect photolysis by NO2− sensitization (p < 0.05). As discussed above, the transformation of ATP was dominated by direct photolysis (94%), therefore, the inhibition of ATP direct photolysis in wastewater was mainly due to the light-screening effect of wastewater constituents.
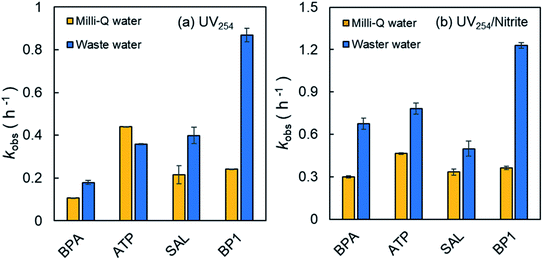 |
| Fig. 5 Effects of water matrices on the (a) direct and (b) NO2−-sensitized photolysis of phenolic compounds under UV254 irradiation. Experimental conditions: 10 μM phenolic compound BPA/ATP/SAL/BP1, 100 μM NO2−, solution pH 7.0. Error bars represent the standard deviation of duplicates. The characteristics of wastewater can be found in Table S2, ESI.† Note that statistically significant difference was observed between experimental data obtained in Milli-Q water and wastewater (p < 0.05). | |
In addition, in the UV254/NO2−/wastewater system (Fig. 5b), the photolysis rate constants of the target compounds were significantly higher than those of the direct photolysis (UV254/wastewater, p < 0.05) (Fig. 5a), highlighting the role of NO2− as a photosensitizer in attenuating phenolic contaminants in real wastewater. It should be noted that the photolysis rate constants of target compounds in the UV254/NO2−/wastewater system were also higher than those obtained in the UV254/NO2−/Milli-Q water system (Fig. 5b). This result can be ascribed to the photosensitizing effects of wastewater constituents such as NO3− (5.348 mg L−1) and EfOM (27 mg L−1 as TOC) ((R6)–(R8)).
|  | (R6) |
| 3EfOM* + O2 → EfOM + 1O2 | (R8) |
4. Conclusions and environmental implications
Exposure to UV254 radiation resulted in efficient removal of BPA, ATP, SAL and BP1 in aqueous solution in the absence or presence of NO2−. The photochemical reactivity of these phenolic compounds are derived from the chromophores and conjugated structures present in their molecular structures. Molar extinction coefficient (ε254i) and quantum yield (Φi) are two important parameters determining the direct photolysis rate of phenolic compounds. The ε254i values of BPA, ATP, SAL, and BP1 were measured to be 680, 7650, 470, and 8700 M−1 cm−1, respectively, and their quantum yields were determined to be 0.01, 0.0044, 0.0290, and 0.0014 mol einstein−1, respectively. The presence of NO2− significantly accelerated the photolysis of BPA, SAL, and BP1. In addition, light screening factor calculation and the radical quenching study suggested that HO˙ and NO2˙ played important roles in the NO2−-sensitized photolysis of BPA and BP1. The second-order rate constants between NO2˙ and the phenolic compounds were determined to be within (1.35–2.44) × 104 M−1 s−1 by kinetic modeling. For ATP, direct photolysis was the dominant pathway accounting for its attenuation under UV254 irradiation in the presence of NO2−. SPE coupled with HRMS analyses identified a series of intermediate products, including hydroxylated, nitrated, nitrosated, dimerized, and alkyl side chain cleavage products. SRNOM played complex roles in direct and NO2−-sensitized photolysis of phenolic compounds by serving as a photosensitizer, light screening and radical quenching agent. Wastewater constituents, such as NO3− and EfOM, could promote direct and NO2−-sensitized photolysis of BPA, SAL, and BP1 in the wastewater matrix. While NO2− could accelerate the photolysis of phenolic compounds in both Milli-Q and wastewater matrices, the potential formation of carcinogenic and mutagenic nitrated/nitrosated derivatives should be scrutinized. Therefore, photolysis may play a dual role in the attenuation of phenolic compounds during wastewater UV disinfection.
Conflicts of interest
There are no conflicts to declare.
Acknowledgements
The authors gratefully acknowledge financial support from the National Natural Science Foundation of China (Grant No. 22076080). We greatly appreciate the anonymous reviewers for their valuable comments and constructive suggestions.
References
- W. Zhao, G. Yu, L. Blaney and B. Wang, Development of emission factors to estimate discharge of typical pharmaceuticals and personal care products from wastewater treatment plants, Sci. Total Environ., 2021, 769, 144556 CrossRef CAS.
