DOI:
10.1039/D2EA00067A
(Critical Review)
Environ. Sci.: Atmos., 2022,
2, 891-920
Snow particles physiochemistry: feedback on air quality, climate change, and human health
Received
8th June 2022
, Accepted 31st July 2022
First published on 5th August 2022
Abstract
During the last several decades, numerous researchers have provided evidence that physical and biogeochemical processes at air-snow/ice-water interfaces are very complex, and, in many cases, interlinked. This review focuses on the current state of knowledge regarding snow-borne particles. It integrates snow science from different angles: from the formation of snow and precipitation to transformations through natural and anthropogenic processes and impacts and snow management in urban areas sites. We discuss the physical, chemical, and biological characteristics of particles in snow, such as their composition, abundance, size distribution, ice nucleation properties, genomic features, and microphysical processes, in urban settings, remote areas of the Arctic, and remote industrial regions (oil sands). We explore physicochemical processes of snow particles: from microbial to emerging contaminants, like nano/microplastics, light-absorbing carbonaceous organics, halogenated and nanometals particles. We review the possible contributions of snow particles to atmospheric radiation and climate, biogeochemistry, human health, and urban snow management. We propose further research directions to improve understanding of air-snow feedback, and sustainable snow management in urban areas, in the age of emerging contaminants in a changing climate.
Environmental significance
Fresh snow is a snapshot of atmospheric processes. Airborne particles or aerosols are pivotal for heterogeneous ice nucleation, ice growth, accumulation of ice crystals, and snow precipitation. Snow can accommodate and uptake particles (natural, anthropogenic including emerging contaminants) and gaseous species, as it falls. In this paper, we explore the selected physicochemical characterization of snow-borne particles and their roles in complex snow transformations, biogeochemical processes, microphysics, and snow reflectivity or albedo. We discuss the role of snow particles on freezing–melting cycles and their impact on snow interactions with the Earth's atmosphere, lithosphere, hydrosphere, and cryosphere.
|
1. Introduction
Overview: Fresh snow is a snapshot of atmospheric processes.1 Airborne particles or aerosols are pivotal for heterogeneous ice nucleation, ice growth, accumulation of ice crystals, and snow precipitation. Airborne particles are also important factors in determining the type, intensity, and frequency of precipitation.2 Snow can accommodate and uptake particles and gaseous species, as it falls. In this paper, we explore the selected physicochemical characterization of snow-borne particles and their roles in complex snow physicochemical transformations, biogeochemical processes, microphysics, and snow reflectivity or albedo (Fig. 1). We also discuss the role of snow particles on freezing–melting cycles and their impact on snow interactions with the Earth's atmosphere, lithosphere, hydrosphere, and cryosphere.3
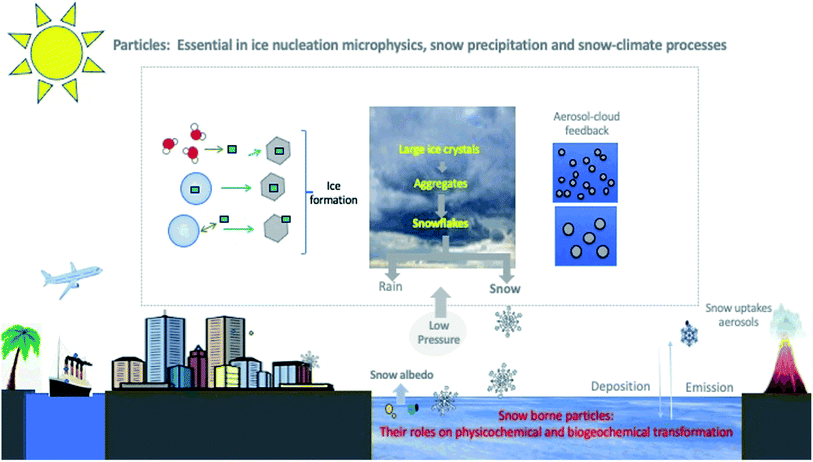 |
| Fig. 1 Simplified schematic of the role of particles in snow formation, precipitation, and physicochemical transformation in the presence of natural and anthropogenic activities. A few cartoons have been obtained from online public domains and inspired by a Meteorological textbook.7 For simplification, only one pathway represents condensation and immersion freezing nucleation processes. | |
Snow-borne particle characterization provides insights into the understanding of aerosol-cloud interactions, which is a research priority identified by the Intergovernmental Panel on Climate Change (IPCC).4 The lack of understanding of aerosols and their interactions in cloud processes has been identified as an important uncertainty in the formation of precipitation. To address this challenge, the physical and chemical properties of aerosols, such as size, composition, contact angle – the liquid-vapor interface angle with a solid surface, hygroscopicity, surface properties, and photochemistry should be understood.5 Interestingly, the health effects of aerosols are also determined by these physicochemical properties. The World Health Organization (WHO)6 has considered airborne particles, particularly nanoparticles (<100 nm diameters), as a top research priority and a significant cause of premature human death. In this paper, we also explore how snow particles originating from the atmosphere can affect climatic processes and others that have also the potential for adverse health effects.
Urban snow: The urban areas cover only about 3% of the World's land surface.8 Yet, urban atmospheric emissions are responsible for ∼50% to 80% of the total global greenhouse gas emissions9–11 and are a significant source of airborne particles that include nanoparticles and emerging contaminants. The United Nations has estimated that the majority of the world population (∼55%) live in an urban area, and this number will grow to 68% by 2050.12 As shown in Fig. 2, many cities in the world receive snow precipitation. Snow is a (photo)biogeochemical interface for uptake, transformation and emission of gases and particles.13,14 In polluted urban regions, snow has been shown to be effective for uptake of particulate matter, including airborne nanoparticles.15 Yet, after snow events and upon melting–freezing cycles, the emission of airborne nanoparticles to the atmosphere is observed.15 In urban regions, vehicle combustion particulate emission has been shown to alter snow composition.14,16 Furthermore, the usage of salt particles widely used for snow removal in wintertime, has been shown to significantly impact atmospheric compositions and pollutant fates, and thus affecting air quality.17,18
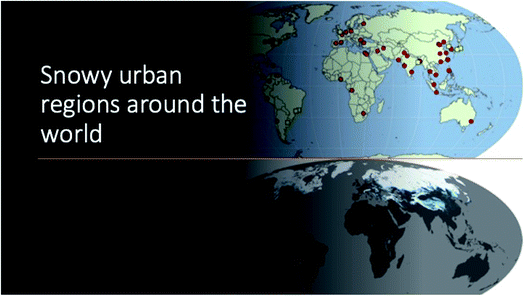 |
| Fig. 2 The top world map shows the major cities of the globe, and the bottom picture shows snowy regions in the world. Note that in the Southern Hemisphere, there are several cities in the mountainous areas which receive snow (white-blue dots). The original graphs were obtained from the public domains made by the public maps made by two organizations: United Nations (UN) and NASA. We herein modified them by giving 3-dimensional effects, and hence the original pictures are changed.19,20 | |
Particles in snow – from natural to emerging particles: Snow particles are linked to a few climate processes. Particles are known to alter snow albedo,21 and thereby reflect energy by snow surfaces. Furthermore, dust and volcanic ash can induce insulation effects on snow and ice.21 Coal mine dust particles, for instance, are suggested to accelerate Arctic snowmelt.22 Snow-borne microbes have been shown to be influential on metal cycling at snow-air interfaces.23,24 Snow particles such as black carbon and other light-absorbing particles25 affect the energy at the snow-air interface, and affect the cryosphere and melting processes,25 which will be described in detail in this paper.
Like snow and ice that are ubiquitous in the Arctic, the Antarctic, some subarctic, mid-latitudes, and mountainous regions, snow-borne particles are prevailing features of different types of snow (Fig. 2). They can reveal information about their sources, for instance we can determine whether they come from anthropogenic or natural emissions and also any atmospheric transformation processes they have undergone.26,27 Snow-borne particles vary in size, from nm to sub-millimeter, and are of diverse composition: organic, inorganic (including certain metals), and biological.27–31 Snow-borne microorganisms have been identified under harsh weather conditions.26,32,33 and have been shown to impact the degradation of organic compounds such as proteins, and carbohydrates,33,34 and nutrient cycling.35,36
The snow particle composition, number density, and distribution vary in various regions of the globe and at different times.37 As the atmosphere is the fastest moving fluid in the Earth's ecosystem, air pollutants such as aerosols can be distributed widely, affecting the snow composition locally, regionally, and globally. For instance, due to their small sizes, airborne nanoparticles are subject to long-range transport. Even in remote areas such as the Arctic regions, snow composition is changing due to long-range transport of pollutants and local industrial activities such as metal mining,38 oil exploration,39 military activities, and tourism.40 It is important to note that aerosols and snow-borne particles may contain toxic components (e.g., polycyclic aromatic hydrocarbons (PAHs) or pesticides).41–43 There is increasing evidence that a wide range of snow particles around the world contain components such as microplastics and nanometals, which are considered as emerging contaminants.43,44 Micro- and nano-plastics have been detected in snow, marine, and terrestrial ecosystems including oceans, rivers, air, drinking water, sediments, and food45–48 The major human exposure pathways to microplastics and some other emerging contaminants such as PAHs are via inhalation and ingestion.49 Snow surfaces have been shown to be efficient for uptake of airborne nano- and microparticles, transformation, and releasing them into the atmosphere.15 Characterization of the snow-borne particles can thus provide us with information that can advance our understanding of climate change, human health, and our environment.
Snow in remote industrial sites can be influenced by anthropogenic activities such as refining Canadian oil sands.50 Industrial activities generate concern due to their impacts on both human health and the environment.51,52 In oil sand fields located at northern latitudes, an elevated concentration of metals in particles has been observed in snow.53 Many of these metals are considered toxic or priority pollutants of health concern under the US Environmental Protection Agency's Clean Water Act (e.g., Cr, Ni, As, Se, Cd, and Pb).54
Goals of this review: There are several reviews on snow-gas interactions in the atmosphere, which include reactions of halogens in polar regions and biogeochemical processes.55–59 In this paper, by focusing on snow particles, we provide insights into the variability of snow-borne composition in different regions, and compare three sites in North America: (a) Arctic sites, (b) a model urban sites, and (c) a remote-industrial region (Alberta oil sands). We will focus on the importance of particles of diverse origins, from natural to emerging contaminants, in the formation of snow in the atmosphere, precipitation processes, their transformation within snow, and their potential climatic feedback and release to the Earth's ecosystem including the atmosphere and water, where they can impact ecosystem health. We also discuss newly developed analytical techniques with potential application for snow particle research, and the existence of emerging contaminants (e.g., nano- and microplastics). Besides, we explore possible health effects of particles observed in snow. In addition, we discuss the ice nucleation and radiative impacts of light-absorbing carbonaceous aerosols in cryosphere. Since there are limited studies for the snow particles in southern hemisphere,60–63 we thus focused this review predominantly in the Northern Hemisphere. Lastly, we show different snow management strategies in urban areas, while proposing future research directions.
2. Snow particle physicochemical characterization: anthropogenic vs. natural
In this section, we discuss the physicochemical characterization of snow, including size, composition, and number distribution of particles in three different climates: (1) Arctic, (2) remote industrial site, and (3) a model cold-climate urban environment. We learn how snow particles can vary in size, undergoing transformations, and undergo a cycle of melting and freezing, allowing interactions with other parts of the ecosystem, including the Earth's atmosphere.
Since fresh snow uptakes aerosols, snow particles can originate from either anthropogenic or natural sources (including biogenic sources), or both anthropogenic and natural sources in the case of complex particles. Aerosols from both sources can be either organic or inorganic (including a wide range of metals). Tables 1 (VOC & SVOC), 2 (BTEX & PAH's), and 3 (metals) indicate various types of chemical compounds observed in snow in various locations. There is additional information on the concentration of snow components and the methodology used to obtain their quantifications. In Table 2, the snow composition of BTEX (benzene, toluene, ethyl benzene, and xylene) and PAHs and their increase in concentration after exposure to exhaust, allowing to comprehend better how efficient snow is in the uptake of pollutants, where some of snow particles can be transformed or re-emitted to the atmosphere. Table 3 shows the concentration of various metals, including known toxic metals, observed in different types of snow, some of which were explicitly obtained in snow particles.37
Table 1 Selected observed organic compounds the Arctic, Alpine, and Urban snow. All analyses used molten snow, showing that they were in condensed phases. Many of these organic compounds have been shown to form particles and droplets78
Compound |
Type of snow |
Concentration (μg L−1) |
Technique |
Ref. |
Benzene |
Arctic |
0.437–3.76 |
SPME/GC-FID |
79
|
Arctic |
4.17 |
SPME/GC-MS |
80
|
Urban |
1.48 |
SMPE/GC-MS |
14
|
Urban |
6.48 |
SPME/GC-MS |
80
|
Alpine |
0.069–0.148 |
HS-SPDE/GC-FID |
81
|
Toluene |
Arctic |
0.271–2.81 |
SPME/GC-FID |
79
|
Arctic |
43.6 |
SPME/GC-MS |
80
|
Urban |
2.18 |
SMPE/GC-MS |
14
|
Urban |
22.6 |
SPME/GC-MS |
80
|
Alpine |
0.397–0.651 |
HS-SPDE/GC-FID |
81
|
Chlorobenzene |
Arctic |
0.104–1.08 |
SPME/GC-FID |
79
|
Arctic |
2.74 |
SPME/GC-MS |
80
|
Ethylbenzene |
Arctic |
0.008–0.132 |
SPME/GC-FID |
79
|
Arctic |
2.65 |
SPME/GC-MS |
80
|
Arctic |
1.0–1.1 |
PTI/GC-MS |
82
|
Urban |
0.13–2.7 |
GC-MS |
83
|
Alpine |
0.074–0.29 |
HS-SPDE/GC-FID |
81
|
m-/p-Xylene |
Arctic |
0.162–1.89 |
SPME/GC-FID |
79
|
Arctic |
0.65 |
SPME/GC-MS |
80
|
Urban |
8.59 |
SPME/GC-MS |
80
|
Arctic |
3.0–3.3 |
PTI/GC-MS |
82
|
Urban |
0.17–7.4 |
GC-MS |
83
|
Alpine |
0.026–0.169 |
HS-SPDE/GC-FID |
81
|
Styrene |
Arctic |
3.46–39.0 |
SPME/GC-FID |
79
|
Arctic |
0.22 |
SPME/GC-MS |
80
|
Urban |
7.82 |
SPME/GC-MS |
80
|
Trimethylbenzene |
Arctic |
0.031–1.16 |
SPME/GC-FID |
79
|
Arctic |
0.4–1.3 |
PTI/GC-MS |
82
|
Alpine |
0.030–0.477 |
HS-SPDE/GC-FID |
81
|
Dichlorobenzene |
Arctic |
7.18–20.1 |
SPME/GC-FID |
79
|
Arctic |
4.35 |
SPME/GC-MS |
80
|
1,2-Dimethylbenzene |
Arctic |
0.16 |
SPME/GC-MS |
80
|
1,2,4-Trimethylbenzene |
Urban |
4.59 |
SPME/GC-MS |
80
|
Arctic |
5.41 |
SPME/GC-MS |
80
|
Acetophenone |
Arctic |
1.25 |
SPME/GC-MS |
80
|
1-Methylnaphthalene |
Alpine |
0.838 |
HS/GC-MS |
84
|
Biphenyl |
Alpine |
1.05 |
HS/GC-MS |
84
|
Fluorene |
Alpine |
1.11 |
HS/GC-MS |
84
|
Urban |
0.001 |
SPME-GC-MS |
14
|
Phenanthrene |
Urban |
0.208 |
SMPE/GC-MS |
14
|
Alpine |
5.65 |
HS/GC-MS |
84
|
Fluoranthene |
Urban |
0.417 |
SMPE/GC-MS |
14
|
Alpine |
0.319 |
HS/GC-MS |
84
|
Pyrene |
Urban |
0.097 |
SMPE/GC-MS |
14
|
Alpine |
0.146 |
HS/GC-MS |
84
|
Naphthalene |
Urban |
0.09 |
SPME/GC-MS |
14
|
Acenaphthylene |
Urban |
0.223 |
SPME/GC-MS |
14
|
Table 2 Quantification of BTEX and PAHs in snow (in mg L−1) upon exposure of snow to exhaust engine emissions.14 They were observed in melted snow, and they were condensed. Both BTEX and PAH have been shown to form droplets105
Table 3 Reported field data concentrations of alkali, post-transition, transition, and rare metals in snow/ice matrices. Different types of metals are in different colors
Tables 1–3 reveal some key information. Firstly, there is a significant temporal and special variability (different time and location) in the measurement of various organic and inorganic compounds as well as metal analysis. Secondly, predominant anthropogenic organic compounds such as those listed in Table 1, are usually more abundant in urban areas or other polluted sites, in comparison to remote regions. This trend is not universally valid, as there are sources of anthropogenic activities in remote sites too. Thirdly, snow accommodates various particles and gaseous pollutants. Table 2 collectively illustrate that the same snow samples when exposed to gasoline exhibit much higher level of PAHs and BTEX in particulate forms. Fourthly, as shown in Table 3, in contrast to mostly anthropogenic organic compounds, snow concentrations of alkali, post-transition, transition, and rare metals are not always the highest in polluted regions. For instance, the highest concentration of iron is observed in Arctic sites due to the abundance of the Arctic dust, partly transported from the Asian deserts and deposited in the Arctic snow,27 whereas the highest concentration of cobalt is observed in snow in the Athabasca oil sands region (Alberta, Canada), as cobalt is commonly used in oil industries.40
Complementary to the chemical characterization and quantification in Tables 1–3, Fig. 3 illustrates various types of snow particle morphology, as well as particle size and number density distribution, respectively.
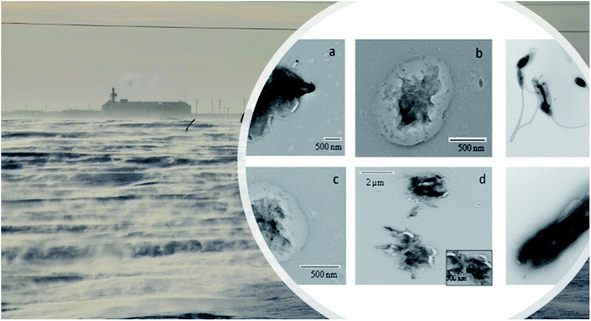 |
| Fig. 3 High Resolution Transmission Electron Microscopy (HR-TEM) images of selected biological nano-size and micron-size particles in the Arctic snow samples in an Arctic site (modification of Mortazavi et al., 2015 (ref. 29)). | |
Several technologies have been used for chemical characterization of snow. Fig. 5 depicts the analysis using electrospray chemical ionization and matrix-assisted laser-induced ablation mass spectrometry spectra of biological material in snow. High-resolution electron microscopy images identify the existence of likely bio-organic particles in melted snow samples in Alert due to the optical contrasts in the image. Electron dispersive imaging (Fig. 5) indicates that the identical particles also contain various metals such as Al, Fe, and Mg, which are consistent with the ultra-trace metal analysis in Table 3, using triple quadruple inductively coupled plasma mass spectrometry (QQQ-ICP/MS/MS) for metals. Since there are various chemical compounds in snow as particles or attached to a particle, in the following section, we only focus on a few categories of snow particles, to better understand their characteristics and impacts.
2.1. Instrumental analytical analysis
Characterization of particles in snow include the study of their chemical composition and physical properties like size and morphology, some of these include the following.
