Occurrence and stability of ptesculentoside, caudatoside and ptaquiloside in surface waters†
Received
30th August 2021
, Accepted 23rd December 2021
First published on 17th January 2022
Abstract
The illudane glycosides ptesculentoside (PTE), caudatoside (CAU) and ptaquiloside (PTA) are found in bracken ferns (Pteridium sp.). PTA is known to contaminate water bodies adjacent to bracken ferns and hence contribute to water toxicity. This study for the first time reports the presence of PTE and CAU in surface waters with concentrations up to 5.3 μg L−1 and outlines their stability under semi-natural conditions using water of two diverse lakes at their natural pH or pH adjusted to 6.5, with temperature controlled at 5 or 15 °C, and in the presence or absence of microbial activity. Under the same set of tested conditions the three illudane glycosides degraded at similar rates: with half-lives of approximately two days at pH 7.4 and 15 °C, and approximately 12 days at pH 5.2–6.5 and 5 °C. The water origin had significant influence on the degradation rates, but only due to its difference in pH. In most cases, the degradation rates of all the three illudane glycosides could be predicted using the existing first-order model for PTA hydrolysis. As PTE and CAU exhibit the same leaching pattern and stability as PTA, previous predictions of bracken environmental impact are likely underestimated, as PTE and CAU have not been monitored and included in the risk assessment.
Environmental significance
Plant toxins are found in many natural waters as aquatic micropollutants. Illudane glycosides are a group of fern derived carcinogens which are released to surface and ground waters during precipitation events. Ptaquiloside is a well-known example. In this work we have demonstrated that other illudane glycosides exhibit similar occurrence and stability in water recipients to ptaquiloside. Our results also indicate that the extent of water contamination with illudane glycosides in fern infested areas can be several times higher than previously considered when only ptaquiloside was measured. Thus, also human exposure to bracken toxins is higher than hitherto anticipated.
|
1. Introduction
Bracken (generic name Pteridium, family Dennstaedtiaceae) is the fifth most abundant plant genus in the world,1 and its carcinogenicity is usually related to the illudane glycoside ptaquiloside (PTA).2–5 An electrophilic cyclopropyl group in the conjugated dienone reacts with nucleophiles such as water, alkaloids, amines and DNA6,7 (Fig. 1A), which by alkylating DNA causes mutations.4,8–11 Furthermore, bracken-based food is linked with human upper gastrointestinal tract cancers.12–14 Long-term residence in areas with dense bracken growth also correlates with an increased risk of gastric cancer, even if the plant is not consumed.9,15 It has been suggested that humans are exposed to PTA via inhalation of airborne spores,16 ingestion of farm animal products (dairy, meat)17–21 and drinking water from bracken-infested locations.22–25
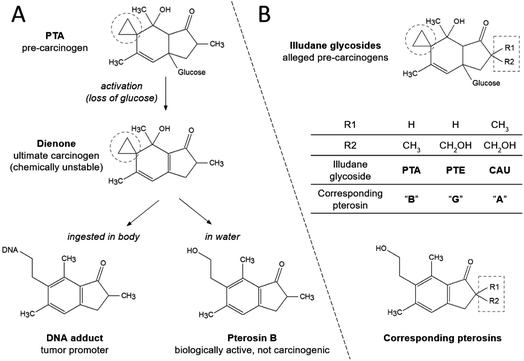 |
| Fig. 1 Hydrolysis of PTA in aqueous solution (A) and the structures of illudane glycosides and corresponding pterosins (B). The electrophilic cyclopropyl group is encircled. | |
Increased human carcinogenicity in bracken-infested regions may also be related to the plant leachates that seep into drinking water sources.22,23,26 Water containing PTA can cause in vivo gastric carcinogenesis in mice and in vitro DNA damage in human gastric epithelial cells.4,27 PTA is a glycoside and, thus, a very polar compound. It can be washed off from plants by precipitation and transported by water through soil to receiving water bodies.22,28–30 As a result, PTA has been detected in groundwater located near bracken stands in Denmark (up to 1270 ng L−1),25,26 in drinking water wells in Sweden (up to 270 ng L−1),25 at surface water abstraction sites for drinking water production in Ireland (up to 670 ng L−1)23 and in a stream leading to an active drinking water reservoir in the United Kingdom (up to 2200 ng L−1).22 The maximum tolerable concentration of PTA in drinking water is estimated to 2 ng L−1.24
Several compounds with structures nearly identical to PTA and including a highly electrophilic cyclopropyl group responsible for DNA alkylation and genetic mutations (Fig. 1) have been elucidated in Pteridaceae and Dennstaedtiaceae, e.g., hypolosides A, B and C in Hypolepis punctata and Dennstaedtia hirsta.31 In Pteridium illudane glycosides with cyclopropyl functional group mainly comprise PTA, ptesculentoside (PTE) and caudatoside (CAU).32–34 Empirical evidence suggests that PTE and CAU have the potential to cause the same adverse symptoms to cattle as PTA.34,35
Recent reports revealed a significant content of PTE and CAU, in addition to PTA, in bracken ferns.34,36,37 Specimens of various Pteridium species from six continents displayed an average distribution among PTE, CAU and PTA of 22%, 37% and 41%, respectively (0.01–5.3 mg g−1 of the three Illudane glycosides in dry weight of the plants).36 In Pteridium aquilinum and Pteridium aquilinum var. latiusculum (Desv.) from a 1500 km geographic gradient in northern Europe, the corresponding distribution of PTE, CAU and PTA was 2%, 31% and 67% (0–13.7 mg g−1 dry weight),37 and hence with a stronger dominance of PTA compared with the global average values. Other studies have found similar distribution with a notable exception to Australia, where PTE comprised 40–73% of the three illudane glycosides in Pteridium esculentum (hence the origin of the term ptesculentoside).32,34,36
The total content of PTA in mature bracken stands is estimated to fall in the range 0.1–16 kg PTA per Ha.29,30,38 The above-mentioned studies of content of PTE and CAU imply that the total capacity of reactive illudane glycosides in bracken stands may be double that of PTA. Moreover, PTE and CAU may have more significant implications on nearby water quality as they are slightly more polar than PTA.34,36 A recent PTA survey of water wells included PTE and CAU and detected up to 750 ng L−1 of CAU in drinking water wells located in bracken-dominated regions in Sweden.25 To our knowledge, no other studies have examined other illudane glycosides besides PTA as environmental contaminants.
In water, PTA hydrolyses into the less toxic non-carcinogenic product pterosin B (Fig. 1A), and rapid first-order hydrolysis takes place in both basic and strongly acidic conditions. However, PTA is stable under neutral to moderately acidic conditions and at low temperatures.6,8,28,39–41 The rate of PTA hydrolysis can be described by the following equation based on data from lab studies in buffered aqueous solutions:39
| kobs = kA[H+] + kN + kBkW[H+]−1. | (1) |
where
kobs is the observed PTA hydrolysis first-order rate constant at 22 °C;
kA = 25.7 h
−1 mol
−1;
kN = 9.49 × 10
−4 h
−1;
kB = 4.83 × 10
−4 h
−1 mol
−1;
kW = 10
−14.1. The activation energy of the reaction is 74.4 (±2.6) kJ mol
−1 determined at pH 4.5.