- T. A. Ternes, Occurrence of drugs in German sewage treatment plants and rivers, Water Res., 1998, 32(11), 3245–3260 CrossRef CAS.
- S. Rashid and Y.-Q. Liu, Comparison of life cycle toxicity assessment methods for municipal wastewater treatment with the inclusion of direct emissions of metals, PPCPs and EDCs, Sci. Total Environ., 2021, 756, 143849 CrossRef CAS.
- I. Michael, L. Rizzo, C. S. McArdell, C. M. Manaia, C. Merlin, T. Schwartz, C. Dagot and D. Fatta-Kassinos, Urban wastewater treatment plants as hotspots for the release of antibiotics in the environment: a review, Water Res., 2013, 47, 957–995 CrossRef CAS.
- M. M. P. Tsui, H. W. Leung, P. K. S. Lam and M. B. Murphy, Seasonal occurrence, removal efficiencies and preliminary risk assessment of multiple classes of organic UV filters in wastewater treatment plants, Water Res., 2014, 53, 58–67 CrossRef CAS.
- S. K. Behera, H. W. Kim, J.-E. Oh and H.-S. Park, Occurrence and removal of antibiotics, hormones and several other pharmaceuticals in wastewater treatment plants of the largest industrial city of Korea, Sci. Total Environ., 2011, 409(20), 4351–4360 CrossRef CAS.
- Y. Kawamura, Y. Ogawa, T. Nishimura, Y. Kikuchi, J. Nishikawa, T. Nishihara and K. Tanamoto, Estrogenic activities of UV stabilizers used in food contact plastics and benzophenone derivatives tested by the yeast two-hybrid assay, J. Health Sci., 2003, 49, 205–212 Search PubMed.
- K. Kümmerer, The presence of pharmaceuticals in the environment due to human use – present knowledge and future challenges, J. Environ. Manage., 2009, 90(8), 2354–2366 Search PubMed.
- P. Calza, D. Vione, A. Novelli, E. Pelizzetti and C. Minero, The role of nitrite and nitrate ions as photosensitizers in the phototransformation of phenolic compounds in seawater, Sci. Total Environ., 2012, 439, 67–75 CrossRef CAS.
- E. De Laurentiis, C. Prasse, T. A. Ternes, M. Minella, V. Maurino, C. Minero, M. Sarakha, M. Brigante and D. Vione, Assessing the photochemical transformation pathways of acetaminophen relevant to surface waters: Transformation kinetics, intermediates, and modelling, Water Res., 2014, 53, 235–248 CrossRef CAS.
- Y. Li, Y. Pan, L. Lian, S. Yan, W. Song and X. Yang, Photosensitized degradation of acetaminophen in natural organic matter solutions: the role of triplet states and oxygen, Water Res., 2017, 109, 266–273 CrossRef CAS.
- S. Zhou, L. Li, Y. Wu, S. Zhu, N. Zhu, L. Bu and D. D. Dionysiou, UV365 induced elimination of contaminants of emerging concern in the presence of residual nitrite: roles of reactive nitrogen species, Water Res., 2020, 178, 115829 CrossRef CAS.
- J. C. Kruithof, P. C. Kamp and B. J. Martijn, UV/H2O2 treatment: a practical solution for organic contaminant control and primary disinfection, Ozone: Sci. Eng., 2007, 29(4), 273–280 CrossRef CAS.
- S. Canonica, L. Meunier and U. von Gunten, Phototransformation of selected pharmaceuticals during UV treatment of drinking water, Water Res., 2008, 42, 121–128 CrossRef CAS.
- E. R. Blatchley, S. C. Weng, M. Z. Afifi, H. H. Chiu, D. B. Reichlin, S. Jousset and R. S. Erhardt, Ozone and UV254 radiation for municipal wastewater disinfection, Water Environ. Res., 2012, 84, 2017–2027 CrossRef CAS.
- I. Kim and H. Tanaka, Photodegradation characteristics of PPCPs in water with UV treatment, Environ. Int., 2009, 35(5), 793–802 CrossRef CAS.
- M. Dong and F. L. Rosario-Ortiz, Photochemical formation of hydroxyl radical from effluent organic matter, Environ. Sci. Technol., 2012, 46, 3788–3794 CrossRef CAS.
- S. Mostafa and F. L. Rosario-Ortiz, Singlet oxygen formation from wastewater organic matter, Environ. Sci. Technol., 2013, 47, 8179–8186 CrossRef CAS.