Mass spectrometry (MS): this technique is the most widely used to obtain the chemical composition of particles in snow. Common techniques using MS include GC-MS for SVOC and combustion products like PAHs.64–66 Matrix-assisted laser desorption/ionization time-of-flight (MALDITOF-MS) for large molecules like polymers and biopolymers including DNA, proteins, and carbohydrates.1,47
Induced Coupled Plasma (ICP): For the analysis of metals in snow, ICP coupled with MS,27,38,67–69 optical emission spectrometry (ICP-OES)41,70 and atomic emission spectroscopy (ICP-AES)71 are the primary methods used.
Particle sizers: these techniques give the particle size distribution in a sample. For some of these techniques, snow needs to be melted prior to the analysis. Dynamic Light Scattering (DLS)72 and Nanoparticle Tracking Analysis (NTA)1,72 are techniques that can calculate the size of a particle in a liquid based on their Brownian motion. Other techniques include the aerosolization of particles and the measure their sizes in air, these include Scanning-Mobility Particle Sizer (SMPS) Optical Particle Sizer (OPS).1,73
Microscopy: microscopy techniques such as scanning electron microscopy (SEM), and transmission electron microscopy (TEM) have been used to study particles after melting an drying74 of snow samples.26,75,76 While these techniques can analyze particles from a variety of chemical composition and sizes, the samples preparation prior to analysis can alter the particles and the distribution of particles within the samples, not to mention that obtaining particle distributions with these techniques is often challenging.77
2.2. Examples for snow-particle interactions with the earth ecosystem
2.2.1. PAHs.
Polycyclic Aromatic Compounds (PACs) contain at least two fused aromatic rings and can contain other elements other than carbon like nitrogen and sulfur, however PACs that only contain carbon are more abundant, these are known as PAHs. The primary source of anthropogenic organic aerosols found in snow is the combustion of fossil fuels and biomass burning, PACs being a common product of this combustion,85 with an estimated production of 33.2 Tg of soot particles containing PACs per year.86 Low-molecular-weight PACs occur in the atmosphere mainly in the gas phase, whereas multi-ringed PACs are bound mainly to particles.87 Studies suggest that the main source of PACs in snow is airborne particulate matter generated from local combustion,88 namely diesel engine exhaust, and coal and biomass combustion.89 PACs containing sulfur are dibenzothiophene and its derivatives are commonly found in bitumen and petroleum products indicating petrogenic activities as their origin. Canadian oil sands regions are common areas where these products are generated.39,90–92 Thia- and aza-arenes are PACs containing nitrogen that can be found in bitumen, but also can be generated by combustion and mining of oils sands.39,90,93,94
The EPA considers 16 PAHs as priority pollutants, however, pyrene, phenanthrene, naphthalene, and fluoranthene have been identified as the most common PAHs in snow,89 and several of these are known as carcinogenic compounds.95 Other studies have indicated that many of the PAHs found in snow may end up in rivers and other water bodies after snowmelt in spring as part of the natural hydrological cycle of snow.96 PAHs can also have toxic effects on aquatic organisms or be absorbed by plants and consumed by terrestrial animals making their way up in the food chain.49 Upon snow melt, the release of airborne particles has been observed.15 Human exposure is through inhalation of air and re-suspended soil and dust, consumption of food and water, and dermal contact with soil and dust.15
2.2.2. Secondary organic aerosols.
Organic aerosols can be produced from volatile and semi-volatile organic compounds via atmospheric photo-oxidation and condensation processes. These particles are called Secondary Organic Aerosols (SOA). They can be further deposited on snow.97Table 1 shows reported data of volatile and semi-volatile compounds found in snow. Precursors of SOA can be anthropogenic and biogenic in origin, however, biogenic sources produce 5.5 times more SOA precursors than anthropogenic sources. A large portion of these precursors are terpenes, which are usually oxidized by ozone, NO3, and hydroxyl radicals among other oxidants.98,99 It is also considered that many SOA are formed when partially oxidized organic vapors condense on ice particles and undergo chemical reactions. These are removed from the atmosphere when the snow precipitates.100 SOA can act as ice-nucleating particles (INPs), but also water can condense on their surface to form water droplets and then act as INP.101
2.2.3. Water soluble organic particles.
Water-soluble organic particles are released in high concentrations during the early stages of the snow melting process, whereas hydrophobic compounds and particle-bound compounds are released at later stages.102,103 Depending on the snowpack depth, the release process efficiency differs. Shallow snowpack, for instance, experiences an irregular melting process (numerous melting episodes over winter), and thus releases small quantities of chemicals and particles throughout wintertime.104
2.2.4. Biological particles.
Another type of organic aerosols of biogenic origin are bioaerosols, which include microorganisms and remnants of biological activities such as reproductive material (e.g., pollen) and decaying matter.106 Forested areas represent the major source of bioaerosols inland, yet, oceans are proposed to be the major sources of bioaerosols overall. It is believed that bubble-bursting in the ocean surface layer is a predominant pathway in which bioaerosols are released from the oceans into the atmosphere.107 It has been revealed that up to 25% of insoluble aerosols are bioaerosols,108 which can travel long distances and end up on snow.109–111Fig. 3 and 5a–d show some examples of particles, including biological particles of different sizes, from a few nanometers to a few micrometers, in Arctic snow samples.
Next generation sequencing (NGS) technology or massively parallel sequencing approach to DNA sequencing,27 has allowed the rapid sequence of the entire genome of a species like humans. NGS has also evolved in environmental microbiology genomic research significantly. During the last decade, the usage of NGS paved the way for further understanding of the large types of biological particles in snow, such as bacteria, in urban and remote sites.29 Most environmental microbes cannot be cultured.28,112 NGS provides an opportunity to identify all potential bacteria communities or other microorganisms in a given snow sample. NGS analysis may allow distinction between bacterial communities in the Arctic and Urban snow.27,113 For instance, in one study, the snow genomic analysis known phyla or candidate divisions were detected with the majority of sequences related to one of the five major phyla: Proteobacteria, Actinobacteria, Bacteroidetes, Firmicutes, and Cyanobacteria. As shown in Fig. 3, the morphology of biological particles was obtained using high resolution electron microscopy with energy-dispersive X-ray. NGS analysis led to the identification of metal interacting bacteria particles in all types of snow and frost flowers in the Arctic.27 The combination of various cutting-edge mass spectrometry techniques, cryo-high-resolution electron microscopy and various optical sizers, along with NGS and culture methods, may allow much more comprehensive understanding of the role of snow biological particles in the future.
2.2.5. Inorganic particles and mineral dust.
Like organic aerosols, inorganic aerosols in snow can be of anthropogenic and natural origins. Mineral dust particles are one of the largest contributors to the formation of snow.114 Mineral dust particles are generated from soil and ground by the effect of rapid winds. The common elemental composition of mineral dust includes metal elements like alkaline metals and transition metals (Fig. 5e and f). Deserts such as the Saharan desert in Africa and the Gobi and Takla-Makan deserts in China, are abundant sources of mineral dust in the Northern Hemisphere.115 In the following sections, we discuss how dust and black carbon particles in snow can affect snow albedo and physicochemical processes such as melting.
2.2.6. Other snow particles like sea spray and volcanic particles.
In the Arctic and sub-Arctic regions, sea spray, volcanic activities, and forested areas are among the most critical sources of particles. There is, consequently, a wide diversity in size distributions of particles in snow in the Arctic and sub-Arctic regions, as shown in Fig. 4,116 which depicts the size-aggregated particles, including nanoparticles in snow, that exchange with the atmosphere.15 Long-range transport of mineral dust, organic particles, and soot, among other particles, to the Arctic region further enriches the chemical diversity of particles that can be deposited on snow.117
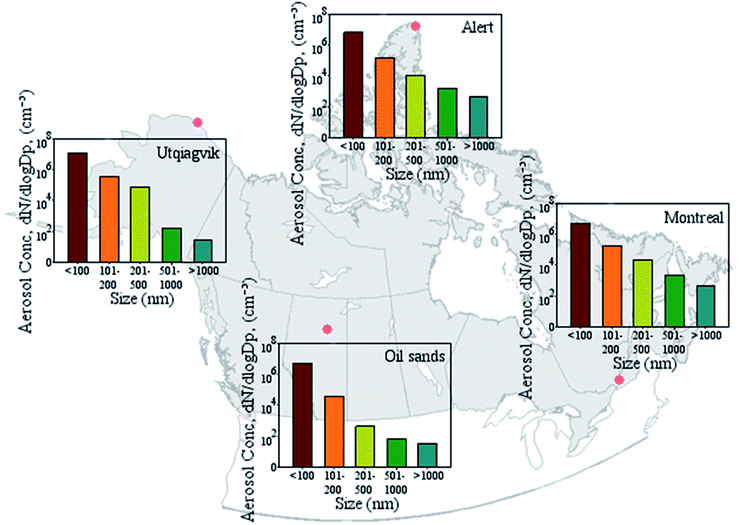 |
| Fig. 4 Abundance of different size fractions in North American snow. Data obtained from references.1,102 The data was obtained using a scanning mobility particle sizer and an optical particle sizer with size limits from 11.5 to 9033.88 nm. Each color represents a different size fraction, which is on x-axis. | |
Most of the aerosols generated by sea spray, volcanic activities, and forested areas are salts (like Cl−, Na+, Mg2+, Ca2+, K+, NO3−, and SO42−), along with organic components including biological compounds or organic remnants of biological processes like microorganisms' debris, membrane proteins or organelles.118 Forested areas and vegetation are also among those generating biological particles (Fig. 2).119 In general, unless the Arctic region is close to a significant source of particles, such as mining activities, the concentration of chemicals is smaller in contrast to urban regions (Table 1). Particles in the range of <200 nm are the most abundant particle sizes in snow and comprise 38–78% of the total number of particles (by mass, smaller particles make up a small fraction of all particles), with 11–19% under 100 nm.1 Atmospheric aerosol size distribution systematically shows that nano-size particles (<200 nm) are the most abundant particles in the air in cold climate.15 Hence, there is a similar trend with the abundance and size distribution of aerosols in the atmosphere and fresh snow, confirming that most upper layers of snow exchange efficiently with the atmosphere. Clearly, aged snow particles are expected to influence on surfaces such as soil and water, and upon freezing-thawing periods.120
2.2.7. Importance of snow particles in selected photochemical processes.
There are several field observations as well as experimental and modelling research pointing to the importance of photochemical and/or heterogeneous reactions in the presence of snow borne particles.124,125 For example, researchers have found that not only the snow albedo but also the photochemical processes can occur as a function of the black carbon content in snow.124 Snowpack is an effective venue for photochemistry.55,56,126 It has also been shown that increasing black carbon concentration in the snow reduces the rate of photolysis of impurities and pollutants in the snowpack such as nitrate.127–129 Furthermore, the redox reactions of toxic compounds such as mercury are shown to be affected by snow particles and biological particles.24,130 Further research is recommended to evaluate the complex (photo)chemical reactions in snow matrices in the presence of a variety of particles.
2.3. Why do we care about snow-borne particles?
Here we summarize a few processes that make particle-bound species in snow important to understand in the Earth's ecosystem. The role of particles in the ice nucleation and potential implication on freezing–melting processes are discussed separately in Section 3.
2.3.1. Albedo (reflectivity).
In brief, the importance of snow particles such as black carbon on radiative properties (albedo), as well as changing properties and air-snow interactions have been studied.131 Previous research has found a significant correlation between black carbon in snow and spectral surface albedo.131 For example, the addition of even 10 ng per gram of equivalent black carbon in snow lowered the snow's albedo by 0.004, noting the recorded concentrations of black carbon in ice cores usually range from 0.01 to >20 ng g−1.131,132 Increasing the amount of the equivalent black carbon by an order of magnitude further results in the albedo reduction effect increasing 5-fold.131 Current atmospheric models fail to predict with high accuracy the real distribution of particles in the atmosphere, including the transport of particles from their sources to snow-covered areas and their deposition, as well as their effects on snow albedo, snow grain shape, and the particle-snow internal mixing.133,134 Particles that change snow albedo can also modify the melting and freezing processes of snow.27 Black carbon reduces snow albedo, which increases the absorption of radiation, resulting in early snowmelt.135,136 Research has also shown that trace amounts of dust, HULIS (HUmic Like Substances) and black carbon deposited on or within a snowpack can reduce its albedo relative to pure snow.137,138
2.3.2. Ecosystem and health.
As mentioned earlier, certain particles in snow are hazardous to ecosystems and human health (such as PAHs and toxic metals).121 Furthermore, with the development of newer cutting-edge technologies, emerging contaminants such as nano- and micro-plastics are being detected in the Arctic and urban snow.52 During the precipitation, melting, and evaporation cycle of snow, these contaminants are released back to the air and surrounding surfaces like soil. More water-soluble species can also be released into aquatic systems especially as snow melts in spring. This results in the discharge of pollutants stored in the snowpack into the environment. Exposure to PACS and PAHs from the snowmelt can lead to toxic effects like reduction and delay of reproduction of aquatic lifeforms, increased physiological sensitivity, and reduced physical abilities like swimming and aerobic capacity.139,140 Other effects include increased indices of cancer and death.94 Toxic effects of metals in snowmelt contaminated by oil sands include increased sensitivity of fish and amphibian species and delays in some developmental stages.141,142
2.3.3. Importance of addressing the shortcomings of current air quality and aerosol.
Numerical models currently provide regional and global understanding of particle distribution in snow, aerosol-cloud interactions, and reactions at snow/ice surfaces.143 These models use simplified schemes to investigate the role aerosols and aerosol-cloud interaction play in air pollution.133,143 However, uncertainties make the prediction of air quality, cloud processes, and climate change challenging.4 Furthermore, blowing snow transport and sublimation can further change the surface distribution of snow, redistribute ice particles vertically, and cool and moisten the atmospheric boundary layer.144 In a northern urban setting, pollution from anthropogenic activities, namely local traffic and industry combined with long-range transport, adds to the complexity of snow composition and makes the understanding of snow processes more difficult.145 Yet, they should be understood, as they are required for regional and global air quality and the climate processes.
2.3.4. Snow as a reaction medium: scavenging and transformation of particles.
During or after snow formation, aerosols can be scavenged in clouds. Scavenging of aerosols can also occur after snow precipitates from the clouds. Snow crystals and scavenged aerosols are deposited on the Earth's surface via precipitation.146 The time it takes for an aerosol particle to be deposited onto the Earth's surface depends on different factors, one of which is the particle size. In general, particles larger than 10 μm do not remain suspended long enough to be removed through wet deposition.147 Evidence suggests that dry deposition is the dominant way in which large particles are removed from the atmosphere and settle on snow.148 Smaller particles and trace compounds are preferentially removed via wet deposition.149 Common species that are deposited with falling snow are black carbon (soot), metals (e.g., Mg, Al, V, Mn, Fe, Co, Cu, As, Se, Sb, Tl, Pb), and ions or salts (e.g., Cl−, Br−, NO3−, SO42−, C2O42−, NH4+, Na+, K+, Mg2+, Ca2+). Black carbon (including carbon nanostructures) is poorly removed from the atmosphere through dry deposition processes. A recent study have found that ice nucleation and cloud condensation nucleation are the predominant pathways in which black carbon particles are scavenged and removed,150,151 although ice could also reduce the rate of wet scavenging of black carbon particles due to Wegener–Bergeron–Findeisen process.151 Particles containing organic compounds can also be scavenged or deposited on snow. In general, large, non-polar organic particles are more efficiently scavenged by snow than rain, while small, polar molecules are better scavenged by rain, especially at ∼0 °C, as polar species are more soluble in water than ice. Below −10 °C, organic compounds tend to be preferably scavenged and deposited by snow.152
Soon after snow falls on the ground, the ice crystals start changing in a process called snow metamorphism. Snow metamorphism is the change of the crystal structure of snow due to processes like melting–freezing and sublimation–deposition, or recrystallization processes that happen within snow. This process keeps happening even after fresh snow is deposited onto the snowpack. Due to metamorphism, the different chemicals in snow can be transformed and redistributed within the snowpack. After snow melts, the newly formed or transformed chemicals can be released into the environment. For instance, the concentration of incorporated organic particulate matter can increase when particles are deposited into the snowpack, which in turn will likely reduce the snow albedo.143 The reduction of snow albedo is due to a higher concentration of insoluble light-absorbing particles, like black carbon, which reduce the diffuse reflection of solar radiation within snow.153 A recent study154 has shown that a loss in dissolved organic carbon concentration can be expected after fresh snow metamorphizes into fine firn in a glacier due to early snowmelt, while an enrichment can be expected after fine firn becomes granular ice due to erosion of surrounding surfaces, and therefore releasing DOC, when firn melts (late snowmelt) and becomes granular ice. Upon snow metamorphism, black carbon particles within the snowpack can decrease snow albedo, contributing to an early snowmelt in spring.155 Further studies on the diverse roles of snow particles are recommended.
2.3.5. The potential impact of salt particles on air quality.
In the Arctic, it has long been established that there is halogen exchange between the upper layer of snow and the atmosphere.59,156–158 In urban regions, during the cold seasons, different materials are used for de-icing roads. The most commonly used materials worldwide are salts (e.g., NaCl, CaCl2 and MgCl2).159 Recent studies have shown that the addition of salts in wintertime coincides with elevated Cl− concentrations in PM2.5 aerosols and snow.18 Additionally, halogens can react with various organic and inorganic species in these cold urban regions, and thus affect the atmospheric chemical composition. For instance, a significant amount of photolabile chlorine (e.g., ClNO2, Cl2, etc.) has been observed in Montreal, Canada, during wintertime.118 The city receives approximately 2.1 m of snow and annually uses 140
000 tons of salt (NaCl, CaCl2 with additional gravel at different temperatures), to de-ice roads.160 Other studies have identified road salts as the primary source of chloride-containing aerosols (e.g., ClNO2 that is considered a source of nitrogen oxides and chlorine radicals in the air) in inland urban areas in the wintertime.17 Because of the abundance of anthropogenic reactive halogens in urban and remote industrial sites, their interactions with snow affect the air quality and biogeochemistry of these regions.
2.3.6. Little known processes.
There are gaps of knowledge in our understanding of contaminant cycling at aerosol/air, snow/air, ice/air, and air/water interfaces. Additionally, interfacial processes such as absorption–adsorption, homogeneous and heterogeneous reactions, photochemistry, and biological transformations are inadequately understood. Particle size distributions and their chemical compositions in snow can be determined with cutting-edge technologies such as optical spectroscopy and cryo-electron microscopy. However, little is still known about the details of exchange and transformation processes.
2.4. Air-snow transfer of particles: a case study for snow exposure to vehicle exhaust
The transportation sector is a major source of primary particles and gases that undergo gas to particle conversion (e.g., vehicular emissions such as diesel exhaust) in urban snow.1 Research has shown that ice and snow can interact with particles by providing a large surface area in which pollutants (like soot and PAHs) can be adsorbed and then undergo physicochemical reactions.13,55,57 Additionally, the low temperatures of snowpack can promote condensation of low-volatility organic gases which can then oxidize and form SOA.161
The existence of snow and cold temperature can affect the snow-air exchange processes. Low temperatures can also reduce the motion of smaller particles, which decreases the collision–coalescence processes that form large particles.162 When snow melts, nanoparticles can be released back into the environment through the meltwater;163 this way snow can act as a source or a sink of nanoparticles under different environmental conditions.