39
Use of the laboratory-based rate equation (eqn (1)) at pH 7 results in half-lives of PTA of 12 days at 15 °C, and 40 days at 5 °C. At pH 5, the half-lives reach 50 days at 15 °C and 150 days at 5 °C. Hence, PTA endurance in freshwater resources is facilitated by cold weather, as well as slightly acidic pH. In natural waters and soils, PTA degradation can be accelerated by microbial activity and decelerated by organic matter content.28,41,42 Also, the presence of clay silicates may slow down PTA degradation, possibly through sorption.28 Therefore, factors not included in eqn (1) may increase or decrease the rate of PTA degradation in natural waters compared with predictions made by the equation. Due to the very similar molecular structures and absence of functional groups that could majorly influence formation of different intermediates or the final products, the three illudane glycosides (Fig. 1B) may express similar aquatic stability.
This study aimed to demonstrate presence of PTE, CAU and PTA in surface waters and outline their stability in near-natural conditions. We hypothesise that the compounds can be found near bracken ferns, and that they express similar aquatic stability which can be explained through the existing model for PTA hydrolysis. These hypotheses were tested by (1) measuring the content of PTE, CAU, PTA and associated pterosins at 16 locations in Denmark, Sweden and Spain, and by (2) comparing the theoretical PTA degradation rate constants with the observed PTE, CAU and PTA degradation in two distinct types of lakes in Denmark by varying pH, temperature and microbial activity.
2. Materials and methods
2.1. Solvents and chemicals
The acids, bases and buffers (formic, hydrochloric, acetic and trifluoroacetic acids, sodium hydroxide, ammonium acetate) were of an analytical grade from Sigma-Aldrich (Denmark). The polyamide for column chromatography was from Fluka Analytical, Sigma-Aldrich Co (Germany). LC-MS grade acetonitrile was from Merck Millipore (Germany), and LC-MS grade methanol was from Honeywell (Germany). The LC-MS grade water (electrical conductivity <0.05 μS cm−1, TOC < 2 μg L−1) was prepared by the Sartorius Arium Pro Ultrapure dispenser (Germany). The standards of illudane glycosides and pterosins were prepared from powder of furled Pteridium esculentum fronds from Bribie Island, Australia, containing 2400, 220 and 800 μg g−1 of PTE, CAU and PTA, respectively, provided by Prof Mary T. Fletcher (The University of Queensland).36
2.2. Analytical method
Reference aqueous standards of PTE, CAU, PTA and the corresponding pterosins G, A and B (Fig. 1) (hereafter referred to as PtrG, PtrA and PtrB, respectively) were extracted, isolated and purified from bracken ferns and analysed in samples using an Agilent 1260 Infinity HPLC System equipped with Agilent 6130 Single Quadrupole mass spectrometer according to the method described in Kisielius et al.36 The instrumental limits of detection (LOD) regarding PTE, CAU, PTA, PtrG, PtrA and PtrB were 80, 260, 220, 10, 20 and 30 ng L−1, respectively; instrumental limits of quantification (LOQ) were 250, 780, 680, 30, 50 and 90 ng L−1, respectively.36
2.3. Mass spectral data
The MS2 data for the precursor ion of each of the six compounds (Table 1) were recorded at different collision energies43 and uploaded to the European MassBank database allowing free data access and exchange.
Table 1 Recovery of the illudane glucosides and the corresponding pterosins at 100 times preconcentration of aqueous compound solutions (1 × 10−3 ng mL−1) by SPE, followed by evaporation. The compounds are listed in order of decreasing polarity,36 the recovery percentages are determined from the averages of duplicate measurements
Compound (Fig. 1) |
Recovery (%) |
Standard deviation |
PTE |
74.2 |
3.3 |
CAU |
91.2 |
3.8 |
PtrG |
91.8 |
5.1 |
PTA |
99.2 |
1.8 |
PtrA |
96.2 |
1.4 |
PtrB |
71.5 |
1.9 |
2.4. Illudane glycoside survey of surface waters
2.4.1. Sampling.
Sixteen surface water locations (lakes, rivers, ditches) representing a broad geographical span were sampled at least twice during the bracken growth season, 2019 (Table S1 of the ESI†). Triplicate samples were obtained by drawing water from 1 cm depth at approx. 0.5 m distance from the shore into a 50 mL sterile syringe. Next, the samples were filtered through a 0.45 μm pore size sterile cellulose acetate filter (Q-Max Frisenette Syringe Filters) into a sterile conical 50 mL polypropylene centrifuge tube (Thermo Fisher Scientific, Korea). The samples were buffered by adding 1 mL of 0.3 M ammonium acetate adjusted with glacial acetic acid to pH 5, which is optimal for stabilising PTA against hydrolysis.22 Finally, the samples were immediately placed on ice and frozen to −20 °C within eight hours. The frozen samples from Spain were sent to Denmark for analysis by the University of Oviedo. The illudane glycoside and pterosin concentrations were measured in triplicate samples and determined as average. If a compound was quantified in only two of the triplicate samples, the concentrations were noted as the average of the two positive samples. If a compound was quantified in only one of the triplicate samples, the concentration was not quantified but registered as a trace.
2.4.2 Solid-phase extraction.
To make the analytical method applicable for low concentrations of the compounds in water, it was supplemented by the following 100-fold preconcentration method. The solid-phase extraction (SPE) cartridges OASIS MAX 20 cc Vac RC 60 mg (Waters co, Ireland) were successively conditioned by 2 mL of MeOH and 2 mL of DI water and then loaded with 20 mL of the water sample containing the analytes, washed with 2 mL DI water and run dry for 20 seconds. The compounds were eluted into 2 mL volume analytical HPLC autosampler vials in two stages: first stage 0.75 mL and second stage 0.5 mL MeOH, allowing the cartridges to run dry for 20 seconds after each stage. The approx. speed of conditioning, loading, washing and elution was 3 mL min−1. The collected eluate was dried with a gentle airflow in a 30 °C heating block (Mikrolab Aarhus Supertherm) and redissolved with 0.2 mL mixture of 40% (v/v) MeOH and 0.1 M ammonium acetate adjusted to pH 5.0 using glacial acetic acid.
The combined SPE and evaporation method was validated using 20 mL of the DI water samples spiked with 0.2 mL of 0.1 ng mL−1 of the compounds. The water containing each single compound was processed in separate SPE cartridges in duplicates and analysed by the LC-MS. The rates of the compound recoveries are provided in Table 1. Recovery of the most polar compound, PTE, could be increased by lowering the volumes of loading and/or DI water washing, whereas increasing the elution volume may increase the recovery of the least polar PtrB.