- A. L. Boreen, W. A. Arnold and K. McNeill, Photodegradation of pharmaceuticals in the aquatic environment: a review, Aquat. Sci., 2003, 65(4), 320–341 CrossRef CAS.
- S. Yan and W. Song, Photo-transformation of pharmaceutically active compounds in the aqueous environment: a review, Environ. Sci.: Processes Impacts, 2014, 16(4), 697–720 RSC.
- D. Vione, M. Minella, V. Maurino and C. Minero, Indirect photochemistry in sunlit surface waters: photoinduced production of reactive transient species, Chem.–Eur. J., 2014, 20, 10590–10606 CrossRef CAS.
- O. S. Keen, N. G. Love and K. G. Linden, The role of effluent nitrate in trace organic chemical oxidation during UV disinfection, Water Res., 2012, 46, 5224–5234 CrossRef CAS.
- P. I. Hora, P. J. Novak and W. A. Arnold, Photodegradation of pharmaceutical compounds in partially nitritated wastewater during UV irradiation, Environ. Sci.: Water Res. Technol., 2019, 5, 897–909 RSC.
- F. Machado and P. Boule, Photonitration and photonitrosation of phenolic derivatives induced in aqueous solution by excitation of nitrite and nitrate ions, J. Photochem. Photobiol., A, 1995, 86, 73–80 CrossRef CAS.
- D. Vione, V. Maurino, C. Minero and E. Pelizzetti, New process in the environmental chemistry of nitrite: nitration of phenol upon nitrite photoinduced oxidation, Environ. Sci. Technol., 2002, 36, 669–676 CrossRef CAS.
- C. Minero, S. Chiron, G. Falletti, V. Maurino, E. Pelizzetti, R. Ajassa, M. E. Carlotti and D. Vione, Photochemical processes involving nitrite in surface water samples, Aquat. Sci., 2007, 69, 71–85 CrossRef CAS.
- G. Marussi and D. Vione, Secondary formation of aromatic nitroderivatives of environmental concern: photonitration processes triggered by the photolysis of nitrate and nitrite ions in aqueous solution, Molecules, 2021, 26, 2550 CrossRef CAS.
- Y. Li, H. Qin, Y. Li, J. Lu, L. Zhou, J. M. Chovelon and Y. Ji, Trace level nitrite sensitized photolysis of antimicrobial agents parachlormetaxylenol and chlorophene in water, Water Res., 2021, 200, 117275 CrossRef CAS.
- R. C. Scholes, C. Prasse and D. L. Sedlak, The role of reactive nitrogen species in sensitized photolysis of wastewater-derived trace organic contaminants, Environ. Sci. Technol., 2019, 53(11), 6483–6491 CrossRef CAS.
- V. Burkina, V. Zlabek and G. Zamaratskaia, Effects of pharmaceuticals present in aquatic environment on phase I metabolism in fish, Environ. Toxicol. Pharmacol., 2015, 40(2), 430–444 CrossRef CAS.
- H. Pang, Q. Zhang, X. Lu, K. Li, H. Chen, J. Chen, X. Yang, Y. Ma, J. Ma and C. Huang, Nitrite-mediated photooxidation of vanillin in the atmospheric aqueous phase, Environ. Sci. Technol., 2019, 3(24), 14253–14263 CrossRef.
- G. Knopp, C. Prasse, T. A. Ternes and P. Cornel, Elimination of micropollutants and transformation products from wastewater treatment plant effluent through pilot scale ozonation followed by various activated carbon and biological filters, Water Res., 2016, 100, 580–592 CrossRef CAS.
- B. Barletta, E. Bolzacchini, S. Meinardi, M. Orlandi and B. Rindone, The NO3 radical-mediated liquid phase nitration of phenols with nitrogen dioxide, Environ. Sci. Technol., 2000, 34, 2224–3223 CrossRef CAS.
- P. Barzaghi and H. Herrmann, A mechanistic study of the oxidation of phenol by OH/NO2/NO3 in aqueous solution, Phys. Chem. Chem. Phys., 2002, 4, 3669–3675 RSC.
- A. Bedini, V. Maurino, C. Minero and D. Vione, Theoretical and experimental evidence of the photonitration pathway of phenol and 4-chlorophenol: a mechanistic study of environmental significance, Photochem. Photobiol. Sci., 2012, 11, 418–424 CrossRef CAS.