In a laboratory experiment done in an environmental chamber (Fig. 6), it was found that both snow and exhaust emissions affected each other. Snow modified the size distribution (a shift of the modes to lower sizes) of exhaust, presumably by limiting its growth.16 Additionally, exhaust exposure changed the chemical composition of snow. The concentrations of benzene, toluene, ethylbenzene, xylene (BTEX), and some PAHs were higher in snow samples subjected to exhaust emissions compared to fresh snow, as shown in Table 2.16
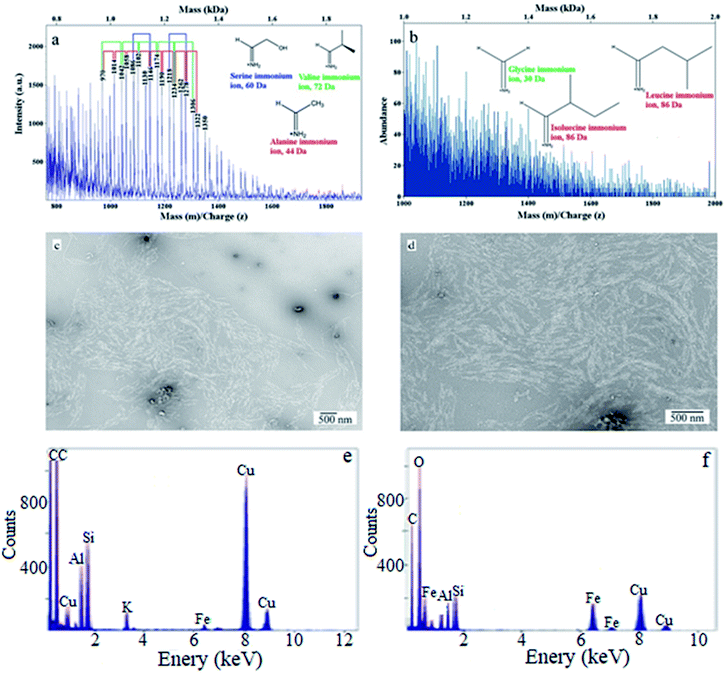 |
| Fig. 5 Images (a and b) show electrospray chemical ionization and matrix-assisted laser-induced ablation mass spectrometry spectra of biological material in snow, and images (c and d) are high resolution electron microscopy images identifying the existence of likely bio-organic particles in melted snow samples in Alert due to the optical contrasts in the image. Images (e and f) show electron dispersive imaging indicating that the same particles also contain various metals such as Al, Fe, and Mg. The analysis was done on a copper grid1 and thus the Cu signal is predominantly from the grid itself. | |
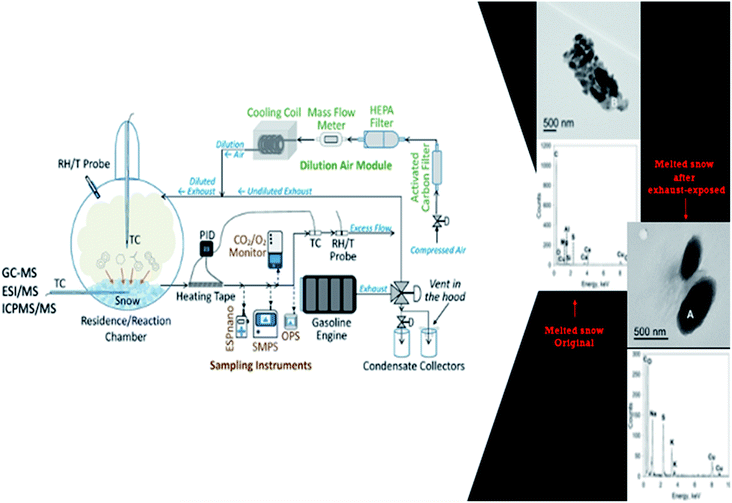 |
| Fig. 6 At LHS, a simplified schematic of snow chamber-exhaust facility is shown.16 At RHS, high-resolution transmission electron microscopy (HR-TEM) with energy dispersive spectroscopy (EDS) of duplicate melted snow samples in a site before and after exhaust exposure, are illustrated. An increase of particles in snow upon gasoline exposure was also observed. | |
3. The role of particles in ice nucleation processes: impacts on freezing-melting process
Clouds are required for snow precipitation, but not all clouds make precipitation.7 Indeed, only a tiny fraction of clouds precipitate, such as nimbostratus clouds that can produce snow (Fig. 1).7 Several physicochemical processes are needed, from the formation of ice nuclei and their growth, to snow precipitation. In this section, we explore a brief background about the role of particles in heterogeneous nucleation processes, and examples of different compositions and sizes of ice nuclei.
3.1. Heterogeneous ice nucleation: a brief survey of theory
Homogeneous nucleation is a process in which water vapour molecules become solidified or frozen, in absence of a foreign phase (aka a nucleating agent), at low temperatures (≤37 °C).7 Heterogeneous nucleation follows almost the same concept of homogeneous nucleation. However, this type of nucleation involves preferential sites such as phase boundaries, surfaces, or impurities like aerosols such as dust.164 Aerosols serve as nucleating agents on which ice crystal growth occurs. In heterogeneous nucleation, due to lower surface energy, the free energy barrier reduces and facilitates nucleation at these preferential sites. The existence of aerosols renders heterogeneous nucleation to require less activation energy than homogeneous nucleation. As such, the freezing process occurs at a higher temperature in heterogeneous nucleation processes in comparison to homogeneous nucleation processes.
There are four different types of heterogeneous nucleation: these are (a) deposition freezing, (b) condensation freezing, (c) contact freezing, and (d) immersion freezing, as shown in Fig. 1.
To better understand heterogeneous nucleation, we consider a hypothetical scenario that the nucleation proceeds via immersion freezing. When a spherical nucleus is formed at a preferential active site (considered from here on a surface), the spherical surface is reduced, and thus the area of the interface between the nucleus and the surrounding fluid, for instance, supercooled liquid water, is less than 4πr2. This geometric reduction decreases the surface area term in eqn (1) and thus lowers the nucleation activation barrier.165
| 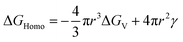 | (1) |
Here Δ
GV is the difference in Gibbs free energy per unit volume between the thermodynamic phase where ice nucleation is taking place and the phase that is nucleating,
r is the ice nucleus radius, and
γ is the surface energy/tension between the ice nucleus and its surroundings. At very low values of
r, the surface term (
r2 term) dominates, and the free energy is positive, and at higher values, the volume term (
r3 term) dominates, and the Gibbs free energy is negative.
In the Earth's atmosphere, heterogeneous nucleation is achieved using aerosols as seeds for heterogeneous ice nucleation (Fig. 1). For heterogeneous ice nucleation, eqn (1) can be corrected by a factor to compensate for the loss of surface area and volume.
| ΔGHeter = ΔGHomo × f(θ) | (2) |
where
f(
θ) is a function of the contact angle between the particle and the ice embryo.
166 | 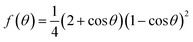 | (3) |
The contact angle (θ) is the degree between the interactions of the ice embryo with the INP. It should not be confused with the contact angle used to measure the wettability of a surface that is commonly used in material sciences. Geometric observations show that as the contact angle decreases, and so does the spherical surface area. Therefore, the smaller the contact angle, the lower the activation barrier and hence, the faster the nucleation rate.165
In laboratory studies, the contact angles of ice nucleating materials, including those found in snow, are usually calculated as described in Marcolli et al. (2007).167 Contact angles (α) were calculated from the compatibility function fhet for supercooled water.
| 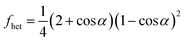 | (4) |
Which is responsible for the reduction of the Gibbs free energy ΔG barrier in the presence of an ice nucleating particle.
| 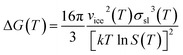 | (5) |
where
νice is the volume of a water molecule in ice,
σsl is the interfacial tension between water and the ice embryo, and
S is the ice saturation ratio. These parameters were calculated as in Zobrist
et al. (2007)
168 using the heterogeneous ice nucleation rate coefficient
jhet and the surface area of the ice nucleating particle in a droplet of water.
Particles with small contact angles tend to be more efficient at nucleating ice than large contact angle particles. Water suspensions of various environmental particles, including those found in snow, have been shown to exhibit strong properties to act as effective ice nuclei.169,170 For example, long-term studies of snow in the Arctic and sub-Arctic and mid-latitude sites indicate that melted snow freezes above −10 °C,1,171 even after removing particles larger than 200 nm, indicating that snow nano-size particles, including nanoparticles can be good ice nuclei. These agglomerates of nanoparticles, still nanosized, have a high surface-to-volume ratio and sufficient curvature, to have adequate contact angle for ice nucleation. A few laboratory studies have shown that such nano-size agglomeration of different materials can lead to high ice nucleation capability.169,170,172
Diverse types of particles are suggested to act as INP in the atmosphere, e.g., metal containing particles (e.g., Fe2O3), mineral dust, soot, and biological particles (e.g., airborne bacteria and viruses), and emerging contaminants such as nano- and micro-plastics.97,172 INP can also nucleate ice directly from water vapor or act as cloud condensation nuclei to form a water droplet and then freeze it.173 Three factors are predominantly responsible for enhancing the rate of nucleation: (i) an overlayer of water acting like a template material, having a similar crystalline structure to ice;169 (ii) the appearance of a contact layer, being buckled in an ice-like fashion, and (iii) improved nucleation on compact surfaces, having very strong adsorption energy.174
3.2. Examples of particles' impact on ice nucleation and freezing–melting processes
Previous studies have confirmed the presence of different microorganisms (e.g., bacteria, algae, and viruses) on snow and glacier ice.175 For example, some groups have investigated the genomics of bacterial populations in snow,26 identifying five major phyla: Proteobacteria, Actinobacteria, Bacteroidetes, Firmicutes, and Cyanobacteria, several of which have properties like ice nucleating.176 Beside bacteria, several other types of microorganisms have been found in Arctic snow which seem to have resistance to heavy metals.27Fig. 3 and 4 illustrate the presence of biological material in Arctic snow samples and particles containing various metals, demonstrating how diverse particles in snow are and how biological matters coexist with various types of particles, including nanoparticles, which are abundant in snow.
During the last two decades, research has been focusing on understanding how some of the most efficient INPs work, namely the bacterial ice-nucleating protein (InaZ),177 and how some processes affect immersion freezing of well-known INP (e.g. the oxidative polymerization of catechol on dust particles178). There has also been researches on novel materials that can act as INPs like graphene–graphene oxide nanoparticles179 and inorganic composite particles.170 Recent studies indicate that several types of anthropogenic particles, including emerging compounds such as nano- and micro-plastics and synthetic complex inorganic materials,169,172 in addition to dust and biological particles, can serve as effective ice nuclei.
Human activities continuously create new materials, including nano- and microparticles, which are released in the environment. Such particles are detected in the air,43 and they are deposited15 in snow. As such, there will be likely new ice freezing nuclei and anti-freeze materials, which will be released in the Earth's ecosystem, facilitating freezing or melting processes in the future.
4. Emerging contaminants in snow
Emerging contaminants refer to synthetic or natural compounds that are not monitored in the environment regularly. Yet, these compounds are known (or suspected) to have adverse effects on the ecosystem or/and on human health.180 Emerging contaminants are considered to be of serious concern due to their potential health effects. Recent scientific and technological advances allow ultra-trace quantification of selected contaminants in the environment. Recent innovations in medical field allow researchers to assess health impacts of selected emerging contaminants. Such pollutants are everywhere, from microplastics in oceans to nanometals in electronic waste.181 These emerging contaminants have also been observed in various types of snow, in Arctic regions, industrial sites, and Urban settings.158
Emerging contaminants include synthetic or natural micro-organisms and their products, which can adversely affect ecosystems and human health. These contaminants are widely distributed in the environment, from microplastics in oceans to nanometals in landfills. An example of this is the Athabasca Oil Sand Region in Alberta, Canada, where the extraction and processing of oil sand generate aerosols that can be deposited in snow. Snow from this region contains high concentrations of C, Si, Al, and Fe.37,39,182
The concentrations of metallic species in snow, compiled from literature, are shown in Table 3. These species can be of lithogenic origin. They are released into the environment by activities and processes such as mining, land clearing, and wind-blown dust.182 Metallic species that are recognized as priority pollutants by U.S. EPA and WHO (Cr, Ni, Cu, As, Se, Cd, and Pb) have also been detected in snow from oil sand regions54 at concentrations that are 10 to 25 times higher than those in urban areas.
Snowmelt runoff can introduce these metals into aquatic bodies, which ultimately become concentrated in aquatic life and irrigated food crops. Consumption of contaminated fish and crops increases human exposure to these toxicants.183–185 Recent research confirms the existence of nano- and microplastics (<5 μm) in snow.44,186,187 These particles are in low concentrations but are present globally – from the Arctic to the Alps and cities and Arctic sea ice.44,188,189 The sources of these particles are suspected to be the protective coatings on ships, vehicles and buildings, containing various plastic materials such as polyethylene and polystyrene.44
In recent decades, there has been rapid development of analytical techniques (Table 3) and remediation technologies relevant to emerging contaminants. For instance, several techniques have been used to detect microplastics in environmental samples like Raman spectroscopy and thermal assisted mass spectrometry.190,191 However, pre-concentration methods are required, especially in the case of snow where concentrations can be in the ultra-trace level. New techniques are being developed and applied to the ultra-trace analysis of microplastics like nanostructured laser desorption/ionization time-of-flight mass spectrometry (NALDI-TOF-MS).47 This technique allows direct micro-/nano-plastic analysis in different environmental matrices like snow, without requiring pre-treatment. It is beneficial to continue with the research and development of tools to detect and quantify microplastics.
4.1. Potential health impacts of microplastics
Large particles of plastics or macro-plastics have shown to have adverse health effects on the Earth's ecosystem. For instance, upon ingestion of plastics, certain wildlife starve to death or suffocate by plastics.192 During the last decade, several studies suggested the potential adverse health effects of nano- and micro-plastics in ecosystem and human health.193 There are a couple of evidence of health impacts in other animals.194–197 One of these studies has shown that microplastic may affect the genome by inducing transcriptional change, immune response, and behaviour alteration in adult zebrafish.196 In the second study,197 the analysis of juvenile Daphnia revealed a variety of subtle responses of morphological traits. Yet, in adult Daphnia, alterations in the expression of genes related to stress response genes involved in body function and body composition were observed already 48 h after exposure. As for human exposure research, a recent study has evaluated microplastic consumption by humans.48 They estimated that Americans consume 39
000 to 52
000 microplastic particles per year, depending on age and sex. These estimates increased to 74
000 and 121
000 when inhalation was considered. In brief, macro-plastic adverse health effects are well-established. However, there is growing evidence for other animals, and the adverse effect of microplastic on human and ecosystem health are likely, but further research is required to further substantiate it.
5. Light-absorbing carbonaceous particles in snow
5.1. Sources of LACs
Light-absorbing carbonaceous particles (LACs) consist of black carbon (BC) and light-absorbing organic carbon (i.e., brown carbon, BrC). The primary source of BC are fossil fuel combustion and biomass burning. The dominant component of BC is elemental carbon (EC) which makes it strongly absorb solar radiation ranging from UV to near infrared. However, the sources of BrC are more diverse. BrC primarily comes from biomass burning and fossil fuel combustion, whereas the secondary sources include photochemical reactions of anthropogenic or biogenic secondary organic aerosol precursors,209–212 and heterogenous reactions with NOx.210–213 The complex matrices of BrC make it difficult to determine every component of BrC, but there are several representative compounds that have been proved to be BrC, such as HULIS, nitroaromatics, PAHs, and vanillin.210–212,214
LACs can undergo long-range atmospheric transport (LRAT) and then deposit on the surface of snow and glaciers. During this process, both wet deposition and dry deposition can take place.201 For dry deposition, when the size and weight of LACs gradually increase, it would be easier for LACs to be deposited by wind. For wet deposition, which is the main deposition of LACs, there are more ways to deposit LACs. For instance, LACs can serve as ice nuclei or cloud condensation nuclei to form ice, cloud, or rain. They can also be scavenged by precipitation. Although there are very limited human activities in the cryosphere like Arctic,215–218 Tibetan Plateau219 and Alps,220–222 LACs can be deposited in cryosphere by LRAT, affecting regional and global climate in the end.
5.2. Optical properties of LACs
Absorption coefficient (Abs) is usually used to describe the absorption of LAC particles in a power-law relationship with absorption Angström exponent (AAE),where Abs is with the unit of Mm−1; K is a constant; l is the wavelength of light with the unit of nm. Once the mass concentration of LACs is determined, mass absorption cross-section (MAC) or mass absorption efficiency (MAE) at a specific wavelength can be calculated by eqn (7), | 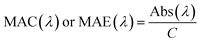 | (7) |
where MAC or MAE is with the unit of m2 g−1; C is the mass concentration with the unit of μg m−3. MAC is usually used to describe the optical property of BC or BrC particles, whereas MAE is used to describe the optical property of BrC solution.211
AAE of BC is close to unity. Consequently, BC shows less dependence on wavelength, strongly absorbing light from UV to near infrared. However, AAE of BrC varies from smaller than 1 to as great as 8,230 indicating high wavelength dependence. As a result, the absorption of BrC sharply decreases with increasing wavelength, with strong absorption on UV but weak absorption on short-wavelength visible light. The value of AAE of BrC can be calculated in a selected range of wavelength by eqn (8),
| 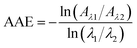 | (8) |
where
Aλ is the absorbance of BrC at wavelength
λ, which can be measured by UV-vis spectrophotometer.
The measurement of BC absorption has been well summarized by Bond et al.223 Briefly, there are various instruments based on several methods to measure the absorption of BC: (1) photoacoustic method, such as photoacoustic extintiometer (PAX); (2) filter-based optical absorption method, such as aethalometer; (3) thermal-optical method, such as thermal optical reflectance (TOR); (4) laser-induced incandescence method, such as single particle soot photometer (SP2). Compared with the diverse methods for BC, to our best knowledge, there is no commercial instrument designed specifically for BrC. Although PAX has a short-wavelength laser module, it cannot distinguish mineral dust from LACs. Absorption measured by aethalometer can be used to estimate the contribution of BrC absorption only if the value of AAE is determined, whereas AAE for BrC in the ambient air will not be constant. Current methods to determine the absorption, mass concentration, and chemical constitutions of BrC are filter-based. Generally, samples are collected on one filter and then are subtracted by water or methanol to do further analyses, including total organic carbon (TOC) analysis, UV-vis spectrophotometry, and GC-MS or LC-MS. The filter-based methods will not change the chemical properties of BrC. However, they may change the size distribution and morphology of BrC, causing bias in measuring the optical properties of BrC.
Fresh BC particles are nanosized spheres with sizes less than 100 nm.223 Once emitted, BC will undergo internal or external mixing with other components in the air, including BrC, to form aggregates with sizes ranging from nanometers to micrometers.223 Internal mixing or external mixing with BrC would also modify the optical properties of BC to cause enhanced absorption, which is termed as “lensing effect”.211,224,225 In turn, the size and morphology of BC can affect light absorption of BrC, causing overestimation on MAC of BrC.226,227 In addition, the effects of atmospheric evolutions of BrC are more complex. On the one hand, photochemical or heterogeneous reactions could facilitate the formation of new BrC components and lead to absorption enhancement.210,211 On the other hand, these reactions may cause photobleaching of BrC,210,211 decreasing the absorption contributed by BrC. The complex matrices of BrC make it difficult for researchers to comprehensively evaluate the overall impacts of various atmospheric processes on BrC, which, in the end, hinders the work on accurately modeling the climate effect of BrC.
5.3. The radiation impacts of LACs deposited on snow
The radiation impacts of BC have been comprehensively investigated and evaluated by other researchers.201,215,216,223,228 To be brief, BC deposited in the cryosphere mainly comes from anthropogenic sources like fossil fuel combustion in winter and biomass burning in summer.201 The deposition of BC in snow can cause radiative forcing (RF) with a few watts per square meter in fresh snow. Nevertheless, as snow ages, RF induced by BC will gradually increase to even as large as hundreds of watts per square meter, leading to the acceleration of glacier melt.114
Unlike BC, although BrC in snow has drawn more attention in the past two decades, there are only a few studies which investigated and evaluated the optical properties and RF of BrC in the cryosphere. Wu et al. summarized the knowledge of MAE and AAE of BrC but did not include the data of RF.225Table 4 provides a summary of up-to-date knowledge of the optical properties and RF of BrC in the cryosphere. As shown in Table 4, the RF of BrC is sometimes described as a ratio of radiative forcing of BC, which is due to lack of knowledge of precise components of BrC. As discussed above, the complex constitutions of BrC make it challenging to determine the mass concentration of BrC, and thus MAC of BrC can be either overestimated or underestimated. The RF of BrC can only be estimated as a ratio of RF of BC. And for the cases which provide RF in the form of numbers, the values of RF of BrC are not accurate either. Additionally, it is evident that AAE of BrC varies more than AAE of BC, which confirms the strong wavelength dependence of BrC. Moreover, the variation of AAE indicates BrC consists of both strong absorbers and weak absorbers, making it hard for model researchers to parameterize the optical properties of BrC, causing deviation in modeling RF of BrC in the end.