2.5. Stability of illudane glycosides
2.5.1. Collection of natural water free of illudane glycosides.
A spiking experiment to determine the compounds' stability required the matrix of natural water with no original content of illudane glycosides. Two lakes were selected in areas with no adjacent bracken ferns in Eastern Denmark: dissolved natural organic matter rich, acidic water of Bøllemosen (WGS84: 55.8269, 12.5639, hereafter called Blm) and clear weak alkaline water of Skodsborg Dam (WGS84: 55.8177, 12.5659, hereafter called Skd). The water samples were collected in 1 L polypropylene bottles on 10 december 2019 at approx. 2 m distance from the shore in approx. 50 cm depth. Prior to use, the bottles were washed with 10% HCl, rinsed five times with DI water and one time with water from the respective lake. The numbers of colony-forming units in the water samples were estimated according to the Danish standards concerning water survey, sampling, transport and storage for microbiological studies.44 Selected water properties are given in Table 2.
Table 2 Physicochemical parameters for the lake waters used in the spiking experiments (lake Bøllemosen (Blm) and Skodsborg Dam (Skd))
Parameter |
Measured values |
Blm |
Skd |
Applied equipment: VWR pHenomenal MU 6100H.
HACH TU5200 laser meter.
Shimadzu TOC-VCPN.
VWR pHenomenal MU 6100H, electrode VWR CO 11.
|
pH in fresh samplea |
5.2 |
7.4 |
Turbidity (FNU)b |
2.6 |
0.3 |
Non-purgeable organic carbon (NPOC) (mg l−1)c |
38.1 |
13.3 |
Electric conductivity (μS cm−1)d |
65 |
210 |
Colony forming units (CFU mL−1)44 |
64 |
230 |
2.5.2. Experimental design.
The stability of the illudane glycosides in water was tested in a full factorial experimental design based on four selected physicochemical and microbiological water parameters: water origin, temperature, pH, and sterilisation (Table 3). The design comprised all possible 16 (24) combinations of two discrete values for each parameter. The selected experimental design allowed a complete study of the effects of the interactions between the parameters.
Table 3 Values of parameters tested in a full factorial experimental design to determine the illudane glycoside degradation rates
Parameter |
Values |
Water origin |
Blm/Skd |
Temperature |
5 °C/15 °C |
pH |
Controlled pH 6.5/natural pH |
Sterilisation |
Sterilised/not sterilised |
The waters Blm and Skd (Table 3) were kept at 5 and 15 °C in ventilated incubators (Sanyo MIR-253). The pH values were kept at either natural pH or adjusted to approx. pH 6.5 using 1 M HCl or 1 M NaOH. The water was kept as sampled or sterilised through a 0.2 μm pore size sterile cellulose acetate filter (Q-Max Frisenette Syringe Filters). Once prepared, the water was distributed into 95 mL portions in loosely closed 100 mL Schott Duran laboratory glass bottles. The bottles were incubated in aerobic conditions for a six-day equilibration period prior to spiking with PTE, CAU and PTA. The pH values in the bottles were subsequently measured at 23, 72 and 504 hours after spiking the compounds (MeterLab PHM220).
2.5.3. Spiking with PTE, CAU and PTA.
An aqueous extract containing the three illudane glycosides was prepared by shaking 3.2 g of Pteridium esculentum frond powder in 160 mL of DI water in four conical 50 mL polypropylene centrifuge tubes (Thermo Fisher Scientific, Korea) at 75 rpm for 20 min (ELMI Intelli-Mixer RM-2L), centrifuging at 2500g at 1 °C for 20 min (Sigma Zentrifugen 4 K15) and filtering the supernatant through a 2 μm pore size filter paper (Whatman no. 41, Sigma-Aldrich, Germany) under vacuum.36
The filtrate was cleansed from pterosins and hydrophobic impurities when passed through a polyamide, which was packed into two cylindrical glass funnels (ϕ = 1.5 cm; l = 20 cm, BIO-RAD, Econo-Column, USA) (approx. 8 g of polyamide per funnel), at an approx. speed of 3 mL min−1. An extra 8 mL of DI water was added to each funnel to elute the residual illudane glycosides.38 The flasks were kept on ice to prevent thermal degradation. The bottles were spiked with 5 mL of the pooled obtained aqueous plant extract and vigorously shaken, resulting in initial glycoside concentration in each bottle of 2.40 mg L−1 PTE, 0.22 mg L−1 CAU, and 0.80 mg L−1 PTA.
2.5.4. Monitoring of illudane glycoside degradation.
The decrease of concentrations of the analytes in the bottles were monitored by sampling 0.2 mL water immediately after spiking (initial concentration) and after 0.75, 2, 4, 6, 23, 72, 96 and 504 hours. The samples were transferred to HPLC autosampler vials and stabilised against microbial degradation and hydrolysis by the following procedure: 0.2 mL mixture of 40% (v/v) MeOH and 0.1 M ammonium acetate adjusted to pH 5 with glacial acetic acid was added to the samples,22 which were in turn filtered through 0.2 μm pore size membranes integrated into the vial caps (Syringeless filter device Mini-Uniprep, GE Healthcare Life Sciences, UK) and immediately frozen at −20 °C.36 The vials were kept frozen until the 21st day of the experiment and thawed immediately prior to launching the LCMS analysis keeping the vials in a 4 °C autosampler. All samples were duplicated. The bottles were manually shaken before each sampling but kept loosely closed in the incubators to maintain aerobic conditions.
2.5.5. Determination of illudane glycoside degradation rates.
The concentrations of PTE, CAU and PTA in the samples at time t were expressed in percentage of their initial concentrations. Illudane glycoside degradation rates (−k) were defined by the best fitting regression model (Microsoft Excel 2016) (Fig. 2). The average illudane glycoside degradation rates were calculated by eqn (2) with n = 24 (the average degradation rates of the 3 illudane glycosides in 8 tests representing the same value of one parameter (Table 3)). | 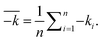 | (2) |
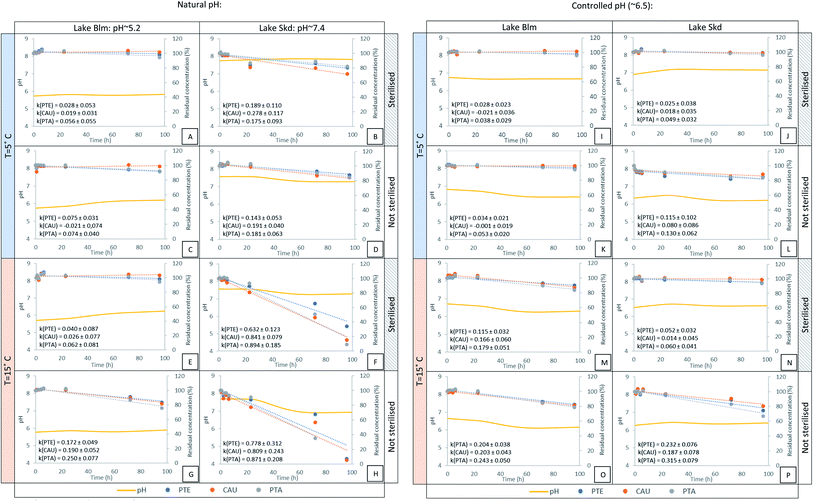 |
| Fig. 2 Measured pH (smoothed curves) and degradation rates k (±95% confidence limits) of the three illudane glycosides in two surface waters with and without pH control, at two different temperatures and with/without sterilization. Zero-order fittings are shown as punctured straight lines (eqn (3)). | |
The average illudane glycoside degradation rates between tests, which represented the opposite values of one parameter (Table 3) were compared in pairwise t-tests with 95% confidence limits, e.g., between the average rates in sterile and the average rates in non-sterile conditions. Comparison of the average degradation rates between individual illudane glycosides was made by setting the null hypothesis stating that the rates were equal and testing it in a One-Way Analysis of Variance (statistical model ANOVA, Microsoft Excel 2016). Observed PTA stability was also compared to its stability in pure aqueous buffered solutions predicted by eqn (1). The predicted PTA rate constants at different temperatures than the temperature in the model were calculated by use of the Arrhenius equation.39
3. Results and discussion
3.1. The presence of illudane glycosides in surface water
The ranges of the average concentrations of the compounds measured in surface water are provided in Table 4. The highest measured PTA concentration was two orders of magnitude lower than the highest reported in surface water in a previous study (2200 ng L−1),22 but nevertheless, exceeding the estimated tolerable drinking water concentrations of 2 ng L−1.24 The highest reported PTE concentrations were measured in waters sampled very adjacent to bracken ferns (Table S1 of the ESI†) that presumably had high PTE contents. The highest PtrG concentrations occurred in the samples with the highest PTE concentrations (Table S1 of the ESI†). This can be explained by incomplete hydrolysis of PTE resulting in its presence together with PtrG (Fig. 1). Samples with detected pterosins but without the corresponding illudane glycosides indicate a previous presence of these compounds.