- Y. Ji, L. Wang, M. Jiang, J. Lu, C. Ferronato and J. M. Chovelon, The role of nitrite in sulfate radical-based degradation of phenolic compounds: an unexpected nitration process relevant to groundwater remediation by in-situ chemical oxidation (ISCO), Water Res., 2017, 123, 249–257 CrossRef CAS.
- Y. Ji, Y. Yang, L. Wang, J. Lu, C. Ferronato and J. M. Chovelon, Sulfate radical-induced incorporation of NO2 group into chlorophenols, Environ. Chem. Lett., 2019, 17, 1111–1116 CrossRef CAS.
- S. Rayne, K. Forest and K. J. Friesen, Mechanistic aspects regarding the direct aqueous environmental photochemistry of phenol and its simple halogenated derivatives, a review, Environ. Int., 2009, 35(2), 425–437 CrossRef CAS.
- Y. Ji, Y. Yang, L. Zhou, L. Wang, J. Lu, C. Ferronato and J. M. Chovelon, Photodegradation of sulfasalazine and its human metabolites in water by UV and UV/peroxydisulfate processes, Water Res., 2018, 133, 299–309 CrossRef CAS.
- H. Xu, Y. Li, J. Lu, J. Lu, L. Zhou, J. M. Chovenlon and Y. Ji, Aqueous photodecomposition of the emerging brominated flame retardant tetrabromobisphenol S (TBBPS), Environ. Pollut., 2021, 271, 116406 CrossRef CAS.
- C. Zeng, Y. Ji, L. Zhou, Y. Zhang and X. Yang, The role of dissolved organic matters in the aquatic photodegradation of atenolol, J. Hazard. Mater., 2012, 239–240, 340–347 CrossRef CAS.
- I. P. Pozdnyakov, X. Zhang, T. A. Maksimova, V. V. Yanshole, F. Wu, V. P. Grivin and V. F. Plyusnin, Wavelength-dependent photochemistry of acetaminophen in aqueous solutions, J. Photochem. Photobiol., A, 2014, 274, 117–123 CrossRef CAS.
- B. M. Baughman, E. Stennett, R. E. Lipner, A. C. Rudawsky and S. J. Schmidtke, Structural and spectroscopic studies of the photophysical properties of benzophenone derivatives, J. Phys. Chem. A, 2009, 113, 8011–8019 CrossRef CAS.
- L. G. Dodson, R. A. Vogt, J. Marks, C. Reichardt and C. E. Crespo-Hernandez, Photophysical and photochemical properties of the pharmaceutical compound salbutamol in aqueous solutions, Chemosphere, 2011, 83, 1513–1523 CrossRef CAS.
-
N. J. Turro, V. Ramamurthy and J. C. Scaiano, Modern Molecular Photochemistry of Organic Molecules, University Science Books, 2010 Search PubMed.
- B. A. Wols and C. H. M. Hofman-Caris, Review of photochemical reaction constants of organic micropollutants required for UV advanced oxidation processes in water, Water Res., 2012, 46, 2815–2827 CrossRef CAS.
- K. Kawabata, K. Sugihara, S. Sanoh, S. Kitamura and S. Ohta, Ultraviolet-photoproduct of acetaminophen: structure determination and evaluation of ecotoxicological effect, J. Photochem. Photobiol., A, 2012, 249, 29–35 CrossRef CAS.
- A. C. Bhasikuttan, A. K. Singh, D. K. Palit, A. V. Sapre and J. P. Mittal, Laser flash photolysis studies on the monohydroxy derivatives of benzophenone, J. Phys. Chem. A, 1998, 102, 3470–3480 CrossRef CAS.
- R. Kumasaka, A. Kikuchi and M. Yagi, Photoexcited states of UV absorbers, benzophenone derivatives, Photochem. Photobiol., 2014, 90(4), 727–733 CAS.
- P. Neta, R. E. Huie and A. B. Ross, Rate constants for reactions of inorganic radicals in aqueous solution, J. Phys. Chem. Ref. Data, 1988, 17(3), 1027–1284 CrossRef CAS.
- R. Xiao, L. Gao, Z. Wei, R. Spinney, S. Luo, D. Wang, D. D. Dionysiou, C.-J. Tang and W. Yang, Mechanistic insight into degradation of endocrine disrupting chemical by hydroxyl radical: an experimental and theoretical approach, Environ. Pollut., 2017, 231, 1446–1452 CrossRef CAS.
- P. Wang, L. Pu, Y. Wu, J. Deng and S. Zhou, Mechanistic insights into paracetamol transformation in UV/NH2Cl process: experimental and theoretical study, Water Res., 2021, 194, 116938 CrossRef CAS.