Table 4 The optical properties and RF of BrC in the cryosphere. aIndicates the measurement was done on dissolved organic carbon (DOC). bIndicates middle altitude of Asia. cIndicates the measurements were done on water-soluble BrC. dIndicates the values were reported as MAE; eindicates the measurements were done on water-soluble organic carbon
Site |
Sample type |
Method |
MAC (m2 g−1) |
AAE |
RF (W m−2) |
Ref. |
Tibetan plateau |
Snow |
UV-vis spectrophotome-ter |
1.4 ± 0.4 (365 nm) |
5.0 ± 5.9 |
0.43 |
248
|
Laboratory |
Snow |
UV-vis spectrophotome-ter |
N/A |
4.12–6.28 (330–400 nm) |
1.23 per ppm of BrC |
249
|
Altai mountaina |
Snow |
UV-vis spectrophotome-ter |
0.45 ± 0.35 (365 nm) |
2.59 ± 1.03 |
0.11 |
250 |
Global Arctic Asiab |
Simulated in snow |
GEOS-Chem, MERRA2, RRTMG |
0.46–1.7 (300–700 nm) |
N/A |
0.0064, 0.13 (Arctic summer) |
215
|
0.24 (Asia winter) |
Arctic |
Snow |
Integrating-sandwich spectrophotome-ter, SP2 |
N/A |
3.5–7.0 |
N/A |
216
|
Arctic |
Aerosolc |
UV-vis spectrophotome-ter |
0.10–0.98 (365 nm)d |
8.5 ± 4.2 |
5–34% of BC |
217
|
Arctic |
Aerosole |
UV-vis spectrophotome-ter |
0.70 ± 0.44 to 1.54 ± 0.75 (365 nm)d |
2.91 ± 1.02, ∼4.76 ± 2.28 |
N/A |
218
|
Furthermore, neither experimental methods nor modeling methods could provide a relatively precise concentration of BrC in the atmosphere or snow/ice. For experimental methods, both water extraction and methanol extraction methods would discard insoluble organic carbon which may contain a fraction of BrC. The following absorption measurement by UV-vis spectrophotometer cannot reflect the contribution from insoluble BrC. For modeling methods, currently, the mass concentration of BrC is estimated by using a certain percentage of organic carbon,229,230 which no doubt would cause deviation in the radiative impacts of BrC.
5.4. Ice nucleation of LACs
5.4.1. Ice nucleation of BC.
The ice nucleation of BC has been widely investigated in the past decades. However, the question whether BC particles are important and efficient INPs in the atmosphere is still under hot debate because there are many conflicting results from both laboratory experiments and field campaigns.223,231 For example, some early research indicates BC can nucleate ice below −20 °C,223,232,233 and can be as ice active as mineral dust.233 However, later experiments found contradictory results, suggesting BC particles are poor ice nuclei.223,234–236 Even for field campaigns from the same site, results can be contradictory. Cozic et al. reported BC enrichment in ice particle residuals, and therefore BC may be able to nucleate ice efficiently in the mixed-phase cloud at Jungfraujoch, Switzerland.220 However, at the same site, Kamphus et al. and Kupiszewski et al. found that BC was depleted in ice residuals, indicating BC was unimportant in mixed-phase cloud ice nucleation.221,222 One of the reasons for controversial results of ice nucleation abilities of BC is that BC may undergo different modes of heterogeneous ice nucleation. For instance, BC particles may be good ice nuclei under contact mode and immersion mode,198 but poor ice nuclei under deposition mode at homogeneous IN temperature.199 Another factor which can affect the ice activation of BC is the mixing state of BC particles. Mixing with OC would modify the surface of BC particles, decreasing the deposition ice nucleation of BC.200
Although there are already several articles reviewing ice nucleation of BC, this work includes recent advances in Table 5 which can complement previous work. Recent work by Nichman et al., Mahart et al., and Zhang et al. applied the pore condensation and freezing (PCF) mechanism to explain the results they obtained on soot (BC), and PCF did fit better with their experiments than classic nucleation theory, which provide insights on future research.226,237,238 PCF was firstly proposed to predict ice formation on porous materials.239 The porous surface of soot or BC makes it suitable to apply PCF to predict the ice nucleation ability of BC.
Table 5 Selected laboratory experiments on ice nucleation of BC. RHi indicates relative humidity to ice, and SSi indicates supersaturation with respect to ice. RHi and SSi are describing the same physical condition. And T indicates temperature
Material |
Size |
Nucleation mode |
T
|
Active fraction density |
RHi/SSi |
Ref. |
Lamp soot |
240 nm |
Deposition |
213–233 K |
N/A |
N/A |
232
|
Soot |
100–250 nm |
Deposition |
216–233 K |
N/A |
135–154% |
251
|
Soot with OC coating |
N/A |
Deposition |
223–226 K |
N/A |
1.22–1.70 |
244
|
Diesel soot |
100–270 nm |
Deposition |
243 K |
N/A |
137% |
240
|
Diesel soot |
120–280 nm |
Deposition |
223 K |
N/A |
143% |
252
|
228 K |
142% |
233 K |
136% |
Carbon black |
800 nm |
PCF |
217–235 K |
0.6 × 106 to 1.2 × 106 cm−2 |
100–150% |
237
|
Soot graphite |
N/A |
Deposition |
228–258 K |
3.7 × 10−2 to 5.2 × 10−2 cm−2 |
1.0–1.35 |
241
|
6.4 × 10−2 cm−2 |
Soot |
400 nm |
PCF |
218 K |
N/A |
100–180% |
238
|
233 K |
Carbon black |
100–400 nm |
PCF |
227–235 K |
N/A |
1.15–1.45 |
226
|
Table 5 also gives clues of why the results of BC ice nucleation are contradictory. In these selected studies, materials chosen by researchers are usually different, such as lamp soot, diesel soot, and carbon black. Sources and combustion conditions strongly affect size, morphology, and chemical constitutions of BC particles, and eventually, results of experiments. Moreover, there is a need to prove the atmospheric relevance of these materials. For example, commercial carbon black particles used by Nichman et al.237 and Zhang et al.226 are manufactured for coating or painting, which are not considered as one of important sources of atmospheric BC, although the advantage of manufactured carbon black is that physicochemical properties of these particles can be controlled.
Consequently, it should be taken into consideration that to what extent, ice nucleation experiments based on these commercial materials or homemade combustion products can suggest actual processes of BC in the atmosphere. As such, one of the challenges in studying ice nucleation of BC is to generate BC particles with controllable physicochemical properties, including size, morphology, coating, and so on. Furthermore, these BC particles should be comparable with atmospheric BC so that conclusions based on these BC particles have atmospheric significance. Some researchers use miniature combustion aerosol standard (miniCAST) to generate BC particles in a stable and reproducible way,231,234,238,240–242 which may indicate a feasible methodology to generate various BC particles. Whereas the selection of specific sizes has been achieved by using differential mobility analyzer (DMA).
Another challenge is how the mixing state will impact ice nucleation ability of BC. Fresh BC particles are nanosized spherules but will grow to aggregate and then agglomerate when aging in the atmosphere.223 During this process, not only will the sizes grow, but also other aerosols, such as organic aerosols and dust, will be internally or externally mixed with BC particles, complicating the physicochemical properties of BC particles.201,223,226,238,243 For example, besides the aforementioned “lensing effect”, the coating of organic carbon on BC particles can change the ice nucleation ability of BC, either enhancing232 or, in most of cases, decreasing ice nucleation of BC.234,240,244
Externally mixing with other aerosols may also alter the surface of BC, affecting hydrophilicity, hygroscopicity and pores or cavities on the surface.223 However, more research shall be done to investigate how these surface properties affect ice nucleation ability of BC. For instance, according to molecular dynamic simulation by Lupi et al., hydrophilicity, simulated by introducing hydroxyl group on the surface of graphite, cannot generally predict ice nucleation ability of BC.245 Yet, a recent study by Xue et al. indicates that the introduction of hydroxyl group on the surface of BC can greatly enhance ice nucleation ability.246 It should be noted that Lupi et al. did simulation on the surface of graphite,245 whereas Xue et al. did their experiments on the surface of graphene,246 which may, to some extent, lead to the discrepancy between their results. Thus, more studies are favored on these properties to draw firm conclusions.
5.4.2. Ice nucleation of brown carbon (BrC).
To our best knowledge, currently, there is no review on the ice nucleation of BrC. Although Laskin et al. and Hems et al. reviewed chemical processes of atmospheric BrC,210,211 ice nucleation ability of BrC and the impacts of aging process on ice nucleation ability were not discussed. Knopf et al. summarized both experimental research and field study on ice nucleation of organic carbon, which covers the components of BrC such as HULIS and PAHs.91 BrC was not discussed specifically in their work, which may further cause discrepancy when modeling the effects of organic carbon on climate. Most of the components of organic carbon are transparent to light and have cooling effect on climate.201 Whereas BrC particles can not only serve as ice nuclei but also absorb radiation, which makes them have more complex effects on the climate,211,212,225 calling for discriminating BrC from other organic carbon when comprehensively evaluating the impacts of organic carbon on climate.
Moreover, it is necessary to bridge the gap between ice nucleation ability and optical properties of BrC. A recent study by Chen et al. reveals that HULIS entities are efficient ice nuclei, comparable with mineral dust and bioaerosols, under mixed-phase cloud conditions, demonstrating the potential impacts of atmospheric BrC in ice nucleation.247 Conjugating optical properties and ice nucleation ability of BrC may facilitate the prediction of ice nucleation ability of BrC by measuring its optical properties. The change of optical properties can reflect the modification of physicochemical properties in molecular level and thus the change of ice nucleation ability.
A major challenge in evaluating ice nucleation of BrC is still the complexity of BrC constitutions discussed above. Knopf et al.91 illustrated that amorphous organic carbon could exist in different phases depending on RH and temperature, from liquid phase to glassy phase.91 The phase of organic carbon governs the mode of ice nucleation that it will undergo in the atmosphere. For example, HULIS, depending on its phase, could serve as immersion IN ins supercooled droplets or as deposition IN under lower supersaturation.201 As such, it is likely that the various components of BrC will serve as efficient INPs like HULIS in mixed-phase clouds, or poor INPs under other conditions. More experiments and field campaigns shall be done to provide more details on ice nucleation ability of BrC and the related conditions. Furthermore, another challenge, as illustrated above, is to discriminate ice nucleation of BrC from that of BC, so that the contribution from BrC to ice nucleation and RF can be assessed.
A significant and challenging task is presently to develop an instrument which can specifically detect BrC in the atmosphere. Such data will be pivotal to assess the contribution of BrC to climate change, considering the proportion of BrC relative to BC in the atmosphere may increase if fossil fuel combustion is constrained. In addition, an open-access database on BrC components would greatly facilitate the research on BrC. Further machine learning methods may be developed on the database to discriminate new components of BrC, in near future.
6. Snow management in cities in the age of increasing emerging contaminants
Snow removal is important municipal management for cold regions which receive a large amount of snow every year. Table 6 summarizes the situations in selected areas in the world, including annual snowfall and the amount of road salts used for snow removal. In urban areas, accumulated snow on pavements and roads will hinder pedestrians' walking and transportation. When ice forms on the ground, it will pose an even serious threat on vehicles and pedestrians. Furthermore, snowpack in urban areas will take part in several heterogeneous processes shown in Fig. 8, causing accumulation, transformation, and transport of particles and metals.
Table 6 Selected aeras which receive snowfall during a year
Location |
Snowfall |
Annual budget for snow removal |
Amount of road salts |
Ref. |
Montreal Canada |
235.6 cm |
166 m CAD |
More than 200 kilotons |
253 and 260 |
Calgary Canada |
103.6 cm |
40.9 m CAD |
30 to 40 kilotons |
254, 261 and 268 |
Helsinki Finland |
74.0 cm |
24 m EURO |
80 to 100 tons |
255, 262, 264 and 269 |
Oslo Norway |
87.2 cm |
5.3 m EURO |
250 kilotons (nationwide) |
256, 263 and 270 |
Stockholm Sweden |
99.8 cm |
190 m SEK |
300 kilotons (nationwide) |
257, 264 and 276 |
Sapporo Japan |
437 cm |
190 m USD |
N/A |
258 and 265 |
Ohio US |
N/A |
65 m USD |
600 kilotons |
259, 271 and 281 |
Illinois US |
100.0 cm |
100 m USD |
522 kilotons |
259, 266, 268 and 272 |
New Jersey US |
N/A |
92.5 m USD |
375 kilotons |
273
|
New York city US |
93.7 cm |
92.3 m USD |
About 407 kilotons |
259, 267 and 274 |
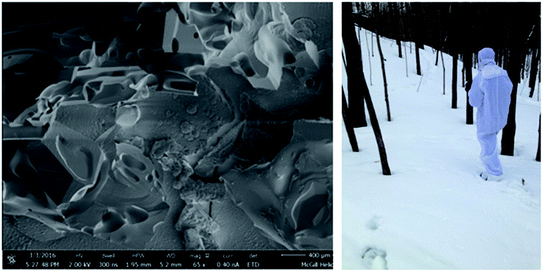 |
| Fig. 7 Electron microscopy of frozen snow with embedded particles using high resolution Leica EM VCT100 cryo-stage on the Dual Beam (FIB-SEM; courtesy of McGill microscopy facilities). The person in a clean suit is Rodrigo Rangel who was collecting snow samples to be analyzed in Montreal, QC, Canada. | |
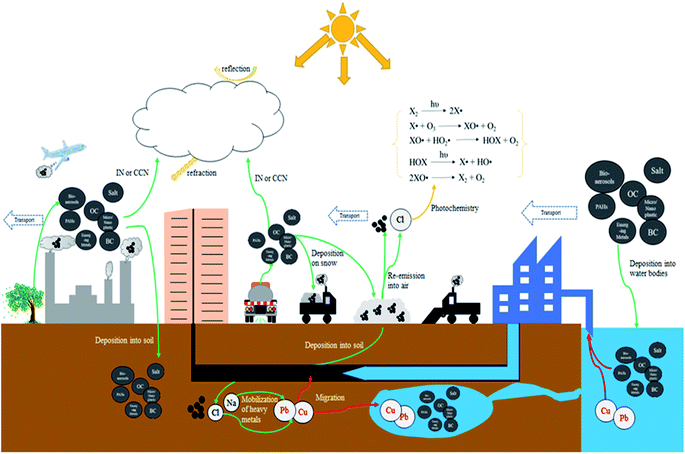 |
| Fig. 8 Air-snow particle interaction in urban areas. | |
There are several methods to do snow removal worldwide, depending on local situations and municipal budgets. One of the most prevalent methods is using snowplows that can efficiently remove snow and ice after snowfalls. However, high labor costs and high rentals for snowplows make it necessary to combine other methods to lower the expenses. Another prevalent but cost-effective method is to spread deicers, such as NaCl, MgCl2, and CaCl2.
Table 7 shows several common deicers, their costs, and their lowest effective temperatures. NaCl is the most widely used deicing materials among these deicers because of its good availability and low cost. However, there are increasing concerns about using NaCl.279–283 First, using NaCl will increase the salinity of fresh water when snow or ice melts, threatening the biota in fresh waters. Secondly, soil which absorbs snowmelt with high salinity cannot support vegetation growth. Li et al. found that shrubs were severely damaged, due to deicing salt pollution of the soils, whereas arbors were less vulnerable.284 More importantly, extra NaCl entering ecosystems will increase the mobilization of heavy metals such as Cu, Cd, and Pb (Fig. 8).
Table 7 The costs and lowest effective temperature of common de-icers. Temperatures were reported in Fahrenheit and converted into Celsius in this table. It should be noted that potassium acetate (Kac.) is usually used as solution with 50% concentration
Deicer |
Cost |
Lowest effective temperature (°C) |
Ref. |
NaCl |
$26 per ton |
−9.4 |
275 and 277 |
MgCl2 |
$95 per ton |
−23.3 |
275
|
CaCl2 |
$294 per ton |
−28.9 |
275 and 277 |
Calcium magnesium acetate (CMA) |
$670 per ton |
−6.7 |
275 and 277 |
Potassium acetate (kac.) |
$4.50 to $5.12 per gallon |
−26.1 |
275, 277 and 278 |
Mahrosh et al. reported that the application of road salts (NaCl) caused an increment of Cu in freshwater systems. The high concentration of NaCl mixed with Cu could lead to less egg survival, delayed hatching, and high percentage of deformities of Atlantic salmon.285 Whereas Cl− may facilitate the dissolution of Pb in soils. And when water with a high concentration of Cl− enters municipal water supplies, the water plumbing may undergo dezincification and galvanic corrosion, thinning pipe walls and releasing Pb into drinking water, which severely threatens human health of residents.282
The atmosphere may also be affected from salt addition to snow in the cities. High concentrations of chloride found in urban cities in winter period, and the concentration of photoactive chlorine in the atmosphere showed a positive correlation with the concentration of chloride.18
Economic costs of de-icing: Snowmelt with high salinity causes corrosion of infrastructures and vehicles, bringing extra economic costs of repair and maintenance of infrastructures and vehicles. For instance, Vitaliano et al., estimated that the damages caused by road salts in the US cost at least $615 per ton for bridge repairs and highway maintenance. And the estimation on vehicular corrosion was $113 per ton.286 These costs may have been increasing in the past three decades, considering the expansion of the transportation network and the increment of vehicle ownerships.202
The current threshold recommended by USEPA for NaCl in the environment has been questioned and needs to be updated, considering the usage of road salts has been increasing in the past decades.203 Beside NaCl, there are also some other de-icers that are used in the world, such as calcium magnesium acetate, urea, beet juice, salt brine, and abrasives.204 However, due to the problem of costs and effectiveness, these de-icers are not used as widely as NaCl.
Another method for snow removal is to use anti-icers before snow, such as salt brine. And in aviation, organic fluids like ethylene glycol are usually applied on the surface of airplanes for anti-freezing, but they are seldomly used in urban areas because of the cost and impacts on the environment.
Although the side effects of using NaCl have been discussed above, it is still the most common deicer in the world. At present, there is no cost-effective and environmental-friendly alternative for NaCl.282 Thus, most efforts in snow management are focused on optimizing snow removal strategies to lower the cost of snowplows and reduce the usage of deicers.282,287 However, it should be noted that, as discussed in Sections 2 and 4, snowpack on the roads can serve as a good reservoir for vehicular exhausts and emerging contaminants. Even after snow removal by snowplows, it is necessary to treat those snow properly. Dumping snow into local rivers or streams would still introduce emerging contaminants and road salts to local water systems, leading to health concerns for residents. It might be a good idea to store snow in deposit sites built with impermeable materials and then treat snow or snowmelt to make sure it is clean and safe enough to enter the city's drainage or rivers.
7. Future directions
We need to better understand the influence of snow and ice surfaces on the processes and life cycle of air pollutants, with field work, laboratory research and modelling studies. We specifically require evaluating the chemical, physical and biological processes occurring on snow and ice surfaces from urban, rural, industrial, as well as remote sites and synthesize an in-depth understanding of the biogeochemical cycling and toxicological effects of snow-borne particles on living ecosystems as well as human health impacts.