Table 4 Measured concentrations of illudane glycosides and corresponding pterosins. Data of each sample, together with a description of water bodies and their distance to bracken, is provided in Table S1 of the ESI
Location |
Number of samples (n = 3)/sampling sites |
Number of positive samples |
Range of analytes in positive samples (ng L−1) |
PTE |
PtrG |
CAU |
PtrA |
PTA |
PtrB |
n.d. stands for no detection (<LOD of the total analytical method).
|
Denmark, Humleore |
34/4 |
7 |
n.d.a |
13.7 |
Trace |
Trace-3.4 |
5.9–21.2 |
n.d. |
Denmark, Grib Skov |
2/1 |
2 |
n.d. |
n.d. |
n.d. |
Trace-2.1 |
n.d. |
n.d. |
Denmark, Helsingør |
2/1 |
0 |
n.d. |
n.d. |
n.d. |
n.d. |
n.d. |
n.d. |
Sweden, Åsljunga |
4/2 |
1 |
n.d. |
n.d. |
n.d. |
Trace |
n.d. |
n.d. |
Spain, Asturias |
9/8 |
8 |
400–5300 |
3–2300 |
6.5 |
1.3–7.3 |
n.d. |
n.d. |
The measurements align with the recent report on illudane glycosides in groundwater, which showed that concentrations are highly variable, and that other illudane glycoside than PTA may dominate.25 While none of the sampled water reported in Table 4 was exploited for drinking water production, the surface water with previously unreported high concentrations of PTE (drainage ditches and pits in the Asturias region in Spain) have occasionally been consumed by horses and cattle. Assuming that the PTA safety thresholds apply to PTE, the highest reported PTE concentration would exceed the safety limit by 2600 times. These indications that PTE and CAU occur as significant surface water contaminants led to further aquatic stability studies of the compounds.
3.2. Effect of pH, temperature, and microbial activity on the stability of PTE, CAU and PTA
3.2.1. Compound stability over 96 hours.
The added PTE, CAU and PTA were fully degraded in the lake waters of Blm and Skd before the end of the experiment (on the 21st day) in 10 out of 16 tests. The last timepoint where the three compounds were detected in all 16 tests was at 96 h, except PTA in the non-sterilised lake Skd water with natural pH at 15 °C, which degraded before 96 h (Graph H, Fig. 2). The change in illudane glycoside concentrations versus time for each combination of parameters over the period of 0 to 96 h did not deviate from a linear model. Hence, a zero-order equation (eqn (3)) was used to fit the results where “A” equals the average residual concentration of the analyte in duplicate samples, and “−k” (Δ% per hour) is a zero-order rate constant (and in this case also the rate) derived from plots A–P (Fig. 2). The parallel lines (all graphs, Fig. 2) demonstrate that the degradation rates for the three compounds were similar under the same set of tested conditions. |  | (3) |
For the non-manipulated water (non-sterilised, non-changed pH), the illudane glycosides in Blm and Skd degraded at significantly different rates (graphs G and H, Fig. 2). Approx. 80% of the added glycosides remained in the water from Blm after 96 h, whereas half of the compounds had degraded after approx. 48 h in the water from Skd. These results demonstrate that degradation of the illudane glycosides strongly depend on the water composition. In pairwise tests with the same water and conditions as in graphs G and H but controlled pH (pH ∼6.5) (graphs O and P, Fig. 2), the compounds degraded at similar rates, and approx. 80% of the added compounds remained after 96 hours. This shows that pH is the main factor controlling degradation and that the relatively high natural pH caused the rapid degradation rates in Skd (Fig. 2).
The pairwise t-test of how the individual parameters affected the degradation rates are listed in Table 5.
Table 5 Average zero-order rate constants for degradation of illudane glycosides as function of reaction conditions and test of statistical significancea
Parameter |
Value |
Average k over 96 h (Δ% per h) |
95% confidence limits (Δ% per h) |
Pairwise t-tests |
The average rates (k) are calculated by eqn (2) from rate constants listed in Fig. 2.
|
Sterilization |
Sterilised |
0.164 |
±0.108 |
p = 0.001
|
Not sterilised |
0.229 |
±0.103 |
Temperature |
5 °C |
0.079 |
±0.034 |
p < 0.001
|
15 °C |
0.314 |
±0.128 |
pH |
Blm natural pH (pH 5.2) |
0.078 |
±0.053 |
p < 0.001
|
Skd natural pH (pH 7.4) |
0.499 |
±0.208 |
Water origin |
Blm with controlled pH (6.5) |
0.103 |
±0.058 |
p = 0.895 |
Skd with controlled pH (6.5) |
0.106 |
±0.060 |
Illudane glycosides |
PTE (average) |
0.179 |
±0.116 |
|
CAU (average) |
0.187 |
±0.140 |
|
PTA (average) |
0.231 |
±0.151 |
|
The null hypothesis that the average degradation rates between the compounds were the same |
p = 0.009
|
|
PTE against CAU (averages) |
p = 0.783 |
PTA against PTE (averages) |
p = 0.001
|
PTA against CAU (averages) |
p = 0.004
|
The three studied parameters showed statistically significant effects on the illudane glycoside degradation rates (p < 0.05). Temperature and pH had the highest impact on the rates, followed by sterilisation (Table 5). The combination of other water physicochemical parameters represented by distinct dissolved organic carbon content, turbidity and electric conductivity (Table 2) did not affect the illudane glycoside degradation rates (for different water origin with controlled pH: p = 0.895 ≫ 0.05).