- M. J. Zhan, X. Yang, Q. M. Xian and L. R. Kong, Photochemical transformation of bisphenol A promoted by nitrate ions, Bull. Environ. Contam. Toxicol., 2006, 76, 105–112 CrossRef CAS.
- Y.-M. Kang, M.-K. Kim and K.-D. Zoh, Effect of nitrate, carbonate/bicarbonate, humic acid, and H2O2 on the kinetics and degradation mechanism of Bisphenol-A during UV photolysis, Chemosphere, 2018, 204, 148–155 CrossRef CAS.
- P. Barzaghi and H. Herrmann, A mechanistic study of the oxidation of phenol by OH/NO2/NO3 in aqueous solution, Phys. Chem. Chem. Phys., 2002, 4, 3669–3675 RSC.
- J. Dong, W. Zhao, S. Zhou, C. Zhang and D. Fu, Transformation of bisphenol A by electrochemical oxidation in the presence of nitrite and formation of nitrated aromatic by-products, Chemosphere, 2019, 236, 124835 CrossRef CAS.
- E. Moctezuma, E. Leyva, C. A. Aguilar, R. A. Luna and C. Montalvo, Photocatalytic degradation of paracetamol: intermediates and total reaction mechanism, J. Hazard. Mater., 2012, 243, 130–138 CrossRef CAS.
- R. Andreozzi, V. Caprio, R. Marotta and D. Vogna, Paracetamol oxidation from aqueous solutions by means of ozonation and H2O2/UV system, Water Res., 2003, 37, 993–1004 CrossRef CAS.
- J. Lu, J. Shao, H. Liu, Z. Wang and Q. Huang, Formation of halogenated polyaromatic compounds by laccase catalyzed transformation of halophenols, Environ. Sci. Technol., 2015, 49, 8550–8557 CrossRef CAS.
- L. Zhou, M. Sleiman, C. Ferronato, J. M. Chovelon, P. de Sainte-Claire and C. Richard, Sulfate radical induced degradation of β2-adrenoceptor agonists salbutamol and terbutaline: phenoxyl radical dependent mechanisms, Water Res., 2017, 123, 715–723 CrossRef CAS.
- L. Zhou, Q. Wang, Y. Zhang, Y. Ji and X. Yang, Aquatic photolysis of β2 agonist salbutamol: kinetics and mechanism studies, Environ. Sci. Pollut. Res., 2016, 24(6), 5544–5553 CrossRef.
- J. Ge, D. Huang, Z. Han, X. Wang, X. Wang and Z. Wang, Photochemical behavior of benzophenone sunscreens induced by nitrate in aquatic environments, Water Res., 2019, 153, 178–186 CrossRef CAS.
- H. Zúñiga-Benítez and G. A. Peñuela, Application of solar photo-Fenton for benzophenone-type UV filters removal, J. Environ. Manage., 2018, 217, 929–938 CrossRef.
- K. McNeill and S. Canonica, Triplet state dissolved organic matter in aquatic photochemistry: reaction mechanisms, substrate scope, and photophysical properties, Environ. Sci.: Processes Impacts, 2016, 18(11), 1381–1399 RSC.
- T. Zeng and W. A. Arnold, Pesticide photolysis in prairie potholes: probing photosensitized processes, Environ. Sci. Technol., 2012, 47(13), 6735–6745 CrossRef.
- J. Wang, J. Chen, X. Qiao, Y. Zhang, M. Uddin and Z. Guo, Disparate effects of DOM extracted from coastal seawaters and freshwaters on photodegradation of 2,4-Dihydroxybenzophenone, Water Res., 2019, 151, 280–287 CrossRef CAS.
- S. Canonica and U. Schönenberger, Inhibitory effect of dissolved organic matter on the transformation of selected anilines and sulfonamide antibiotics induced by the sulfate radical, Environ. Sci. Technol., 2019, 53, 11783–11791 CrossRef CAS.
- L. Lian, S. Yan, B. Yao, S.-A. Chan and W. Song, Photochemical transformation of nicotine in wastewater effluent, Environ. Sci. Technol., 2017, 51, 11718–11730 CrossRef CAS.
Footnote |
† Electronic supplementary information (ESI) available. See DOI: 10.1039/d1em00381j |
|
This journal is © The Royal Society of Chemistry 2022 |
Click here to see how this site uses Cookies. View our privacy policy here.