Future research direction should address the existing challenges, namely:
• There is a need to better characterize the chemical composition, speciation, and abundance of organic volatile, semi-volatile compounds (including bio-organic), metal pollutants, and particulate matter in snow samples, in urban, rural, industrial, and remote areas. To do so, improvements in analytical methods and instruments are required.
• To advance the understanding of the impact of ice nucleation in snow/ice processes, we recommend: (a) to develop instruments that can generate various BC particles in a stable and reproducible way, like miniCAST mentioned above, so that results from different research groups are more comparable; (b) more studies shall be done on mixing states of BC by using various materials, especially organic carbon, to figure out how BC will interact with these materials and what sort of materials can enhance the ice nucleation ability of BC; (c) more characterizations on BC particles used in experiments shall be done to provide details and impacts of physicochemical properties of BC; (d) more research on the size distribution of BC particles are recommended, since size-resolved information of BC particles are significant for more precise modelling study and predictions.
• There are a couple of technologies which have a potential to contribute to future snow research. The first one is cryo-stage high-resolution microscopy that allows snow samples at their original temperature to be studied. Fig. 7 depicts a snow sample taken in an urban park (Mont Royal, Montreal, Canada) and analyzed with such a unit (Leica EM VCT100 cryo-stage on the DualBea). This technique may obtain insights into structure particle distribution and morphology within frozen snow. Another promising technique is Digital Inline Holography Microscope (DIHM),205 which has been used for atmospheric research205 and marine research.206 Both these technologies hold promise for future physicochemical snow research, particularly with the elements of robotics and automation.
• There is evidence of reactive urban grime, which refers to films deposited on urban surfaces, including snowy cities.207 Potential biological effects on film extracts, using zebrafish embryo essays, evaluated a dose-dependent syndrome of abnormalities including cardiovascular, hematopoietic, and behavioral defects.207 These films are composed of a complex mixture of organic and inorganic compounds, containing significant levels of inorganic nitrate and sulfate.14,16,208 Yet, upon photolysis, they release air pollutants such as NOx (NO + NO2) and HONO in urban areas. There is not much known about the interactions of snow and urban grime, yet some components of urban grime such as PAHs have been observed in snow. Hence further research in the domain is recommended.
• We must answer fundamental questions such as: What is the fate of snow-borne compounds after photochemical aging? Do the aging processes change the emission fluxes of chemical compounds to the gas phase? How do chemical compounds of anthropogenic origin change the distribution and composition of nanoparticles in the air and snow at the snow grain level? What types of interactions do snow-borne anthropogenic materials have with the atmosphere, water, and soil? Can we use snow as a probe for new emerging contaminants, as some contaminants have been shown to be more concentrated in snow than rain? What is the life cycle of emerging contaminants in snow? What are the effects of the cycles of melt, precipitation, and freezing on chemical compositions and distribution of emerging contaminants?
Conflicts of interest
There are no conflicts to declare.
Acknowledgements
We would like to thank the Natural Science and Engineering Research Council of Canada for financial support, and PRIMA Quebec. We thank Mr Ryan Hall for proofreading. We are very grateful to Prof. Paul Shepson, Prof. Dominé and Prof. Grannas for valuable suggestions.
References
- R. B. Rangel-Alvarado, Y. Nazarenko and P. A. Ariya, Snow-borne nanosized particles: abundance, distribution, composition, and significance in ice nucleation processes, J. Geophys. Res.: Atmos., 2015, 120(22), 774 Search PubMed.
- P. Hou, S. Wu, J. L. McCarty and Y. Gao, Sensitivity of atmospheric aerosol scavenging to precipitation intensity and frequency in the context of global climate change, Atmos. Chem. Phys., 2018, 18(11), 8173 CrossRef CAS.
-
M. Ananicheva, T. V. Callaghan, D. Dahl-Jensen, S. Gerland, M. A. Granskog, G. K. Hovelsrud, M. Johansson, J. R. Key, W. N. Meier and M. S. Olsen, Snow, water, ice and permafrost in the Arctic (SWIPA): climate change and the cryosphere, Arctic Monitoring and Assessment Programme (AMAP), Oslo, 2011, vol. 1, p. 550 Search PubMed.
-
Intergovernmental Panel on Climate Change Summary for policymakers of IPCC special report on global warming of 1.5 °C approved by governments, https://www.ipcc.ch/2018/10/08/summary-for-policymakers-of-ipcc-special-report-on-global-warming-of-1-5c-approved-by-governments/, accessed 2020-10-14 Search PubMed.
- M. Tang, D. J. Cziczo and V. H. Grassian, Interactions of Water with Mineral Dust Aerosol: Water Adsorption, Hygroscopicity, Cloud Condensation, and Ice Nucleation, Chem. Rev., 2016, 116(7), 4205–4259 CrossRef CAS PubMed.
-
World Health Organization, 7 million premature deaths annually linked to air pollution, World Health Organization, Geneva, Switzerland, 2014 Search PubMed.
-
C. D. Ahrens, P. L. Jackson and C. E. O. Jackson, Meteorology today: an introduction to weather, climate and the environment, Cengage Learning, 10th edn, 2012, vol. 1, p. 640 Search PubMed.
-
NASA Global Rural-Urban Mapping Project (GRUMP), v1, https://sedac.ciesin.columbia.edu/data/collection/grump-v1, accessed 2020-11-24 Search PubMed.
-
K. Seto, S. Dhakal, A. Bigio, H. Blanco, G. Delgado, D. Dewar, L. Huang, A. Inaba, A. Kansal and S. Lwasa, Human settlements, infrastructure and spatial planning. Climate change 2014: Mitigation of climate change, in Contribution of Working Group III to the Fifth Assessment Report of the Intergovernmental Panel on Climate Change, Cambridge University Press, Cambridge, United Kingdom and New York, NY, USA, 2014, vol. 1, pp. 923–1000 Search PubMed.
-
R. K. Pachauri, M. R. Allen, V. R. Barros, J. Broome, W. Cramer, R. Christ, J. A. Church, L. Clarke, Q. Dahe and P. Dasgupta, Climate change 2014: synthesis report. Contribution of Working Groups I, II and III to the fifth assessment report of the Intergovernmental Panel on Climate Change, IPCC, Geneva, Switzerland, 2014, vol. 1, p. 151 Search PubMed.
- D. Satterthwaite, Cities' contribution to global warming: notes on the allocation of greenhouse gas emissions, Environ. Urban., 2008, 20(2), 539–549 CrossRef.
-
United Nations 68% of the world population projected to live in urban areas by 2050, says UN, https://www.un.org/development/desa/en/news/population/2018-revision-of-world-urbanization-prospects.html, accessed 2020-11-23 Search PubMed.
- P. A. Ariya, F. Domine, G. Kos, M. Amyot, V. Côté, H. Vali, T. Lauzier, W. Kuhs, K. Techmer and T. Heinrichs, Snow – a photobiochemical exchange platform for volatile and semi-volatile organic compounds with the atmosphere, Environ. Chem., 2011, 8(1), 62–73 CrossRef CAS.
- Y. Nazarenko, S. Fournier, U. Kurien, R. B. Rangel-Alvarado, O. Nepotchatykh, P. Seers and P. A. Ariya, Role of snow in the fate of gaseous and particulate exhaust pollutants from gasoline-powered vehicles, Environ. Pollut., 2017, 223, 665–675 CrossRef CAS PubMed.
- D. Pal, A. Dastoor and P. A. Ariya, Aerosols in an urban cold climate: physical and chemical characteristics of nanoparticles, Urban Clim., 2020, 34, 100713 CrossRef.
- Y. Nazarenko, U. Kurien, O. Nepotchatykh, R. B. Rangel-Alvarado and P. A. Ariya, Role of snow and cold environment in the fate and effects of nanoparticles and select organic pollutants from gasoline engine exhaust, Environ. Sci.: Processes
Impacts, 2016, 18(2), 190–199 RSC.
- S. M. McNamara, K. R. Kolesar, S. Wang, R. M. Kirpes, N. W. May, M. J. Gunsch, R. D. Cook, J. D. Fuentes, R. S. Hornbrook and E. C. Apel, Observation of Road Salt Aerosol Driving Inland Wintertime Atmospheric Chlorine Chemistry, ACS Cent. Sci., 2020, 6(5), 684–694 CrossRef CAS PubMed.
- R. Hall, O. Nepotchatykh, E. Nepotchatykh and P. A. Ariya, Anthropogenic Photolabile Chlorine in the Cold-Climate City of Montreal, Atmosphere, 2020, 11(8), 812 CrossRef CAS.
-
R. LeGates and A. Pamuk, Space, Culture, and Urban Policy, https://online.sfsu.edu/nsfgis/map_gallery/world_cities.htm, accessed 2020-12-05 Search PubMed.
-
Earth Observatory Snow Cover, https://earthobservatory.nasa.gov/global-maps/MOD10C1_M_SNOW, accessed 2020-12-05 Search PubMed.
- M. Dragosics, O. Meinander, T. Jónsdóttír, T. Dürig, G. De Leeuw, F. Pálsson, P. Dagsson-Waldhauserová and T. Thorsteinsson, Insulation effects of Icelandic dust and volcanic ash on snow and ice, Arabian J. Geosci., 2016, 9(2), 126 CrossRef.
- A. L. Khan, H. Dierssen, J. P. Schwarz, C. Schmitt, A. Chlus, M. Hermanson, T. H. Painter and D. M. McKnight, Impacts of coal dust from an active mine on the spectral reflectance of Arctic surface snow in Svalbard, Norway, J. Geophys. Res.: Atmos., 2017, 122(3), 1767–1778 CrossRef.
- A. J. Poulain, E. Garcia, M. Amyot, P. G. C. Campbell, F. Raofie and P. A. Ariya, Biological and Chemical Redox Transformations of Mercury in Fresh and Salt Waters of the High Arctic during Spring and Summer, Environ. Sci. Technol., 2007, 41(6), 1883–1888 CrossRef CAS PubMed.
- A. J. Poulain, S. M. Ni Chadhain, P. A. Ariya, M. Amyot, E. Garcia, P. G. Campbell, G. J. Zylstra and T. Barkay, Potential for mercury reduction by microbes in the high arctic, Appl. Environ. Microbiol., 2007, 73(7), 2230–2238 CrossRef CAS PubMed.
- S. J. Doherty, C. Dang, D. A. Hegg, R. Zhang and S. G. Warren, Black carbon and other light-absorbing particles in snow of central North America, J. Geophys. Res.: Atmos., 2014, 119(22), 12807–12831 CAS.
- R. Mortazavi, S. Attiya and P. Ariya, Arctic microbial and next-generation sequencing approach for bacteria in snow and frost flowers: selected identification, abundance and freezing nucleation, Atmos. Chem. Phys., 2015, 15(11), 6183–6204 CrossRef CAS.
- R. Mortazavi, S. Attiya and P. A. Ariya, Diversity of metals and metal-interactive bacterial populations in different types of Arctic snow and frost flowers: implications on snow freeze-melt processes in a changing climate, Sci. Total Environ., 2019, 690, 277–289 CrossRef PubMed.
-
P. A. Ariya, G. Kos, R. Mortazavi, E. D. Hudson, V. Kanthasamy, N. Eltouny, J. Sun and C. Wilde, Bio-organic materials in the atmosphere and snow: measurement and characterization, in Atmospheric and Aerosol Chemistry, Springer, 2014, pp. 145–199 Search PubMed.
- P. A. Ariya, H. Niki, G. W. Harris, K. G. Anlauf and D. E. Worthy, Polar sunrise experiment 1995: hydrocarbon measurements and tropospheric Cl and Br-atoms chemistry, Atmos. Environ., 1999, 33(6), 931–938 CrossRef CAS.
- J. Comte, A. I. Culley, C. Lovejoy and W. F. Vincent, Microbial connectivity and sorting in a High Arctic watershed, ISME J., 2018, 12(12), 2988–3000 CrossRef CAS PubMed.
-
The European Space Agency Snow grain size – it matters, https://www.esa.int/Applications/Observing_the_Earth/Copernicus/Sentinel-3/Snow_grain_size_it_matters, accessed 2020-11-24 Search PubMed.
- T. Harding, A. D. Jungblut, C. Lovejoy and W. F. Vincent, Microbes in high arctic snow and implications
for the cold biosphere, Appl. Environ. Microbiol., 2011, 77(10), 3234–3243 CrossRef CAS PubMed.
- R. Antony, A. Sanyal, N. Kapse, P. K. Dhakephalkar, M. Thamban and S. Nair, Microbial communities associated with Antarctic snow pack and their biogeochemical implications, Microbiol. Res., 2016, 192, 192–202 CrossRef CAS PubMed.
- R. Antony, K. Mahalinganathan, M. Thamban and S. Nair, Organic carbon in Antarctic snow: spatial trends and possible sources, Environ. Sci. Technol., 2011, 45(23), 9944–9950 CrossRef CAS PubMed.
- A. Hodson, A. M. Anesio, M. Tranter, A. Fountain, M. Osborn, J. Priscu, J. Laybourn-Parry and B. Sattler, Glacial ecosystems, Ecol. Monogr., 2008, 78(1), 41–67 CrossRef.
- V. Cote, G. Kos, R. Mortazavi and P. A. Ariya, Microbial and “de novo” transformation of dicarboxylic acids by three airborne fungi, Sci. Total Environ., 2008, 390(2–3), 530–537 CrossRef CAS PubMed.
- R. B. Rangel-Alvarado, C. E. Willis, J. L. Kirk, V. L. St Louis, M. Amyot, D. Bélanger and P. A. Ariya, Athabasca oil sands region snow contains efficient micron and nano-sized ice nucleating particles, Environ. Pollut., 2019, 252, 289–295 CrossRef CAS PubMed.
- T. Walker, S. Young, P. Crittenden and H. Zhang, Anthropogenic metal enrichment of snow and soil in north-eastern European Russia, Environ. Pollut., 2003, 121(1), 11–21 CrossRef CAS PubMed.
- E. N. Kelly, D. W. Schindler, P. V. Hodson, J. W. Short, R. Radmanovich and C. C. Nielsen, Oil sands development contributes elements toxic at low concentrations to the Athabasca River and its tributaries, Proc. Natl. Acad. Sci. U. S. A., 2010, 107(37), 16178–16183 CrossRef CAS PubMed.
- E. J. Stewart, S. E. Howell, D. Draper, J. Yackel and A. Tivy, Sea ice in Canada's Arctic: Implications for cruise tourism, Arctic, 2007, 370–380 Search PubMed.
- M. V. Vasić, A. Mihailović, U. Kozmidis-Luburić, T. Nemes, J. Ninkov, T. Zeremski-Škorić and B. Antić, Metal contamination of short-term snow cover near urban crossroads: correlation analysis of metal content and fine particles distribution, Chemosphere, 2012, 86(6), 585–592 CrossRef PubMed.
- T. Meyer, Y. D. Lei and F. Wania, Transport of polycyclic aromatic hydrocarbons and pesticides during snowmelt within an urban watershed, Water Res., 2011, 45(3), 1147–1156 CrossRef CAS PubMed.
- M. F. Rahim, D. Pal and P. A. Ariya, Physicochemical studies of aerosols at Montreal Trudeau Airport: the importance of airborne nanoparticles containing metal contaminants, Environ. Pollut., 2019, 246, 734–744 CrossRef CAS PubMed.
- M. Bergmann, S. Mützel, S. Primpke, M. Tekman, J. Trachsel and G. Gerdts, White and wonderful? Microplastics prevail in snow from the Alps to the Arctic, Sci. Adv., 2019, 5, 1157 CrossRef PubMed.
- H. Bouwmeester, P. C. Hollman and R. J. Peters, Potential health impact of environmentally released micro-and nanoplastics in the human food production chain: experiences from nanotoxicology, Environ. Sci. Technol., 2015, 49(15), 8932–8947 CrossRef CAS PubMed.
- R. Sussarellu, M. Suquet, Y. Thomas, C. Lambert, C. Fabioux, M. E. J. Pernet, N. Le Goïc, V. Quillien, C. Mingant and Y. Epelboin, Oyster reproduction is affected by exposure to polystyrene microplastics, Proc. Natl. Acad. Sci. U. S. A., 2016, 113(9), 2430–2435 CrossRef CAS PubMed.
-
Z. Wang, N. Saadé and P. A. Ariya, Observation of nano and microplastic in fresh snow, Submitted, 2020 Search PubMed.
- K. D. Cox, G. A. Covernton, H. L. Davies, J. F. Dower, F. Juanes and S. E. Dudas, Human consumption of microplastics, Environ. Sci. Technol., 2019, 53(12), 7068–7074 CrossRef CAS PubMed.
- H. I. Abdel-Shafy and M. S. Mansour, A review on polycyclic aromatic hydrocarbons: source, environmental impact, effect on human health and remediation, Egypt. J. Pet., 2016, 25(1), 107–123 CrossRef.
- J. Liggio, S.-M. Li, K. Hayden, Y. M. Taha, C. Stroud, A. Darlington, B. D. Drollette, M. Gordon, P. Lee and P. Liu, Oil sands operations as a large source of secondary organic aerosols, Nature, 2016, 534(7605), 91 CrossRef CAS PubMed.
- A. Parajulee and F. Wania, Evaluating officially reported polycyclic aromatic hydrocarbon emissions in the Athabasca oil sands region with a multimedia fate model, Proc. Natl. Acad. Sci. U. S. A., 2014, 111(9), 3344–3349 CrossRef CAS PubMed.
- J. Kurek, J. L. Kirk, D. C. Muir, X. Wang, M. S. Evans and J. P. Smol, Legacy of a half century of Athabasca oil sands development recorded by lake ecosystems, Proc. Natl. Acad. Sci. U. S. A., 2013, 110(5), 1761–1766 CrossRef CAS PubMed.
- J. L. Kirk, D. C. Muir, A. Gleason, X. Wang, G. Lawson, R. A. Frank, I. Lehnherr and F. Wrona, Atmospheric deposition of mercury and methylmercury to landscapes and waterbodies of the Athabasca oil sands region, Environ. Sci. Technol., 2014, 48(13), 7374–7383 CrossRef CAS PubMed.
-
U.S.EPA, Environmental Protection Agency. Toxic and Priority Pollutants, 1963, https://pubs.usgs.gov/circ/1953/0285/report.pdf Search PubMed.
- F. Dominé and P. B. Shepson, Air-snow interactions and atmospheric chemistry, Science, 2002, 297(5586), 1506–1510 CrossRef PubMed.
- A. Grannas, A. E. Jones, J. Dibb, M. Ammann, C. Anastasio, H. Beine, M. Bergin, J. Bottenheim, C. Boxe and G. Carver, An overview of snow photochemistry: evidence, mechanisms and impacts, Atmos. Chem. Phys., 2007, 7(2), 4165–4283 Search PubMed.
- V. McNeill, A. Grannas, J. Abbatt, M. Ammann, P. Ariya, T. Bartels-Rausch, F. Domine, D. Donaldson, M. I. Guzman and D. Heger, Organics in environmental ices: sources, chemistry, and impacts, Atmos. Chem. Phys., 2012, 12(20), 9653–9678 CrossRef CAS.
- A. Steffen, T. Douglas, M. Amyot, P. Ariya, K. Aspmo, T. Berg, J. Bottenheim, S. Brooks, F. Cobbett and A. Dastoor, A synthesis of atmospheric mercury depletion event chemistry in the atmosphere and snow, Atmos. Chem. Phys., 2008, 8(6), 1445–1482 CrossRef CAS.
- W. R. Simpson, R. Von Glasow, K. Riedel, P. Anderson, P. Ariya, J. Bottenheim, J. Burrows, L. Carpenter, U. Frieß and M. E. Goodsite, Halogens and their role in polar boundary-layer ozone depletion, Atmos. Chem. Phys., 2007, 7, 4285–4403 Search PubMed.