The average degradation rates of the illudane glycosides had large confidence limits (Table 5, 95% confidence limits). The null hypothesis stating that the degradation rates of the three compounds throughout the 16 tests were identical was rejected by p < 0.05, implying a statistically significant difference between the degradation rates of the illudane glycosides. No statistically significant difference was found between the average rates of PTE and CAU (p = 0.783 > 0.05), but during the 96 h period, PTA degraded statistically different from the two other compounds (p < 0.05). Comparison of the average k of PTA (0.231) with the combined average k of PTE and CAU (0.179 and 0.187) (Table 5) shows that on average PTA degraded only approximately 20% faster than PTE and CAU.
An overview of the reaction rates as determined by interactions between reaction conditions are shown in pairwise correlation plots in Fig. 3. Sterilisation reduced the degradation rates by the same degree, regardless of the water origin (parallel lines, plot A). The change in temperature had stronger effect for non-sterilised than sterilised waters (plot B). This may be explained as temperature does not only affect the chemical hydrolysis rates,39 but also the microbial activity. The parameters compared in the plots C to E present similar co-dependencies like in Plot B. The markedly different rates between water origins vanished when water pH was adjusted to the same value (plot F). Since the water origin represents lakes with distinct physicochemical parameters (Table 2), plot F demonstrates that pH is the only parameter determining the difference in degradation rates between the two waters studied. In summary, the same degradation rates should therefore apply for surface waters of variable origin and physicochemical parameters, but with the same pH.
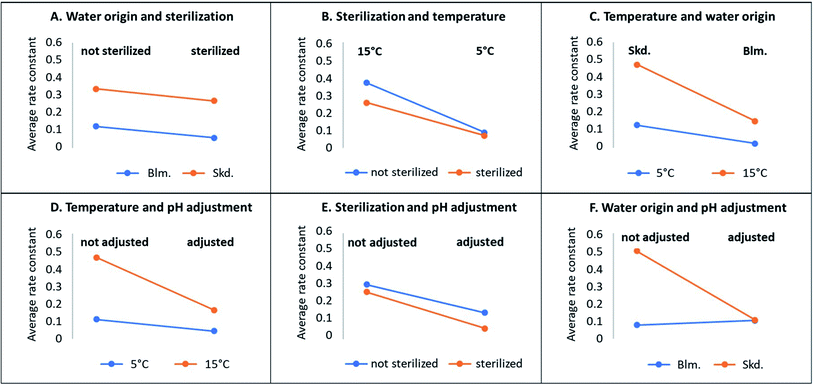 |
| Fig. 3 Sensitivity of the zero-order rate constants (eqn (2)) for illudane glycoside degradation depending on origin of water, sterilization, pH, and temperature. | |
3.2.2. Compound stability over 21 days (504 h).
After 21 days, illudane glycosides were still present in six of the tested combinations (Table 6). The most stable conditions were sterilisation, low temperature and slightly acidic to neutral pH (pH 5.2–6.5) (conditions of experiments A and I). Only low concentrations of PTE and CAU were measured for a combination of pH 5.2 and low temperature in non-sterilised water (conditions of experiment C). These observations indicate that illudane glycosides will not persist for long periods in presence of microbial activity.
Table 6 Residual concentration of illudane glycosides after 21 days (504 h) in lake water. The letter codes of the experiments correspond to Fig. 2
c
Experiment ID, pH, water origin and temperaturea |
Residual conc. (% ± 95% confidence limits) and indication of sterilisation |
PTE |
CAU |
PTA |
Bold and italic indicate 5 °C and 15 °C, respectively.
Sterilization.
A dash represents no detection.
|
A (pH ≈ 5.2) Blmb |
94.2 ± 33.3 |
95.3 ± 19.3 |
93.6 ± 56.4 |
B (pH ≈ 7.4) Skdb |
54.5 ± 9.9 |
39.8 ± 0.4 |
64.0 ± 10.7 |
C (pH ≈ 5.2) Blm |
3.0 ± 2.5 |
8.4 ± 2.0 |
— |
D (pH ≈ 7.4) Skd |
— |
— |
— |
E (pH ≈ 5.2) Blmb |
— |
— |
— |
F (pH ≈ 7.4) Skdb |
— |
— |
— |
G (pH ≈ 5.2) Blm |
— |
— |
— |
H (pH ≈ 7.4) Skd |
— |
— |
— |
I (pH ≈ 6.5) Blmb |
91.9 ± 2.9 |
93.7 ± 22.6 |
92.6 ± 13.2 |
J (pH ≈ 6.5) Skdb |
77.0 ± 20.4 |
65.8 ± 28.0 |
82.6 ± 19.9 |
K (pH ≈ 6.5) Blm |
— |
— |
— |
L (pH ≈ 6.5) Skd |
— |
— |
— |
M (pH ≈ 6.5) Blmb |
— |
— |
— |
N (pH ≈ 6.5) Skdb |
12.2 ± 2.0 |
8.1 ± 0.8 |
3.2 ± 0.8 |
O (pH ≈ 6.5) Blm |
— |
— |
— |
P (pH ≈ 6.5) Skd |
— |
— |
— |
3.3. Comparison of predicted and observed stability of PTA
No rate laws or kinetic data has been published for degradation of PTE and CAU. We have now demonstrated that the kinetics of PTE and CAU degradation follow the same pattern as for PTA. Here, we compare the observed degradation rates for the first 96 hours where monitoring was intense (Fig. 2) with the predictions of the hydrolysis kinetic rate law (eqn (1)). It is evident, that the apparent zero-order degradation we observed (eqn (3)) is a result of the short observation period as the short timeframe results in linear degradation even with eqn (1). The predictions of all the studied conditions also appear linear over the studied period and are shown in Fig. S1 of the ESI,† with one example in Fig. 4.
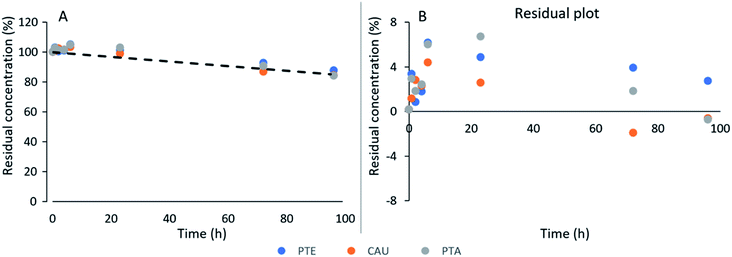 |
| Fig. 4 (A) Example of observed illudane glycoside degradation data of experiment D (Fig. 2) and predicted PTA hydrolysis at the given conditions (eqn (1), pH 7.4, T 5 °C: dashed line), and (B) residual plot of the observed concentrations versus the model. | |
To test for the general versatility of eqn (1) to describe the short term degradation kinetics of the illudane glycosides in lake waters, we have compared the predicted and the observed residual PTA concentrations at 90 hours near the end of the study period (Table 7).