- T. Kinase, K. Adachi, N. Oshima, K. Goto-Azuma, Y. Ogawa-Tsukagawa, Y. Kondo, N. Moteki, S. Ohata, T. Mori and M. Hayashi, Concentrations and size distributions of black carbon in the surface snow of Eastern Antarctica in 2011, J. Geophys. Res.: Atmos., 2020, 125(1), e2019JD030737 CAS.
- P. Kukučka, G. Lammel, A. Dvorská, J. Klánová, A. Möller and E. Fries, Contamination of Antarctic snow by polycyclic aromatic hydrocarbons dominated by combustion sources in the polar region, Environ. Chem., 2010, 7(6), 504–513 CrossRef.
- A. R. Aves, L. E. Revell, S. Gaw, H. Ruffell, A. Schuddeboom, N. E. Wotherspoon, M. LaRue and A. J. McDonald, First evidence of microplastics in Antarctic snow, Cryosphere, 2022, 16(6), 2127–2145 CrossRef.
- C. Chang, C. Han, Y. Han, S. D. Hur, S. Lee, H. Motoyama, S. Hou and S. Hong, Persistent Pb pollution in central East Antarctic snow: A retrospective assessment of sources and control policy implications, Environ. Sci. Technol., 2016, 50(22), 12138–12145 CrossRef CAS PubMed.
- G. Carrera, P. Fernández, R. M. Vilanova and J. O. Grimalt, Persistent organic pollutants in snow from European high mountain areas, Atmos. Environ., 2001, 35(2), 245–254 CrossRef CAS.
- A. Prieto, S. Schrader and M. Moeder, Determination of organic priority pollutants and emerging compounds in wastewater and snow samples using multiresidue protocols on the basis of microextraction by packed sorbents coupled to large volume injection gas chromatography–mass spectrometry analysis, J. Chromatogr. A, 2010, 1217(38), 6002–6011 CrossRef CAS PubMed.
- K. Kawamura, Identification of C2–C10. omega.-oxocarboxylic acids, pyruvic acid, and C2–C3. alpha.-dicarbonyls in wet precipitation and aerosol samples by capillary GC and GC/MS, Anal. Chem., 1993, 65(23), 3505–3511 CrossRef CAS.
- C. Telloli, Metal concentrations in snow samples in an urban area in the Po Valley, Int. J. Geosci., 2014, 5(10), 1116 CrossRef.
- A. Bazzano, K. Latruwe, M. Grotti and F. Vanhaecke, Lead isotopic analysis of Antarctic snow using multi-collector ICP-mass spectrometry, J. Anal. At. Spectrom., 2015, 30(6), 1322–1328 RSC.
- C. Barbante, T. Bellomi, G. Mezzadri, P. Cescon, G. Scarponi, C. Morel, S. Jay, K. Van de Velde, C. Ferrari and C. F. Boutron, Direct determination of heavy metals at picogram per gram levels in Greenland and Antarctic snow by double focusing inductively coupled plasma mass spectrometry, J. Anal. At. Spectrom., 1997, 12(9), 925–931 RSC.
- O. Akba, E. Kilinc, I. Aydin, S. Erdogan, F. Aydin, M. Z. Duz and C. Hamamci, Major and trace element contamination of short-term snow cover during and after a dust storm and analysis by ICP-OES, Inflammation, 2013, 10, 11 CrossRef PubMed.
-
J. Pilecka, I. Grinfelde, K. Valujeva, I. Straupe and O. Purmalis, Heavy metal concentration and distribution of snow and Lichea samples in urban area: Case study of Jelgava, International Multidisciplinary Scientific GeoConference: SGEM, 2017, vol. 17, pp. 459–466 Search PubMed.
- J. L. Axson, J. M. Creamean, A. L. Bondy, S. S. Capracotta, K. Y. Warner and A. P. Ault, An in situ method for sizing insoluble residues in precipitation and other aqueous samples, Aerosol Sci. Technol., 2015, 49(1), 24–34 CrossRef CAS PubMed.
- Y. Nazarenko, R. B. Rangel-Alvarado, G. Kos, U. Kurien and P. A. Ariya, Novel aerosol analysis approach for characterization of nanoparticulate matter in snow, Environ. Sci. Pollut. Res., 2017, 24(5), 4480–4493 CrossRef CAS PubMed.
- W. Rosenthal, J. Saleta and J. Dozier, Scanning electron microscopy of impurity structures in snow, Cold Reg. Sci. Technol., 2007, 47(1–2), 80–89 CrossRef.
- E. Drab, A. Gaudichet, J. Jaffrezo and J. Colin, Mineral particles content in recent snow at Summit (Greenland), Atmos. Environ., 2002, 36(34), 5365–5376 CrossRef CAS.
- G. G. Leppard, A. Heissenberger and G. J. Herndl, Ultrastructure of marine snow. I. Transmission electron microscopy methodology, Mar. Ecol.: Prog. Ser., 1996, 135, 289–298 CrossRef.
- J. Li and K. Osada, Water-insoluble particles in spring snow at Mt. Tateyama, Japan: Characteristics of the shape factors and size distribution in relation with their origin and transportation, J. Meteorol. Soc. Jpn., 2007, 85(2), 137–149 CrossRef.
- S. D. Janssens, S. Koizumi and E. Fried, Behavior of self-propelled acetone droplets in a Leidenfrost state on liquid substrates, Phys. Fluids, 2017, 29(3), 032103 CrossRef.
- G. Kos, V. Kanthasami, N. Adechina and P. A. Ariya, Volatile organic compounds in Arctic snow: concentrations and implications for atmospheric processes, Environ. Sci.: Processes Impacts, 2014, 16(11), 2592–2603 RSC.
- G. Kos and P. A. Ariya, Determination of a wide range of volatile and semivolatile organic compounds in snow by use of solid-phase micro-extraction (SPME), Anal. Bioanal. Chem., 2006, 385(1), 57–66 CrossRef CAS PubMed.
- E. Fries, K. Sieg, W. Püttmann, W. Jaeschke, R. Winterhalter, J. Williams and G. K. Moortgat, Benzene, alkylated benzenes, chlorinated hydrocarbons and monoterpenes in snow/ice at Jungfraujoch (46.6 N, 8.0 E) during CLACE 4 and 5, Sci. Total Environ., 2008, 391(2–3), 269–277 CrossRef CAS PubMed.
- J. P. Bower, E. Hood and L. A. Hoferkamp, Major solutes, metals, and alkylated aromatic compounds in high-latitude maritime snowpacks near the trans-Alaska pipeline terminal, Valdez, Alaska, Environ. Res. Lett., 2008, 3(4), 045010 CrossRef.
- J. Czuczwa, C. Leuenberger and W. Giger, Seasonal and temporal changes of organic compounds in rain and snow, Atmos. Environ., 1988, 22(5), 907–916 CrossRef CAS.
- B. Herbert, C. Halsall, L. Fitzpatrick, S. Villa, K. Jones and G. Thomas, Use and validation of novel snow samplers for hydrophobic, semi-volatile organic compounds (SVOCs), Chemosphere, 2004, 56(3), 227–235 CrossRef CAS PubMed.
- I. Faengmark, B. van Bavel, S. Marklund, B. Stroemberg, N. Berge and C. Rappe, Influence of combustion parameters on the formation of polychlorinated dibenzo-p-dioxins, dibenzofurans, benzenes, and biphenyls and polyaromatic hydrocarbons in a pilot incinerator, Environ. Sci. Technol., 1993, 27(8), 1602–1610 CrossRef CAS.
- T. C. Bond, D. G. Streets, K. F. Yarber, S. M. Nelson, J. H. Woo and Z. Klimont, A technology-based global inventory of black and organic carbon emissions from combustion, J. Geophys. Res.: Atmos., 2004, 109, D14203 CrossRef.
-
H. Choi, R. Harrison, H. Komulainen and J. M. D. Saborit, Polycyclic aromatic hydrocarbons, in WHO guidelines for indoor air quality: selected pollutants, World Health Organization, 2010, vol. 1, pp. 289–346 Search PubMed.
- A. Abramova, S. Chernianskii, N. Marchenko and E. Terskaya, Distribution of polycyclic aromatic hydrocarbons in snow particulates around Longyearbyen and Barentsburg settlements, Spitsbergen, Polar Rec., 2016, 52(6), 645–659 CrossRef.
- S. Cui, Z. Song, L. Zhang, Z. Zhang, R. Hough, Q. Fu, L. An, Z. Shen, Y.-F. Li and D. Liu, Polycyclic aromatic hydrocarbons in fresh snow in the city of Harbin in northeast China, Atmos. Environ., 2019, 215, 116915 CrossRef CAS.
- T. Harner, C. Rauert, D. Muir, J. K. Schuster, Y.-M. Hsu, L. Zhang, G. Marson, J. G. Watson, J. Ahad and S. Cho, Air synthesis review: polycyclic aromatic compounds in the oil sands region, Environ. Rev., 2018, 26(4), 430–468 CrossRef CAS.
- A. Berthiaume, E. Galarneau and G. Marson, Polycyclic aromatic compounds (PACs) in the Canadian environment: Sources and emissions, Environ. Pollut., 2021, 269, 116008 CrossRef CAS PubMed.
-
N. Glozier, D. Donald, R. Crosley and D. Halliwell, Wood Buffalo National Park Water Quality: Status and trends from 1989–2006 in three major rivers; Athabasca, Peace and Slave, Environment Canada, Saskatoon, SK, 2009 Search PubMed.
- J. K. Schuster, T. Harner, K. Su, C. Mihele and A. Eng, First results from the oil sands passive air monitoring network for polycyclic aromatic compounds, Environ. Sci. Technol., 2015, 49(5), 2991–2998 CrossRef CAS PubMed.
-
O. S. M. Program, Oil Sands Monitoring Program: Annual Report for 2018–19, Retrieved from: https://open.alberta.ca/publications/2562-9182; 2019 Search PubMed.
- J. T. Andersson and C. Achten, Time to say goodbye to the 16 EPA PAHs? Toward an up-to-date use of PACs for environmental purposes, Polycyclic Aromat. Compd., 2015, 35(2–4), 330–354 CrossRef CAS PubMed.
- S. Birks, S. Cho, E. Taylor, Y. Yi and J. Gibson, Characterizing the PAHs in surface waters and snow in the Athabasca region: Implications for identifying hydrological pathways of atmospheric deposition, Sci. Total Environ., 2017, 603, 570–583 CrossRef PubMed.
- D. A. Knopf, P. A. Alpert and B. Wang, The role of organic aerosol in atmospheric ice nucleation: a review, ACS Earth Space Chem., 2018, 2(3), 168–202 CrossRef CAS.
- K. Tsigaridis, J. Lathiere, M. Kanakidou and D. Hauglustaine, Naturally driven variability in the global secondary organic aerosol over a decade, Atmos. Chem. Phys., 2005, 5(7), 1891–1904 CrossRef CAS.
- S. H. Chung and J. H. Seinfeld, Global distribution and climate forcing of carbonaceous aerosols, J. Geophys. Res.: Atmos., 2002, 107(D19), 1–33 CrossRef.
- J. D. Blando and B. J. Turpin, Secondary organic aerosol formation in cloud and fog droplets: a literature evaluation of plausibility, Atmos. Environ., 2000, 34(10), 1623–1632 CrossRef CAS.
- B. Wang, A. T. Lambe, P. Massoli, T. B. Onasch, P. Davidovits, D. R. Worsnop and D. A. Knopf, The deposition ice nucleation and immersion freezing potential of amorphous secondary organic aerosol: Pathways for ice and mixed-phase cloud formation, J. Geophys. Res.: Atmos., 2012, 117, D16209 Search PubMed.
- T. Meyer, Y. D. Lei and F. Wania, Measuring the release of organic contaminants from melting snow under controlled conditions, Environ. Sci. Technol., 2006, 40(10), 3320–3326 CrossRef CAS PubMed.
- T. Meyer, Y. D. Lei, I. Muradi and F. Wania, Organic contaminant release from melting snow. 2. Influence of snow pack and melt characteristics, Environ. Sci. Technol., 2009, 43(3), 663–668 CrossRef CAS PubMed.
- T. Meyer and F. Wania, Organic contaminant amplification during snowmelt, Water Res., 2008, 42(8–9), 1847–1865 CrossRef CAS PubMed.
-
National Center for Environmental Health (NCEH), Polycyclic Aromatic Hydrocarbons (PAHs), Centers for Disease Control and Prevention (CDC), 2009 Search PubMed.
- A. M. Jones and R. M. Harrison, The effects of meteorological factors on atmospheric bioaerosol concentrations—a review, Sci. Total Environ., 2004, 326(1–3), 151–180 CrossRef CAS PubMed.
- J. Y. Aller, M. R. Kuznetsova, C. J. Jahns and P. F. Kemp, The sea surface microlayer as a source of viral and bacterial enrichment in marine aerosols, J. Aerosol Sci., 2005, 36(5–6), 801–812 CrossRef CAS.
-
C. E. Morris, D. Georgakopoulos and D. Sands, Ice nucleation active bacteria and their potential role in precipitation, in Journal de Physique IV (Proceedings), EDP Sciences, 2004, pp. 87–103 Search PubMed.
- L. A. Currie, J. Kessler, R. A. Fletcher and J. E. Dibb, Long range transport of biomass aerosol to Greenland: Multi-spectroscopic investigation of particles deposited in the snow, J. Radioanal. Nucl. Chem., 2005, 263(2), 399–411 CrossRef CAS.
- H. Puxbaum, A. Caseiro, A. Sánchez-Ochoa, A. Kasper-Giebl, M. Claeys, A. Gelencsér, M. Legrand, S. Preunkert and C. Pio, Levoglucosan levels at background sites in Europe for assessing the impact of biomass combustion on the European aerosol background, J. Geophys. Res.: Atmos., 2007, 112, D23S05 CrossRef.
- R. L. Górny, Microbial aerosols: sources, properties, health effects, exposure assessment—a review, KONA Powder Part. J., 2020, 37, 64–85 CrossRef.
- R. Mortazavi, C. T. Hayes and P. A. Ariya, Ice nucleation activity of bacteria isolated from snow compared with organic and inorganic substrates, Environ. Chem., 2008, 5(6), 373–381 CrossRef CAS.
- R. Mortazavi and P. A. Ariya, The impact of renovation on indoor airborne bacterial and fungal populations, Indoor Built Environ., 2017, 26(10), 1351–1361 CrossRef CAS.
- P. J. DeMott, A. J. Prenni, X. Liu, S. M. Kreidenweis, M. D. Petters, C. H. Twohy, M. Richardson, T. Eidhammer and D. Rogers, Predicting global atmospheric ice nuclei distributions and their impacts on climate, Proc. Natl. Acad. Sci. U. S. A., 2010, 107(25), 11217–11222 CrossRef CAS PubMed.
- B. J. Krueger, V. H. Grassian, J. P. Cowin and A. Laskin, Heterogeneous chemistry of individual mineral dust particles from different dust source regions: the importance of particle mineralogy, Atmos. Environ., 2004, 38(36), 6253–6261 CrossRef CAS.
-
D. Brouwer, G. Lidén, C. Asbach, M. Berges and M. v. Tongeren, Monitoring and Sampling Strategy for (Manufactured) Nano Objects Agglomerates and Aggregates (NOAA); Potential Added Value of the NANODEVICE Project, in Handbook of Nanosafety: Measurement, Exposure and Toxicology, 2014, pp. 254–308 Search PubMed.
- J. Beer, R. Finkel, G. Bonani, H. Gäggeler, U. Görlach, P. Jacob, D. Klockow, C. Langway Jr, A. Neftel and H. Oeschger, Seasonal variations in the concentration of 10Be, Cl−, NO3−, SO42−, H2O2, 210Pb, 3H, mineral dust, and σ18O in greenland snow, Atmos. Environ., Part A, 1991, 25(5–6), 899–904 CrossRef.
- P. De Caritat, G. Hall, S. Gıslason, W. Belsey, M. Braun, N. I. Goloubeva, H. K. Olsen, J. O. Scheie and J. E. Vaive, Chemical composition of arctic snow: concentration levels and regional distribution of major elements, Sci. Total Environ., 2005, 336(1–3), 183–199 CrossRef CAS PubMed.
- V. Kummer and W. R. Thiel, Bioaerosols–sources and control measures, Int. J. Hyg. Environ. Health, 2008, 211(3–4), 299–307 CrossRef PubMed.
- Q. Fu, R. Hou, T. Li, R. Jiang, P. Yan, Z. Ma and Z. Zhou, Effects of soil water and heat relationship under various snow cover during freezing-thawing periods in Songnen Plain, China, Sci. Rep., 2018, 8(1), 1–12 Search PubMed.
- A. Kholodov and K. Golokhvast, Air Pollution of Nature Reserves near Cities in Russia, Scientifica, 2020, 1, 1–8 Search PubMed.
- F. Solademi and S. Thompson, Spatial Analysis of Heavy Metal Emissions in Residential, Commercial and Industrial Areas Adjacent to a Scrap Metal Shredder in Winnipeg, Canada, J. Geosci. Environ. Prot., 2020, 8(5), 359 Search PubMed.
-
Arctic Monitoring, AMAP Assessment 2002: Heavy Metals in the Arctic. Arctic Monitoring and Assessment Programme, 2005, vol. 265 Search PubMed.
- H. Reay, J. France and M. King, Decreased albedo, e-folding depth and photolytic OH radical and NO2 production with increasing black carbon content in Arctic snow, J. Geophys. Res.: Atmos., 2012, 117, D00R20 CrossRef.
- F. Domine, M. Albert, T. Huthwelker, H.-W. Jacobi, A. Kokhanovsky, M. Lehning, G. Picard and W. Simpson, Snow physics as relevant to snow photochemistry, Atmos. Chem. Phys., 2008, 8(2), 208 CrossRef.
- A. M. Grannas, P. B. Shepson and T. R. Filley, Photochemistry and nature of organic matter in Arctic and Antarctic snow, Global Biogeochem. Cycles, 2004, 18, GB1006 CrossRef.
- M. D. King and W. R. Simpson, Extinction of UV radiation in Arctic snow at Alert, Canada (82 N), J. Geophys. Res.: Atmos., 2001, 106(D12), 12499–12507 CrossRef CAS.
- J. France, M. King and J. Lee-Taylor, The importance of considering depth-resolved photochemistry in snow: a radiative-transfer study of NO2 and OH production in Ny-Ålesund (Svalbard) snowpacks, J. Glaciol., 2010, 56(198), 655–663 CrossRef CAS.
- F. Fisher, M. King and J. Lee-Taylor, Extinction of UV-visible radiation in wet midlatitude (maritime) snow: Implications for increased NOx emission, J. Geophys. Res.: Atmos., 2005, 110, D21301 CrossRef.
- A. J. Poulain, E. Garcia, M. Amyot, P. G. Campbell and P. A. Ariya, Mercury distribution, partitioning and speciation in coastal vs. inland High Arctic snow, Geochim. Cosmochim. Acta, 2007, 71(14), 3419–3431 CrossRef CAS.
- C. Pedersen, J. C. Gallet, J. Ström, S. Gerland, S. Hudson, S. Forsström, E. Isaksson and T. Berntsen, In situ observations of black carbon in snow and the corresponding spectral surface albedo reduction, J. Geophys. Res.: Atmos., 2015, 120(4), 1476–1489 CrossRef CAS.
- S. Kang, Y. Zhang, Y. Qian and H. Wang, A review of black carbon in snow and ice and its impact on the cryosphere, Earth-Sci. Rev., 2020, 103346 CrossRef CAS.