Table 7 Comparison of the predicted and observed residual PTA concentrations after degradation for 90 hours. The rows representing experiments in sterilised water are indicateda
Experiment ID |
Predicted residual conc. (%) (eqn (1)) |
Observed residual conc. (%) |
Ratio of predicted and observed residual conc. |
After 30 hours |
After 90 hours (%) |
After 30 hours (%) |
After 90 hours (%) |
After 90 hours |
Rows representing experiments in sterilized water.
Significant deviations from unity.
|
A (T 5 °C, pH ≈ 5.2)a |
99 |
98 |
98 |
95 |
1.0 |
C (T 5 °C, pH ≈ 5.2) |
98 |
93 |
1.1 |
B (T 5 °C, pH ≈ 7.4)a |
95 |
86 |
95 |
84 |
1.0 |
D (T 5 °C, pH ≈ 7.4) |
95 |
84 |
1.0 |
E (T 15 °C, pH ≈ 5.2)a |
98 |
95 |
98 |
94 |
1.0 |
G (T 15 °C, pH ≈ 5.2) |
93 |
78 |
1.2 |
F (T 15 °C, pH ≈ 7.4)a |
86 |
63 |
73 |
20 |
3.2b |
H (T 15 °C, pH ≈ 7.4) |
74 |
22 |
2.9b |
I (T 5 °C, pH ≈ 6.5)a |
99 |
97 |
99 |
97 |
1.0 |
J (T 5 °C, pH ≈ 6.5)a |
99 |
96 |
1.0 |
K (T 5 °C, pH ≈ 6.5) |
98 |
95 |
1.0 |
L (T 5 °C, pH ≈ 6.5) |
96 |
88 |
1.1 |
M (T 15 °C, pH ≈ 6.5)a |
97 |
91 |
95 |
84 |
1.1 |
N (T 15 °C, pH ≈ 6.5)a |
98 |
95 |
1.0 |
O (T 15 °C, pH ≈ 6.5) |
93 |
78 |
1.2 |
P (T 15 °C, pH ≈ 6.5) |
91 |
72 |
1.3 |
Except for rapid PTA degradation in the high-temperature alkaline natural water (experiments F and H), similar residual PTA concentrations were measured as predicted by rate eqn (1). Sterilisation could not explain the overall faster degradation rates in experiments in F and H. The general applicability of eqn (1) to describe PTA hydrolysis in waters of different origin is also in line with studies of PTA hydrolysis in groundwater.42 However, in presence of solids, eqn (1) may not be valid. Hence, PTA degradation in sand filters was found to be two orders of magnitude faster than predicted by eqn (1).45 Also degradation in soils is faster than predicted for pure aqueous solutions.28,41
3.4. Formation of transformation products
During the stability experiments, pterosins G and B were monitored by LC-MS. Conditions favorable for illudane glycoside hydrolysis (high temperature, basic pH) resulted in more substantial formation of the corresponding pterosins, which is in line with previously studied formation of pterosins B and A.45,46 As a combined result of simultaneous pterosin formation and degradation, their concentrations often peaked during intermediate monitoring time intervals (Fig. S2 of the ESI†). Even though pterosins are biologically active compounds, they are not known to be carcinogenic and therefore are considered less relevant water contaminants than the illudane glycosides.47–49
In addition to pterosin formation, other transformation products were observed (Fig. S4–S6 of the ESI†). No analytical standards to validate the compounds' identity were available, but the mass spectrometric data suggest carcinogenic transformation products formed during PTA hydrolysis to PtrB (dienone) and similar for PTE (Fig. 1). The two transformation products often occurred at similar time intervals and conditions, which further confirms the same reaction pathway for the illudane glycosides (Fig. S3 of the ESI†).
4. Conclusion
For the first time we have demonstrated the presence of PTE, CAU and associated pterosins in surface waters adjacent to stands of bracken ferns. Up to 5300 ng L−1 of PTE were found. Comparison with previously reported PTA levels in surface waters (up to 2200 ng L−1) demonstrates that concentration levels of illudane glycosides other than PTA can be significant. The measured individual pterosins further confirm the findings and infer potential abundance of the parent illudane glycosides prior to hydrolysis several times higher than previously considered as only PTA and pterosin B were measured. Illudane glycosides rather than individual PTA should be referred to as water contaminants from bracken.
Illudane glycoside stability experiments showed that PTE, CAU and PTA express similar reaction mechanism and follow the same rate law. The existing PTA hydrolysis equation can be applied to predict degradation rates of the three compounds with a few exceptions. The three illudane glycosides showed comparable stability for all studied conditions. Different rates of illudane glycoside hydrolysis for the tested lake waters could be entirely ascribed to differences in water. This demonstrates that water characteristics known as risky because of high stability of PTA apply also for both PTE and CAU.
The findings also suggest formation of potentially toxic transformation products from illudane glycosides such as dienones. These compounds entail nucleophilic functional groups and would contribute to carcinogenicity of water with decaying illudane glycosides. In order to better assess water carcinogenicity in bracken infested regions, future water quality studies besides PTA and its final hydrolysis product pterosin B should include monitoring of PTE, CAU, associated pterosins and dienone series transformation products.
Funding
This project was funded by the European Union's Horizon 2020 research and innovation program under the Marie Sklodowska-Curie grant agreement no. 722493.
Author contributions
V. K. and L. H. R. conceived and directed the study, V. K., M. D. and J. K. D. performed experiments, N. S. M. developed solid-phase extraction, D. N. L., H. C. B. H. and L. H. R. supervised, V. K. wrote the manuscript with feedback from H. C. B. H. and L. H. R.
Conflicts of interest
There are no conflicts to declare.
Acknowledgements
The authors are grateful to Professor Elena María Fernández González (University of Oviedo, Spain) for the water samples from the Asturias region in Spain, and to Professor Mary T. Fletcher (University of Queensland, Australia) for providing the plant material used for the compound extraction. The authors would also like to thank Dr Werner Brack and Dr Tobias Schulze (Helmholtz Centre for Environmental Research, Germany) for preparing the high-resolution mass spectral data of the compounds for the European MassBank database. Lastly, the authors thank Anita Schjødt Sandager, Sorivan Chhem Kieth (University of Copenhagen, Denmark) and Greater Copenhagen Utility HOFOR (Denmark) for providing the water physicochemical analysis applied in the study, and Liza Rylander Krintel (University College Copenhagen, Denmark) for linguistic advice to the manuscript.
References
- J. A. Thomson, Towards a taxonomic revision of Pteridium (Dennstaedtiaceae), Telopea, 2004, 10, 793–803 Search PubMed.
- M. Matoba, E. Saito, K. Saito, K. Koyama, S. Natori, T. Matsushima and M. Takimoto, Assay of ptaquiloside, the carcinogenic principle of bracken, Pteridium aquilinum, by mutagenicity testing in Salmonella typhimurium, Mutagenesis, 1987, 2, 419–423 CrossRef CAS PubMed.