- C. He, M. G. Flanner, F. Chen, M. Barlage, K.-N. Liou, S. Kang, J. Ming and Y. Qian, Black carbon-induced snow albedo reduction over the Tibetan Plateau: uncertainties from snow grain shape and aerosol–snow mixing state based on an updated SNICAR model, Atmos. Chem. Phys., 2018, 18(15), 11507–11527 CrossRef CAS.
- G. Li, Z. Wang and N. Huang, A snow distribution model based on snowfall and snow drifting simulations in mountain area, J. Geophys. Res.: Atmos., 2018, 123(14), 7193–7203 Search PubMed.
- O. Hadley, C. Corrigan, T. Kirchstetter, S. Cliff and V. Ramanathan, Measured black carbon deposition on the Sierra Nevada snow pack and implication for snow pack retreat, Atmos. Chem. Phys., 2010, 10(15), 7505–7513 CrossRef CAS.
- O. L. Hadley and T. W. Kirchstetter, Black-carbon reduction of snow albedo, Nat. Clim. Change, 2012, 2(6), 437–440 CrossRef CAS.
- S. G. Warren, Impurities in snow: Effects on albedo and snowmelt, Ann. Glaciol., 1984, 5, 177–179 CrossRef.
- S. G. Warren and W. J. Wiscombe, A model for the spectral albedo of snow. II: Snow containing atmospheric aerosols, J. Atmos. Sci., 1980, 37(12), 2734–2745 CrossRef.
- A. Bartlett, Q. Rochfort, L. Brown and J. Marsalek, Causes of toxicity to Hyalella azteca in a stormwater management facility receiving highway runoff and snowmelt. Part I: Polycyclic aromatic hydrocarbons and metals, Sci. Total Environ., 2012, 414, 227–237 CrossRef CAS PubMed.
- S. J. Wallace, S. R. De Solla, J. A. Head, P. V. Hodson, J. L. Parrott, P. J. Thomas, A. Berthiaume and V. S. Langlois, Polycyclic aromatic compounds (PACs) in the Canadian environment: Exposure and effects on wildlife, Environ. Pollut., 2020, 265, 114863 CrossRef CAS PubMed.
- A. Alexander, P. Chambers and D. Jeffries, Episodic acidification of 5 rivers in Canada's oil sands during snowmelt: A 25-year record, Sci. Total Environ., 2017, 599, 739–749 CrossRef PubMed.
-
S. A. Patterson, The Toxic Effects of Oil Sands Contaminants on Developing Amphibians, Queen's University (Canada), 2019 Search PubMed.
- X. Wang, W. Pu, Y. Ren, X. Zhang, X. Zhang, J. Shi, H. Jin, M. Dai and Q. Chen, Observations and model simulations of snow albedo reduction in seasonal snow due to insoluble light-absorbing particles during 2014 Chinese survey, Atmos. Chem. Phys., 2017, 17, 2279–2296 CrossRef CAS.
- R. Schmidt, Properties of blowing snow, Rev. Geophys., 1982, 20(1), 39–44 CrossRef.
- K. Kuoppamäki, H. Setälä, A.-L. Rantalainen and D. J. Kotze, Urban snow indicates pollution originating from road traffic, Environ. Pollut., 2014, 195, 56–63 CrossRef PubMed.
- B. Croft, U. Lohmann, R. V. Martin, P. Stier, S. Wurzler, J. Feichter, R. Posselt and S. Ferrachat, Aerosol size-dependent below-cloud scavenging by rain and snow in the ECHAM5-HAM, Atmos. Chem. Phys., 2009, 9(14), 4653–4675 CrossRef CAS.
- S. M. Fan, L. W. Horowitz, H. Levy and W. J. Moxim, Impact of air pollution on wet deposition of mineral dust aerosols, Geophys. Res. Lett., 2004, 31, L02104 CrossRef.
- Y. Wu, J. Liu, J. Zhai, L. Cong, Y. Wang, W. Ma, Z. Zhang and C. Li, Comparison of dry and wet deposition of particulate matter in near-surface waters during summer, PLoS One, 2018, 13(6), e0199241 CrossRef PubMed.
- P. Luo, H.-G. Ni, L.-J. Bao, S.-M. Li and E. Y. Zeng, Size distribution of airborne particle-bound polybrominated diphenyl ethers and its implications for dry and wet deposition, Environ. Sci. Technol., 2014, 48(23), 13793–13799 CrossRef CAS PubMed.
- J. Ching, M. West and N. Riemer, Quantifying impacts of aerosol mixing state on nucleation-scavenging of black carbon aerosol particles, Atmosphere, 2018, 9(1), 17 CrossRef.
- S. Ding, D. Zhao, C. He, M. Huang, H. He, P. Tian, Q. Liu, K. Bi, C. Yu and J. Pitt, Observed interactions between black carbon and hydrometeor during wet scavenging in mixed-phase clouds, Geophys. Res. Lett., 2019, 46(14), 8453–8463 CrossRef CAS.
- Y. D. Lei and F. Wania, Is rain or snow a more efficient scavenger of organic chemicals?, Atmos. Environ., 2004, 38(22), 3557–3571 CrossRef CAS.
- M. Z. Jacobson, Climate response of fossil fuel and biofuel soot, accounting for soot’s feedback to snow and sea ice albedo and emissivity, J. Geophys. Res.: Atmos., 2004, 109, D21201 CrossRef.
- L. Feng, Y. An, J. Xu, X. Li, B. Jiang and Y. Liao, Biochemical evolution of dissolved organic matter during snow metamorphism across the ablation season for a glacier on the central tibetan plateau, Sci. Rep., 2020, 10(1), 1–10 CrossRef PubMed.
- C. Zhao, Z. Hu, Y. Qian, L. Ruby Leung, J. Huang, M. Huang, J. Jin, M. Flanner, R. Zhang and H. Wang, Simulating black carbon and dust and their radiative forcing in seasonal snow: a case study over North China with field campaign measurements, Atmos. Chem. Phys., 2014, 14(20), 11475–11491 CrossRef.
- P. A. Ariya, J. F. Hopper and G. W. Harris, C2–C7 hydrocarbon concentrations in Arctic snowpack interstitial air: Potential presence of active Br within the snowpack, J. Atmos. Chem., 1999, 34(1), 55–64 CrossRef CAS.
- C. R. Thompson, P. B. Shepson, J. Liao, L. G. Huey, C. Cantrell, F. Flocke and J. Orlando, Bromine atom production and chain propagation during springtime Arctic ozone depletion events in Barrow, Alaska, Atmos. Chem. Phys., 2017, 17(5), 3401 CrossRef CAS.
- W. R. Simpson, P. K. Peterson, U. Frieß, H. Sihler, J. Lampel, U. Platt, C. Moore, K. Pratt, P. Shepson and J. Halfacre, Horizontal and vertical structure of reactive bromine events probed by bromine monoxide MAX-DOAS, Atmos. Chem. Phys., 2017, 17(15), 9291 CrossRef CAS.
- M. A. Cunningham, E. Snyder, D. Yonkin, M. Ross and T. Elsen, Accumulation of deicing salts in soils in an urban environment, Urban Ecosyst., 2008, 11(1), 17–31 CrossRef.
-
Montreal Municipal Web Site, https://ville.montreal.qc.ca/snowremoval/operations-delais, accessed 4 May 2020 Search PubMed.
-
W. C. Hinds, Aerosol Technology: Properties, Behavior, and Measurement of airborne Particles, John Wiley & Sons, Inc., 2nd edn, 1999, vol. 1 Search PubMed.
- J. P. Shi and R. M. Harrison, Investigation of ultrafine particle formation during diesel exhaust dilution, Environ. Sci. Technol., 1999, 33(21), 3730–3736 CrossRef CAS.
- M. Błaś, K. Cichała-Kamrowska, M. Sobik, Ż. Polkowska and J. Namieśnik, Conditions controlling atmospheric pollutant deposition via snowpack, Environ. Rev., 2010, 18, 87–114 CrossRef.
- C. Sholl and N. Fletcher, Decoration
criteria for surface steps, Acta Metall., 1970, 18(10), 1083–1086 CrossRef.
- G. Vali, Quantitative evaluation of experimental results an the heterogeneous freezing nucleation of supercooled liquids, J. Atmos. Sci., 1971, 28(3), 402–409 CrossRef.
-
J. H. Seinfeld and S. N. Pandis, Atmospheric chemistry and physics: from air pollution to climate change, John Wiley & Sons, 2016 Search PubMed.
- C. Marcolli, S. Gedamke, T. Peter and B. Zobrist, Efficiency of immersion mode ice nucleation on surrogates of mineral dust, Atmos. Chem. Phys., 2007, 7(19), 5081–5091 CrossRef CAS.
- B. Zobrist, T. Koop, B. Luo, C. Marcolli and T. Peter, Heterogeneous ice nucleation rate coefficient of water droplets coated by a nonadecanol monolayer, J. Phys. Chem. C, 2007, 111(5), 2149–2155 CrossRef CAS.
- M. Ganguly, S. Dib, U. Kurien, R. Rangel-Alvarado, Y. Miyahara and P. Ariya, Influence of Environmentally Relevant Physicochemical Conditions on a Highly Efficient Inorganic Ice Nucleating Particle, J. Phys. Chem. C, 2018, 122(32), 18690–18704 CrossRef CAS.
- M. Ganguly, S. Dib and P. A. Ariya, Purely Inorganic Highly Efficient Ice Nucleating Particle, ACS Omega, 2018, 3(3), 3384–3395 CrossRef CAS PubMed.
- T. Koop, B. Luo, A. Tsias and T. Peter, Water activity as the determinant for homogeneous ice nucleation in aqueous solutions, Nature, 2000, 406(6796), 611–614 CrossRef CAS PubMed.
- M. Ganguly and P. A. Ariya, Ice Nucleation of Model Nanoplastics and Microplastics: A Novel Synthetic Protocol and the Influence of Particle Capping at Diverse Atmospheric Environments, ACS Earth Space Chem., 2019, 3(9), 1729–1739 CrossRef CAS.
-
H. R. Pruppacher, J. D. Klett and P. K. Wang, Microphysics of clouds and precipitation, Taylor & Francis, 1998 Search PubMed.
- D. A. Knopf and P. A. Alpert, A water activity based model of heterogeneous ice nucleation kinetics for freezing of water and aqueous solution droplets, Faraday Discuss., 2013, 165, 513–534 RSC.
-
V. Miteva, Bacteria in snow and glacier ice, in Psychrophiles: from biodiversity to biotechnology, Springer, 2008, pp. 31–50 Search PubMed.
- P. A. Ariya, J. Sun, N. A. Eltouny, E. D. Hudson, C. T. Hayes and G. Kos, Physical and chemical characterization of bioaerosols–Implications for nucleation processes, Int. Rev. Phys. Chem., 2009, 28(1), 1–32 Search PubMed.
- S. J. Roeters, T. W. Golbek, M. Bregnhoj, T. Drace, S. Alamdari, W. Roseboom, G. Kramer, T. Santl-Temkiv, K. Finster and S. Woutersen, The Ice Nucleating Protein InaZ is Activated by Low Temperature, bioRxiv, 2020, 1–22 Search PubMed.
- N. Link, N. Removski, J. Yun, L. T. Fleming, S. A. Nizkorodov, A. K. Bertram and H. A. Al-Abadleh, Dust-Catalyzed Oxidative Polymerization of Catechol and its Impacts on Ice Nucleation Efficiency and Optical Properties, ACS Earth Space Chem., 2020, 4(7), 1127–1139 Search PubMed.
- M. Joghataei, F. Ostovari, S. Atabakhsh and N. Tobeiha, Heterogeneous ice nucleation by Graphene nanoparticles, Sci. Rep., 2020, 10(1), 1–9 Search PubMed.
- S. Sauvé and M. Desrosiers, A review of what is an emerging contaminant, Chem. Cent. J., 2014, 8(1), 15 Search PubMed.
- H. Ghimire and P. A. Ariya, E-Wastes: Bridging the Knowledge Gaps in Global Production Budgets, Composition, Recycling and Sustainability Implications, Sustainable Chem., 2020, 1(2), 154–182 Search PubMed.
- C. E. Willis, J. L. Kirk, V. L. St. Louis, I. Lehnherr, P. A. Ariya and R. B. Rangel-Alvarado, Sources of methylmercury to snowpacks of the Alberta Oil Sands Region: A study of in situ methylation and particulates, Environ. Sci. Technol., 2018, 52(2), 531–540 CrossRef CAS PubMed.
- A. Veysseyre, K. Moutard, C. Ferrari, K. Van de Velde, C. Barbante, G. Cozzi, G. Capodaglio and C. Boutron, Heavy metals in fresh snow collected at different altitudes in the Chamonix and Maurienne valleys, French Alps: initial results, Atmos. Environ., 2001, 35(2), 415–425 CrossRef CAS.
- M. Baudrimont, A. Arini, C. Guégan, Z. Venel, J. Gigault, B. Pedrono, J. Prunier, L. Maurice, A. Ter Halle and A. Feurtet-Mazel, Ecotoxicity of polyethylene nanoplastics from the North Atlantic oceanic gyre on freshwater and marine organisms (microalgae and filter-feeding bivalves), Environ. Sci. Pollut. Res., 2020, 27(4), 3746–3755 CrossRef CAS PubMed.
- O. Pikuda, E. G. Xu, D. Berk and N. Tufenkji, Toxicity assessments of micro-and nanoplastics can be confounded by preservatives in commercial formulations, Environ. Sci. Technol. Lett., 2018, 6(1), 21–25 CrossRef.
- C. Ferrario, A. Finizio and S. Villa, Legacy and emerging contaminants in meltwater of three Alpine glaciers, Sci. Total Environ., 2017, 574, 350–357 CrossRef CAS PubMed.
- Z. Xie, Z. Wang, O. Magand, A. Thollot, R. Ebinghaus, W. Mi and A. Dommergue, Occurrence of legacy and emerging organic contaminants in snow at Dome C in the Antarctic, Sci. Total Environ., 2020, 140200 CrossRef CAS PubMed.
- M. Bergmann, V. Wirzberger, T. Krumpen, C. Lorenz, S. Primpke, M. B. Tekman and G. Gerdts, High quantities of microplastic in Arctic deep-sea sediments from the Hausgarten observatory, Environ. Sci. Technol., 2017, 51(19), 11000–11010 CrossRef CAS PubMed.
- S. Zhao, M. Danley, J. E. Ward, D. Li and T. J. Mincer, An approach for extraction, characterization and quantitation of microplastic in natural marine snow using Raman microscopy, Anal. Methods, 2017, 9(9), 1470–1478 RSC.
- C. Peng, X. Tang, X. Gong, Y. Dai, H. Sun and L. Wang, Development and Application of a Mass Spectrometry Method for Quantifying Nylon Microplastics in Environment, Anal. Chem., 2020, 92(20), 13930–13935 CrossRef CAS PubMed.
- A.-K. Kniggendorf, C. Wetzel and B. Roth, Microplastics detection in streaming tap water with Raman spectroscopy, Sensors, 2019, 19(8), 1839 CrossRef CAS PubMed.
-
N. A. Abreo, E. Macusi and R. Aurelio, Floating marine macroplastics and its implications on marine wildlife, 2019 Search PubMed.
- C. Campanale, C. Massarelli, I. Savino, V. Locaputo and V. F. Uricchio, A detailed review study on potential effects of microplastics and additives of concern on human health, Int. J. Environ. Res. Public Health, 2020, 17(4), 1212 CrossRef CAS PubMed.
- L. G. A. Barboza, A. D. Vethaak, B. R. Lavorante, A.-K. Lundebye and L. Guilhermino, Marine microplastic debris: An emerging issue for food security, food safety and human health, Mar. Pollut. Bull., 2018, 133, 336–348 CrossRef CAS PubMed.
-
M. Smith, D. C. Love, C. M. Rochman and R. A. Neff, Microplastics in seafood and the implications for human health, Current Environmental Health Reports, 2018, vol. 5 (3) pp. 375–386 Search PubMed.
- G. Limonta, A. Mancia, A. Benkhalqui, C. Bertolucci, L. Abelli, M. C. Fossi and C. Panti, Microplastics induce transcriptional changes, immune response and behavioral alterations in adult zebrafish, Sci. Rep., 2019, 9(1), 1–11 CrossRef CAS PubMed.
- H. K. Imhof, J. Rusek, M. Thiel, J. Wolinska and C. Laforsch, Do microplastic particles affect Daphnia magna at the morphological, life history and molecular level?, PloS One, 2017, 12(11), e0187590 CrossRef PubMed.
- A. P. Fornea, S. D. Brooks, J. B. Dooley and A. Saha, Heterogeneous freezing of ice on atmospheric aerosols
containing ash, soot, and soil, J. Geophys. Res.: Atmos., 2009, 114, D13201 CrossRef.
- G. Kulkarni, S. China, S. Liu, M. Nandasiri, N. Sharma, J. Wilson, A. C. Aiken, D. Chand, A. Laskin and C. Mazzoleni, Ice nucleation activity of diesel soot particles at cirrus relevant temperature conditions: Effects of hydration, secondary organics coating, soot morphology, and coagulation, Geophys. Res. Lett., 2016, 43(7), 3580–3588 CrossRef.
- R. Ullrich, C. Hoose, O. Möhler, M. Niemand, R. Wagner, K. Höhler, N. Hiranuma, H. Saathoff and T. Leisner, A new ice nucleation active site parameterization for desert dust and soot, J. Atmos. Sci., 2017, 74(3), 699–717 CrossRef.
- D. Liu, C. He, J. P. Schwarz and X. Wang, Lifecycle of light-absorbing carbonaceous aerosols in the atmosphere, npj Clim. Atmos. Sci., 2020, 3(1), 1–18 CrossRef.
- J. Dargay, D. Gately and M. Sommer, Vehicle ownership and income growth, worldwide: 1960-2030, Energy J., 2007, 28(4), 143–170 Search PubMed.
- S. E. Findlay and V. R. Kelly, Emerging indirect and long-term road salt effects on ecosystems, Ann. N. Y. Acad. Sci., 2011, 1223(1), 58–68 CrossRef CAS PubMed.
- D. M. Ramakrishna and T. Viraraghavan, Environmental impact of chemical deicers–a review, Water, Air, Soil Pollut., 2005, 166(1), 49–63 CrossRef CAS.
- J. Henneberger, J. P. Fugal, O. Stetzer and U. Lohmann, HOLIMO II: a digital holographic instrument for ground-based in situ observations of microphysical properties of mixed-phase clouds, Atmos. Meas. Tech., 2013, 6(11), 2975–2987 CrossRef.
- N. L. Walcutt, B. Knörlein, I. Cetinić, Z. Ljubesic, S. Bosak, T. Sgouros, A. L. Montalbano, A. Neeley, S. Menden-Deuer and M. M. Omand, Assessment of holographic microscopy for quantifying marine particle size and concentration, Limnol. Oceanogr.: Methods, 2020, 18(9), 516–530 CrossRef PubMed.
- M. L. Diamond, S. E. Gingrich, K. Fertuck, B. E. McCarry, G. A. Stern, B. Billeck, B. Grift, D. Brooker and T. D. Yager, Evidence for organic film on an impervious urban surface: characterization and potential teratogenic effects, Environ. Sci. Technol., 2000, 34(14), 2900–2908 CrossRef CAS.
-
D. J. Donaldson, J. T. Clouthier, K. J. Morenz and A. Marr, Chemical Morphology and Reactivity at Environmental Interfaces, in Multiphase Environmental Chemistry in the Atmosphere, ACS Publications, 2018, pp. 193–207 Search PubMed.
- H. Forrister, J. Liu, E. Scheuer, J. Dibb, L. Ziemba, K. L. Thornhill, B. Anderson, G. Diskin, A. E. Perring, J. P. Schwarz, P. Campuzano-Jost, D. A. Day, B. B. Palm, J. L. Jimenez, A. Nenes and R. J. Weber, Evolution of brown carbon in wildfire plumes, Geophys. Res. Lett., 2015, 42(11), 4623–4630 CrossRef CAS.
- R. F. Hems, E. G. Schnitzler, C. Liu-Kang, C. D. Cappa and J. P. D. Abbatt, Aging of Atmospheric Brown Carbon Aerosol, ACS Earth Space Chem., 2021, 5(4), 722–748 CrossRef CAS.
- A. Laskin, J. Laskin and S. A. Nizkorodov, Chemistry of atmospheric brown carbon, Chem. Rev., 2015, 115(10), 4335–4382 CrossRef CAS PubMed.
- J. Yan, X. Wang, P. Gong, C. Wang and Z. Cong, Review of brown carbon aerosols: Recent progress and perspectives, Sci. Total Environ., 2018, 634, 1475–1485 CrossRef CAS PubMed.
- W. Hu, D. Liu, S. Su, L. Ren, H. Ren, L. Wei, S. Yue, Q. Xie, Z. Zhang, Z. Wang, N. Yang, L. Wu, J. Deng, Y. Qi and P. Fu, Photochemical Degradation of Organic Matter in the Atmosphere, Adv. Sustainable Syst., 2021, 5(11), 2100027 CrossRef CAS.
- P. Lin, P. K. Aiona, Y. Li, M. Shiraiwa, J. Laskin, S. A. Nizkorodov and A. Laskin, Molecular Characterization of Brown Carbon in Biomass Burning Aerosol Particles, Environ. Sci. Technol., 2016, 50(21), 11815–11824 CrossRef CAS PubMed.
- P. Tuccella, G. Pitari, V. Colaiuda, E. Raparelli and G. Curci, Present-day radiative effect from radiation-absorbing aerosols in snow, Atmos. Chem. Phys., 2021, 21(9), 6875–6893 CrossRef CAS.
- S. J. Doherty, S. G. Warren, T. C. Grenfell, A. D. Clarke and R. E. Brandt, Light-absorbing impurities in Arctic snow, Atmos. Chem. Phys., 2010, 10(23), 11647–11680 CrossRef CAS.
- S. Yue, S. Bikkina, M. Gao, L. A. Barrie, K. Kawamura and P. Fu, Sources and Radiative Absorption of Water-Soluble Brown Carbon in the High Arctic Atmosphere, Geophys. Res. Lett., 2019, 46(24), 14881–14891 CrossRef.
- T. E. Barrett and R. J. Sheesley, Year-round optical properties and source characterization of Arctic organic carbon aerosols on the North Slope Alaska, J. Geophys. Res.: Atmos., 2017, 122(17), 9319–9331 CrossRef CAS.
- C. Gul, S. P. Puppala, S. Kang, B. Adhikary, Y. Zhang, S. Ali, Y. Li and X. Li, Concentrations and source regions of light-absorbing particles in snow/ice in northern Pakistan and their impact on snow albedo, Atmos. Chem. Phys., 2018, 18(7), 4981–5000 CrossRef CAS.
- J. Cozic, S. Mertes, B. Verheggen, D. J. Cziczo, S. J. Gallavardin, S. Walter, U. Baltensperger and E. Weingartner, Black carbon enrichment in atmospheric ice particle residuals observed in lower tropospheric mixed phase clouds, J. Geophys. Res.: Atmos., 2008, 113, D15209 CrossRef.
- M. Kamphus, M. Ettner-Mahl, T. Klimach, F. Drewnick, L. Keller, D. J. Cziczo, S. Mertes, S. Borrmann and J. Curtius, Chemical composition of ambient aerosol, ice residues and cloud droplet residues in mixed-phase clouds: single particle analysis during the Cloud and Aerosol Characterization Experiment (CLACE 6), Atmos. Chem. Phys., 2010, 10(16), 8077–8095 CrossRef CAS.
- P. Kupiszewski, M. Zanatta, S. Mertes, P. Vochezer, G. Lloyd, J. Schneider, L. Schenk, M. Schnaiter, U. Baltensperger, E. Weingartner and M. Gysel, Ice residual properties in mixed-phase clouds at the high-alpine Jungfraujoch site, J. Geophys. Res.: Atmos., 2016, 121(20), 12343–12362 Search PubMed.
- T. C. Bond, S. J. Doherty, D. W. Fahey, P. M. Forster, T. Berntsen, B. J. DeAngelo, M. G. Flanner, S. Ghan, B. Kärcher, D. Koch, S. Kinne, Y. Kondo, P. K. Quinn, M. C. Sarofim, M. G. Schultz, M. Schulz, C. Venkataraman, H. Zhang, S. Zhang, N. Bellouin, S. K. Guttikunda, P. K. Hopke, M. Z. Jacobson, J. W. Kaiser, Z. Klimont, U. Lohmann, J. P. Schwarz, D. Shindell, T. Storelvmo, S. G. Warren and C. S. Zender, Bounding the role of black carbon in the climate system: A scientific assessment, J. Geophys. Res.: Atmos., 2013, 118(11), 5380–5552 CrossRef CAS.
- D. A. Lack, J. M. Langridge, R. Bahreini, C. D. Cappa, A. M. Middlebrook and J. P. Schwarz, Brown carbon and internal mixing in biomass burning particles, Proc. Natl. Acad. Sci. U. S. A., 2012, 109(37), 14802–14807 CrossRef CAS PubMed.
- G.-M. Wu, Z.-Y. Cong, S.-C. Kang, K. Kawamura, P.-Q. Fu, Y.-L. Zhang, X. Wan, S.-P. Gao and B. Liu, Brown carbon in the cryosphere: Current knowledge and perspective, Adv. Clim. Chang. Res., 2016, 7(1–2), 82–89 CrossRef.
- C. Zhang, Y. Zhang, M. J. Wolf, L. Nichman, C. Shen, T. B. Onasch, L. Chen and D. J. Cziczo, The effects of morphology, mobility size, and secondary organic aerosol (SOA) material coating on the ice nucleation activity of black carbon in the cirrus regime, Atmos. Chem. Phys., 2020, 20(22), 13957–13984 CrossRef CAS.
- D. Liu, J. W. Taylor, D. E. Young, M. J. Flynn, H. Coe and J. D. Allan, The effect of complex black carbon microphysics on the determination of the optical properties of brown carbon, Geophys. Res. Lett., 2015, 42(2), 613–619 CrossRef CAS.
- S. M. Skiles, M. Flanner, J. M. Cook, M. Dumont and T. H. Painter, Radiative forcing by light-absorbing particles in snow, Nat. Clim. Change, 2018, 8(11), 964–971 CrossRef.
- A. Zhang, Y. Wang, Y. Zhang, R. J. Weber, Y. Song, Z. Ke and Y. Zou, Modeling the global radiative effect of brown carbon: a potentially larger heating source in the tropical free troposphere than black carbon, Atmos. Chem. Phys., 2020, 20(4), 1901–1920 CrossRef CAS.
- J. Zhu and J. E. Penner, Indirect Effects of Secondary Organic Aerosol on Cirrus Clouds, J. Geophys. Res.: Atmos., 2020, 125(7), e2019JD032233 CAS.
- C. Hoose and O. Möhler, Heterogeneous ice nucleation on atmospheric aerosols: a review of results from laboratory experiments, Atmos. Chem. Phys., 2012, 12(20), 9817–9854 CrossRef CAS.
- P. J. DeMott, Y. Chen, S. M. Kreidenweis, D. C. Rogers and D. E. Sherman, Ice formation by black carbon particles, Geophys. Res. Lett., 1999, 26(16), 2429–2432 CrossRef CAS.
- B. J. Murray, D. O'Sullivan, J. D. Atkinson and M. E. Webb, Ice nucleation by particles immersed in supercooled cloud droplets, Chem. Soc. Rev., 2012, 41(19), 6519–6554 RSC.
- B. Friedman, G. Kulkarni, J. Beránek, A. Zelenyuk, J. A. Thornton and D. J. Cziczo, Ice nucleation and droplet formation by bare and coated soot particles, J. Geophys. Res., 2011, 116, D17203 CrossRef.
- R. Ullrich, C. Hoose, O. Mohler, M. Niemand, R. Wagner, K. Hohler, N. Hiranuma, H. Saathoff and T. Leisner, A New Ice Nucleation Active Site Parameterization for Desert Dust and Soot, J. Atmos. Sci., 2017, 74(3), 699–717 CrossRef.
- F. Mahrt, C. Marcolli, R. O. David, P. Gronquist, E. J. B. Meier, U. Lohmann and Z. A. Kanji, Ice nucleation abilities of soot particles determined with the Horizontal Ice Nucleation Chamber, Atmos. Chem. Phys., 2018, 18(18), 13363–13392 CrossRef CAS.
- L. Nichman, M. Wolf, P. Davidovits, T. B. Onasch, Y. Zhang, D. R. Worsnop, J. Bhandari, C. Mazzoleni and D. J. Cziczo, Laboratory study of the heterogeneous ice nucleation on black-carbon-containing aerosol, Atmos. Chem. Phys., 2019, 19(19), 12175–12194 CrossRef CAS.
- F. Mahrt, P. A. Alpert, J. Dou, P. Gronquist, P. C. Arroyo, M. Ammann, U. Lohmann and Z. A. Kanji, Aging induced changes in ice nucleation activity of combustion aerosol as determined by near edge X-ray absorption fine structure (NEXAFS) spectroscopy, Environ. Sci.: Processes Impacts, 2020, 22(4), 895–907 RSC.
- C. Marcolli, Deposition nucleation viewed as homogeneous or immersion freezing in pores and cavities, Atmos. Chem. Phys., 2014, 14(4), 2071–2104 CrossRef.
- C. Chou, Z. A. Kanji, O. Stetzer, T. Tritscher, R. Chirico, M. F. Heringa, E. Weingartner, A. S. H. Prévôt, U. Baltensperger and U. Lohmann, Effect of photochemical ageing on the ice nucleation properties of diesel and wood burning particles, Atmos. Chem. Phys., 2013, 13(2), 761–772 CrossRef.
- R. Ikhenazene, C. Pirim, J. A. Noble, C. Irimiea, Y. Carpentier, I. K. Ortega, F.-X. Ouf, C. Focsa and B. Chazallon, Ice Nucleation Activities of Carbon-Bearing Materials in Deposition Mode: From Graphite to Airplane Soot Surrogates, J. Phys. Chem. C, 2019, 124(1), 489–503 CrossRef.
- G. P. Schill, P. J. DeMott, E. W. Emerson, A. M. C. Rauker, J. K. Kodros, K. J. Suski, T. C. J. Hill, E. J. T. Levin, J. R. Pierce, D. K. Farmer and S. M. Kreidenweis, The contribution of black carbon to global ice nucleating particle concentrations relevant to mixed-phase clouds, Proc. Natl. Acad. Sci. U. S. A., 2020, 117(37), 22705–22711 CrossRef CAS PubMed.
- O. Möhler, S. Büttner, C. Linke, M. Schnaiter, H. Saathoff, O. Stetzer, R. Wagner, M. Krämer, A. Mangold, V. Ebert and U. Schurath, Effect of sulfuric acid coating on heterogeneous ice nucleation by soot aerosol particles, J. Geophys. Res., 2005, 110, D11210 CrossRef.
- I. Crawford, O. Möhler, M. Schnaiter, H. Saathoff, D. Liu, G. McMeeking, C. Linke, M. Flynn, K. N. Bower, P. J. Connolly, M. W. Gallagher and H. Coe, Studies of propane flame soot acting as heterogeneous ice nuclei in conjunction with single particle soot photometer measurements, Atmos. Chem. Phys., 2011, 11(18), 9549–9561 CrossRef CAS.
- L. Lupi and V. Molinero, Does hydrophilicity of carbon particles improve their ice nucleation ability?, J. Phys. Chem. A, 2014, 118(35), 7330–7337 CrossRef CAS PubMed.
- H. Xue, Y. Lu, H. Geng, B. Dong, S. Wu, Q. Fan, Z. Zhang, X. Li, X. Zhou and J. Wang, Hydroxyl Groups on the Graphene Surfaces Facilitate Ice Nucleation, J. Phys. Chem. Lett., 2019, 10(10), 2458–2462 CrossRef CAS PubMed.
- J. Chen, Z. J. Wu, X. Zhao, Y. J. Wang, J. C. Chen, Y. T. Qiu, T. M. Zong, H. X. Chen, B. B. Wang, P. Lin, W. Liu, S. Guo, M. S. Yao, L. M. Zeng, H. Wex, X. Liu, M. Hu and S. M. Li, Atmospheric Humic-Like Substances (HULIS) Act as Ice Active Entities, Geophys. Res. Lett., 2021, 48(14), e2021GL092443 CAS.
-
F. Yan, S. Kang, C. Li, Y. Zhang, X. Qin, Y. Li, X. Zhang, Z. Hu, P. Chen, X. Li, B. Qu and M. Sillanpää, Concentration, sources and light absorption characteristics of dissolved organic carbon on a medium-sized valley glacier, northern in, The Cryosphere, ed. T. Plateau, 2016, vol. 10 (6), pp. 2611–2621 Search PubMed.
- N. D. Beres, D. Sengupta, V. Samburova, A. Y. Khlystov and H. Moosmüller, Deposition of brown carbon onto snow: changes in snow optical and radiative properties, Atmos. Chem. Phys., 2020, 20(10), 6095–6114 CrossRef CAS.
- Y. Zhang, S. Kang, T. Gao, J. Schmale, Y. Liu, W. Zhang, J. Guo, W. Du, Z. Hu, X. Cui and M. Sillanpaa, Dissolved organic carbon in snow cover of the Chinese Altai Mountains, Central Asia: Concentrations, sources and light-absorption properties, Sci. Total Environ., 2019, 647, 1385–1397 CrossRef CAS PubMed.
- K. A. Koehler, P. J. DeMott, S. M. Kreidenweis, O. B. Popovicheva, M. D. Petters, C. M. Carrico, E. D. Kireeva, T. D. Khokhlova and N. K. Shonija, Cloud condensation nuclei and ice nucleation activity of hydrophobic and hydrophilic soot particles, Phys. Chem. Chem. Phys., 2009, 11(36), 7906–7920 RSC.
- G. Kulkarni, S. China, S. Liu, M. Nandasiri, N. Sharma, J. Wilson, A. C. Aiken, D. Chand, A. Laskin, C. Mazzoleni, M. Pekour, J. Shilling, V. Shutthanandan, A. Zelenyuk and R. A. Zaveri, Ice nucleation activity of diesel soot particles at cirrus relevant temperature conditions: Effects of hydration, secondary organics coating, soot morphology, and coagulation, Geophys. Res. Lett., 2016, 43(7), 3580–3588 CrossRef.
-
https://www.canadiangeographic.ca/article/defeating-winter
.
-
https://globalnews.ca/news/7745456/calgary-roads-snow-clearing-budget-2021/
.
-
https://yle.fi/news/3-12330962
.
-
https://www.thelocal.no/20180214/oslo-sets-aside-53-million-kroner-to-clear-away-snow/
.
-
https://sverigesradio.se/artikel/5749141
.
-
https://www.eit.edu.au/snow-removal-an-engineering-challenge-in-japan/
.
-
https://www.theguardian.com/world/2014/mar/04/cost-states-millions-winter-storm-ice
.
-
https://montreal.weatherstats.ca/charts/snow-yearly.html
.
-
https://calgary.weatherstats.ca/charts/snow-yearly.html
.
-
https://www.weather-atlas.com/en/finland/helsinki-climate
.
-
https://weatherspark.com/y/68697/Average-Weather-in-Oslo-Norway-Year-Round
.
-
https://www.worldweatheronline.com/stockholm-weather-averages/stockholms-lan/se.aspx
.
-
https://www.statista.com/statistics/1168003/japan-annual-snowfall-sapporo/
.
-
https://stateclimatologist.web.illinois.edu/snowfall-trends/
.
-
https://www.currentresults.com/Weather/New-York/Places/new-york-city-snowfall-totals-snow-accumulation-averages.php
.
-
https://www.calgary.ca/transportation/roads/road-maintenance/snow-and-ice-control/salt-management-plan-faqs.html
.
-
https://yle.fi/news/3-5900311
.
-
https://www.lifeinnorway.net/how-to-use-less-salt-on-snowy-roads/
.
-
https://www.transportation.ohio.gov/programs/snow-and-ice/snow-ice-practices
.
-
https://www.chicagotribune.com/news/environment/ct-chicago-waterways-lake-michigan-road-salt-20220225-alsooi5f6bg4fcxnshqkqftuw4-story.html
.
-
https://www.nj.com/news/2019/01/will-murphys-plan-to-constantly-salt-the-roads-wreak-environmental-havoc-time-will-tell.html
.
-
https://news.climate.columbia.edu/2018/12/13/cutting-salt-winter-road-maintenance/
.
-
https://stormwater.pca.state.mn.us/index.php?title=Lowest_practical_melting_temperature
.
-
E. Thunqvist, Long-Term Effects of Deicing Salt on the Roadside Environment, Part II, Groundwater and Surface Water, Transportation Research Board Conference Proceedings, 2001, vol. 23 Search PubMed.
-
M. Akin, J. Huang, X. Shi, D. Veneziano and D. Williams, Snow Removal at Extreme Temperature: Final Report, Western Transportation Institute, 2013 Search PubMed.
-
G. Waidley, B. Hirt and R. Wright, Assessing the Effectiveness of Potassium Acetate to Control Snow and Ice on Minnesota Highways: Final Report, Minnesota Department of Transportation, 2020 Search PubMed.
- S. R. Corsi, L. A. De Cicco, M. A. Lutz and R. M. Hirsch, River chloride trends in snow-affected urban watersheds: increasing concentrations outpace urban growth rate and are common among all seasons, Sci. Total Environ., 2015, 508, 488–497 CrossRef CAS PubMed.
- I. Czerniawska-Kusza, G. Kusza and M. Duzynski, Effect of deicing salts on urban soils and health status of roadside trees in the opole region, Environ. Toxicol., 2004, 19(4), 296–301 CrossRef CAS PubMed.
- L. Fay and X. Shi, Environmental Impacts of Chemicals for Snow and Ice Control: State of the Knowledge, Water, Air, Soil Pollut., 2012, 223(5), 2751–2770 CrossRef CAS.
- W. D. Hintz, L. Fay and R. A. Relyea, Road salts, human safety, and the rising salinity of our fresh waters, Front. Ecol. Environ., 2021, 20(1), 22–30 CrossRef.
- A. Jamshidi, A. R. Goodarzi and P. Razmara, Long-term impacts of road salt application on the groundwater contamination in urban environments, Environ. Sci. Pollut. Res., 2020, 27(24), 30162–30177 CrossRef CAS PubMed.
- Z. Li, Y. Liang, J. Zhou and X. Sun, Impacts of de-icing salt pollution on urban road greenspace: a case study of Beijing, Front. Environ. Sci. Eng., 2014, 8(5), 747–756 CrossRef CAS.
- U. Mahrosh, M. Kleiven, S. Meland, B. O. Rosseland, B. Salbu and H. C. Teien, Toxicity of road deicing salt (NaCl) and copper (Cu) to fertilization and early developmental stages of Atlantic salmon (Salmo salar), J. Hazard. Mater., 2014, 280, 331–339 CrossRef CAS PubMed.
- D. F. Vitaliano, An Economic Assessment of the Social Costs of Highway Salting and the Efficiency of Substituting a New Deicing Material, Journal of Policy Analysis and Management, 1992, 11(3), 397–418 CrossRef.
- M. Borris, H. Österlund, J. Marsalek and M. Viklander, Snow pollution management in urban areas: an idea whose time has come?, Urban Water J., 2021, 18(10), 840–849 CrossRef.
|
This journal is © The Royal Society of Chemistry 2022 |