- I. Hirono, H. Ogino, M. Fujimoto, K. Yamada, Y. Yoshida, M. Ikagawa and M. Okumura, Induction of Tumors in ACI Rats Given a Diet Containing Ptaquiloside, a Bracken Carcinogen, J. Natl. Cancer Inst., 1987, 79, 1143–1149 CAS.
- J. Gomes, A. Magalhaes, V. Michel, I. F. Amado, P. Aranha, R. G. Ovesen, H. C. B. Hansen, F. Gartner, C. A. Reis and E. Touati,
Pteridium aquilinum and its ptaquiloside toxin induce DNA damage response in gastric epithelial cells, a link with gastric carcinogenes, Toxicol. Sci., 2012, 126, 60–71 CrossRef CAS PubMed.
- D. M. Potter and M. S. Baird, Carcinogenic effects of ptaquiloside in bracken fern and related compounds, Br. J. Cancer, 2000, 87, 914–920 CrossRef PubMed.
- M. Ojika, K. Wakamatsu, H. Niwa and K. Yamada, Ptaquiloside, a potent carcinogen isolated from bracken fern Pteridium aquilinum var. latiusculum: structure elucidation based on chemical and spectral evidence, and reactions with amino acids, nucleosides, and nucleotides, Tetrahedron, 1987, 43, 5261–5274 CrossRef CAS.
- T. Kushida, M. Uesugi, Y. Sugiura, H. Kigoshi, H. Tanaka, J. Hirokawa, M. Ojika and K. Yamada, DNA damage by ptaquiloside, a potent bracken carcinogen: detection of selective strand breaks and identification of DNA cleavage products, J. Am. Chem. Soc., 1994, 116, 479–486 CrossRef CAS.
- K. Yamada, M. Ojika and H. Kigoshi, Ptaquiloside, the major toxin of bracken, and related terpene glycosides: chemistry, biology and ecology, Nat. Prod. Rep., 2007, 24, 798–813 RSC.
- R. M. Gil da Costa, M. M. S. M. Bastos, P. A. Oliveira and C. Lopes, Bracken-associated human and animal health hazards: chemical, biological and pathological evidence, J. Hazard. Mater., 2012, 1, 203–204 Search PubMed.
- A. S. Prakash, T. N. Pereira, B. L. Smith, G. Shaw and A. A. Seawright, Mechanism of bracken fern carcinogenesis: evidence for H-ras activation via initial adenine alkylation by ptaquiloside, Nat. Toxins, 1996, 4, 221–227 CrossRef CAS PubMed.
- H. Kigoshi, H. Tanaka, J. Hirokawa, K. Mizuta and K. Yamada, Synthesis of analogues of a bracken ultimate carcinogen and their DNA cleaving activities, Tetrahedron Lett., 1992, 33, 6647–6650 CrossRef CAS.
- M. Shahin, B. L. Smith and A. S. Prakash, Bracken carcinogens in the human diet, Mutat. Res., 1999, 443, 69–79 CAS.
- M. E. Alonso-Amelot and M. Avendano, Human carcinogenesis and bracken fern: a review of the evidence, Curr. Med. Chem., 2002, 9, 675–686 CrossRef CAS PubMed.
- M. E. Alonso-Amelot and M. Avendano, Possible association between gastric cancer and bracken fern in Venezuela: An epidemiologic study, Int. J. Cancer, 2001, 91, 252–259 CrossRef CAS PubMed.
- O. P. Galpin, C. J. Whitaker, R. Whitaker and J. Y. Kassab, Gastric cancer in Gwynedd. Possible links with bracken, Br. J. Cancer, 1990, 61, 737–740 CrossRef CAS PubMed.
- L. H. Rasmussen, B. Schmidt and E. Sheffield, Ptaquiloside in bracken spores from Britain, Chemosphere, 2013, 90, 2539–2541 CrossRef CAS PubMed.
- A. Virgilio, A. Sinisi, V. Russo, S. Gerardo, A. Santoro, A. Galeone, O. Taglialatela-Scafati and F. Roperto, Ptaquiloside, the major carcinogen of bracken fern, in the pooled raw milk of healthy sheep and goats: an underestimated, global concern of food safety, J. Agric. Food Chem., 2015, 63, 4886–4892 CrossRef CAS PubMed.
- M. E. Alonso-Amelot, U. Castillo, B. L. Smith and D. R. Lauren, Bracken ptaquiloside in milk, Nature, 1996, 382, 587 CrossRef CAS PubMed.
- M. E. Alonso Amelot, U. Castillo, M. D. Sanchez and F. DeJongh, Detection of ptaquiloside the potent carcinogen of bracken fern Pteridium aquilinum in bovine milk, Am. Chem. Soc., 1992, 203, 1–3 Search PubMed.
- M. E. Alonso-Amelot, U. Castillo and F. De Jongh, Passage of the bracken fern carcinogen ptaquiloside into bovine milk, Lait, 1993, 73, 323–332 CrossRef CAS.
- M. T. Fletcher, K. G. Reichmann, I. J. Brock, R. A. McKenzie and B. J. Blaney, Residue Potential of Norsesquiterpene Glycosides in Tissues of Cattle Fed Austral Bracken (Pteridium esculentum), J. Agric. Food Chem., 2011, 59, 8518–8523 CrossRef CAS PubMed.
- F. Clauson-Kaas, C. Ramwell, H. C. B. Hansen and B. W. Strobel, Ptaquiloside from bracken in stream water at base flow and during storm events, Water Res., 2016, 106, 155–162 CrossRef CAS PubMed.
- C. O'Driscoll, C. Ramwell, B. Harhen, L. Morrison, F. Clauson-Kaas, H. C. B. Hansen, G. Campbell, J. Sheahan, B. Misstear and L. W. Xiao, Ptaquiloside in Irish Bracken Ferns and Receiving Waters, with Implications for Land Managers, Molecules, 2016, 21, 543 CrossRef PubMed.
-
L. H. Rasmussen, Ptaquiloside – an Environmental Hazard?, PhD thesis, The Royal Veterinary and Agricultural University, Denmark, Frederiksberg, 2003.
- N. Skrbic, V. Kisielius, A.-K. Pedersen, S. C. B. Christensen, M. J. Hedegaard, H. C. B. Hansen and L. H. Rasmussen, Occurrence of carcinogenic illudane glycosides in drinking water wells, Environ. Sci. Eur., 2021, 33, 44 CrossRef CAS.
- F. Clauson-Kaas, P. H. Jensen, O. S. Jacobsen, R. K. Juhler and H. C. B. Hansen, The naturally occurring carcinogen ptaquiloside is present in groundwater below bracken vegetation, Toxicol. Environ. Chem., 2014, 33, 1030–1034 CrossRef CAS PubMed.
- J. Gomes, A. Magalhaes, A. S. Carvalho, G. E. Hernandez, S. L. Papp, S. R. Head, V. Michel, L. David, F. Gartner, E. Touati and C. A. Reis, Glycophenotypic Alterations Induced by Pteridium aquilinum in Mice Gastric Mucosa: Synergistic Effect with Helicobacter pylori Infection, PLoS One, 2012, 7, 6 Search PubMed.
- L. H. Rasmussen, H. C. Hansen and D. Lauren, Sorption, degradation and mobility of ptaquiloside, a carcinogenic Bracken (Pteridium sp.) constituent, in the soil environment, Chemosphere, 2005, 58, 823–835 CrossRef CAS PubMed.
- L. H. Rasmussen, S. Kroghsbo, J. C. Frisvad and H. C. B. Hansen, Occurrence of the carcinogenic Bracken constituent ptaquiloside in fronds, topsoils and organic soil layers in Denmark, Chemosphere, 2003, 51, 117–127 CrossRef CAS PubMed.
- D. B. Garcia-Jorgensen, E. Diamantopoulos, V. Kisielius, M. Rosenfjeld, L. H. Rasmussen, B. W. Strobel and H. C. B. Hansen, Bracken growth, toxin production and transfer from plant to soil - A two-year monitoring study, Environ. Sci. Eur., 2021, 33, 45 CrossRef CAS.
- K. Saito, T. Nagao, S. Takatsuki, K. Koyama and S. Natori, The sesquiterpenoid carcinogen of bracken fern, and some analogues, from the Pteridaceae, Phytochemistry, 1990, 29, 1475–1479 CrossRef CAS.
- M. T. Fletcher, P. Y. Hayes, M. J. Somerville and J. J. De Voss, Ptesculentoside, a novel norsesquiterpene glucoside from the Australian bracken fern Pteridium esculentum, Tetrahedron Lett., 2010, 51, 1997–1999 CrossRef CAS.
- U. F. Castillo, A. L. Wilkins, D. R. Lauren, B. L. Smith, N. R. Towers, M. E. Alonso-Amelot and R. Jaimes-Espinoza, Isoptaquiloside and caudatoside, illudane-type sesquiterpene glucosides from Pteridium aquilinum var. caudatum, Phytochemistry, 1997, 44, 901–906 CrossRef CAS.
- M. T. Fletcher, I. J. Brock, K. G. Reichmann, R. A. McKenzie and B. J. Blaney, Norsesquiterpene Glycosides in Bracken Ferns (Pteridium esculentum and Pteridium aquilinum subsp. Wightianum) from Eastern Australia: Reassessed Poisoning Risk to Animals, J. Agric. Food Chem., 2011, 59, 5133–5138 CrossRef CAS PubMed.
- L. G. S. de Oliveira, F. M. Boabaid, V. Kisielius, L. H. Rasmussen, F. Buroni, M. Lucas, C. O. Schild, F. Lopez, M. Machado and F. Riet-Correa, Hemorrhagic diathesis in cattle due to consumption of Adiantopsis chlorophylla (Swartz) Fée (Pteridaceae), Toxicon: X, 2020, 5, 100024 CrossRef CAS PubMed.
- V. Kisielius, D. N. Lindqvist, M. B. Thygesen, M. Rodamer, H. C. B. Hansen and L. H. Rasmussen, Fast LC-MS quantification of ptesculentoside, caudatoside, ptaquiloside and corresponding pterosins in bracken ferns, J. Chromatogr. B: Biomed. Sci. Appl., 2020, 1138, 121966 CrossRef CAS PubMed.
-
V. Kisielius, Toxic secondary metabolites from plants: a new aspect of water quality, PhD thesis, University of Copenhagen, Denmark, Frederiksberg, 2020.
- L. H. Rasmussen, E. Donnelly, B. W. Strobel, P. E. Holm and H. C. B. Hansen, Land management of bracken needs to account for bracken carcinogens - A case study from Britain, J. Environ. Manage., 2015, 151, 258–266 CrossRef CAS PubMed.
- K. B. Ayala-Luis, P. B. Hansen, L. H. Rasmussen and H. C. Hansen, Kinetics of ptaquiloside hydrolysis in aqueous solution, Environ. Toxicol. Chem., 2006, 25, 2623–2629 CrossRef CAS PubMed.
- K. Saito, T. Nagao, M. Matoba, K. Koyama, S. Natori, T. Murakami and Y. SaikI, Chemical assay of ptaquiloside, the carcinogen of Pteridium aquilinum, and the distribution of related compounds in the Pteridaceae, Phytochemistry, 1989, 28, 1605–1611 CrossRef CAS.
- R. G. Ovesen, L. H. Rasmussen and H. C. B. Hansen, Degradation kinetics of ptaquiloside in soil and soil solution, Environ. Toxicol. Chem., 2008, 27, 252–259 CrossRef CAS PubMed.
- J. S. Wu, F. Clauson-Kaas, D. N. Lindqvist, L. H. Rasmussen, B. W. Strobel and H. C. B. Hansen, Does the natural carcinogen ptaquiloside degrade readily in groundwater, Environ. Sci. Eur., 2021, 33, 24 CrossRef CAS.
- MassBank Europe [Online], accessed 30 10 2021, available: https://massbank.eu/MassBank/.
-
Danish Standards Foundation, DS2250 Water quality - Sampling, transportation and storage of samples for microbiological analysis, Copenhagen, Denmark, 1983 Search PubMed.
- N. Skrbic Mrkajic, J. Hama, B. W. Strobel, H. C. B. Hansen, L. H. Rasmussen, A. K. Pedersen, S. C. B. Christensen and M. J. Hedegaard, Removal of phytotoxins in filter sand used for drinking water treatment, Water Res., 2021, 205, 117610 CrossRef PubMed.
- P. J. O'Connor, M. E. Alonso-Amelot, S. A. Roberts and A. C. Povey, The role of bracken fern illudanes in bracken fern-induced toxicities, Mutat. Res., Rev. Mutat. Res., 2019, 782, 108276 CrossRef PubMed.
- M. Saito, M. Umeda, M. Enomoto, Y. Hatanaka, S. Natori, K. Yoshihira, M. Fukuoka and M. Kuroyanagi, Cytotoxicity and carcinogenicity of pterosins and pterosides, 1-indanone derivatives from bracken (Pteridium aquilinum), Experientia, 1975, 31, 829–831 CrossRef CAS PubMed.
- F. L. Hsu, C. F. Huang, Y. W. Chen, Y. P. Yen, C. T. Wu, B. J. Uang, R. S. Yang and S. H. Liu, Antidiabetic Effects of Pterosin A, a Small-Molecular-Weight Natural Product, on Diabetic Mouse Models, Diabetes, 2013, 62, 628–638 CrossRef CAS PubMed.
- Y. Yahara, H. Takemori, M. Okada, A. Kosai, A. Yamashita, T. Kobayashi, K. Fujita, Y. Itoh, M. Nakamura, H. Fuchino, N. Kawahara, N. Fukui, A. Watanabe, T. Kimura and N. Tsumaki, Pterosin B prevents chondrocyte hypertrophy and osteoarthritis in mice by inhibiting Sik3, Nat. Commun., 2016, 7, 10959 CrossRef CAS PubMed.
Footnote |
† Electronic supplementary information (ESI) available. See DOI: 10.1039/d1em00364j |
|
This journal is © The Royal Society of Chemistry 2022 |